Origins of heart diseases
The heart is the first organ to form during embryogenesis.Reference Marín-García 1 Its development is a highly regulated process relying on the intervention of various factors that orchestrate cardiac morphogenesis, myogenesis, contractility and metabolism. Mammalian heart undergoes considerable maturation in utero, such that the majority of cardiomyocytes, present shortly after birth, beat for a lifetime.Reference Thornburg, Jonker and O’Tierney 2 , Reference Van Berlo and Molkentin 3 Thus, an early challenge altering cardiomyocytes maturation, endowment and growth in utero can give rise to coronary artery disease,Reference Bubb, Cock and Black 4 cardiomyopathy,Reference Nakano, Minami and Braas 5 myocarditis,Reference Buyon, Swersky, Fox, Bierman and Winchester 6 congenital malformationsReference Cole, Yutzey and Brar 7 and valvular disease,Reference Mills, Troendle, Conley, Carter and Druschel 8 which can impact lifelong cardiac functions and lead to heart failure. By affecting at least 26 million people,Reference Savarese and Lund 9 heart failure is a worldwide leading cause of death. Heart failure corresponds to the incapacity of the heart to maintain an adequate circulation of blood in the bodily tissues. Heart failure can be caused by inherited disorders or develop after birth, and the reason behind this remains, most of the time, unknown. Mutations in a variety of genes have been associated with heart diseases in human.Reference Ghouse, Skov, Bigseth, Ahlberg, Kanters and Olesen 10 , Reference Nolte, Munoz and Tragante 11 In addition, the use of animal models based on gene mutations suggests a multiplicity of pathways affecting cardiomyocytes such as transcriptional control,Reference Lin, Schwarz, Bucana and Olson 12 calcium homeostasis,Reference Tzimas, Johnson and Santiago 13 force generation and transmission,Reference Ruggiero, Chen, Lombardi, Rodriguez and Marian 14 as well as metabolism.Reference Slingo, Cole and Carr 15 The use of fast and cost-effective technologies, genetic testing based on the entire exome or genome sequencingReference Crotti, Johnson and Graf 16 revealed a high number of variants which have little effect on cardiac health conditions. A number of polymorphisms have been suggested as a risk factor for heart diseases, however, most of these variants represent susceptibilities that require additional mutations or injury to cause the phenotype of disease. As such, polymorphisms in the adrenergic pathway,Reference Dorn, Triposkiadis, Karayannis, Giamouzis, Skoularigis, Louridas and Butler 17 G protein-coupled receptor kinases,Reference Liggett, Cresci and Kelly 18 in the renin–angiotensin–aldosterone systemReference Danser, Schalekamp and Bax 19 , Reference Bai, Wang, Hu and Wei 20 have been described.
Besides genetic causes of heart failure, environment,Reference Humblet, Birnbaum, Rimm, Mittleman and Hauser 21 – Reference Pan, Li and Tsai 24 nutrition and lifestyleReference Pharr, Coughenour and Bungum 25 – Reference Iribarren, Tekawa, Sidney and Friedman 27 have a major impact on heart function. Diet, obesity, diabetes and alcohol consumption (in a dose-dependent mannerReference Catena, Colussi and Verheyen 28 – Reference Brunner, Herbel and Drobesch 30 ) are particularly of high concern in heart disease etiology. Given the worldwide epidemic of obesity and metabolic disorders, strong efforts are being made to gain insight into the mechanisms of cardiovascular health influenced by nutrition. The important role of nutrition on cardiac functions is reflected by the model of mice fed through their long-term feeding with high-fat diet which results in cardiac hypertrophy and fibrosisReference Wang, Li, Zhao, Peng and Zuo 31 – Reference Bobbert, Jenke and Bobbert 33 associated with lipotoxicity.Reference Christoffersen, Bollano and Lindegaard 34 Interestingly enough, correlations between myocardial lipid accumulation and cardiac dysfunction have been noted in humans. When comparing healthy lean individuals to individuals with moderate obesity, an increase in triglyceride accumulation precedes ventricular hypertrophy.Reference Kankaanpaa, Lehto and Parkka 35 Experience of stress such as long-term stress (i.e. work related) as well as acute stress (e.g. experienced during an earthquake,Reference Trichopoulos, Katsouyanni, Zavitsanos, Tzonou and Dalla-Vorgia 36 a terrorist attackReference Kark, Goldman and Epstein 37 or an industrial accidentReference Ruidavets, Paterniti, Bongard, Giroux, Cassadou and Ferrieres 38 ) triggers coronary heart disease,Reference Chandola, Britton and Brunner 39 myocardial infarction or stroke. Those factors linked to environment, nutrition and lifestyle have stronger effects on heart when they occur early during development.
Early exposure to environmental challenges induces cardiac disorders
Since the work of Professor D.J. Barker and the hypothesis of the Developmental Origins of Health and Disease (DOHaD) providing a framework to assess the effect of early nutrition and growth on long-term health, a bulk of data identified the developmental period as a phase when the fetus integrate information from the fetal milieu allowing the organism to adapt to change later in life. The fetus learns to adapt to the environment it expects to encounter once outside of the womb. Such ‘programming’ is a normal, adaptive component of development by which the fetus is predisposed to inhabit an environment with expected resources. When fetal conditions do not match the environment later during life, the child or the adult can develop non-adapted physiological response to the environment. As such, a challenge during fetal life can therefore modify the developmental trajectory and change the developmental ‘predictive adaptive’ response to the adverse in utero environment into maladaptive in adulthood if conditions (e.g. diet and lifestyle) change. Given that turnover of human cardiomyocytes is limited over a lifetime, the heart is particularly sensitive to challenges that perturb the intrauterine environment. In addition to acute effects that may drive long-lasting cardiac dysfunction,Reference Auger, Fraser, Sauve, Bilodeau-Bertrand and Kosatsky 40 early developmental exposure to environmental challenges can induce changes that may impact cardiac functions only later in life (Fig. 1). Insults during infancy, gestational and periconceptional periods are associated with an increased risk of heart diseases in offspring. Fetal hypoxia is one of the most common consequences of complicated pregnancies, occurring during preeclampsia, placental insufficiency or infection. In sheep, a large animal model in which the timing of cardiomyocyte maturation is similar to human, prenatal hypoxia can promote fetal growth restriction associated with a reduction in the total number of cardiomyocyte.Reference Brain, Allison and Niu 41 In perturbed intrauterine environments such as fetal hypoxia,Reference Giussani, Camm and Niu 42 placental insufficiency and malnutrition,Reference Forsen, Eriksson, Tuomilehto, Teramo, Osmond and Barker 43 , Reference Roseboom, De Rooij and Painter 44 adaptations made by the fetus cause permanent changes in tissue structure and function, with depressed cardiac performance and cardiomyopathies that persist into adulthood.Reference Tintu, Rouwet and Verlohren 45 , Reference Botting, McMillen, Forbes, Nyengaard and Morrison 46 Those defects have been associated with left ventricular hypertrophy, altered myocardial contractility, endothelial dysfunction,Reference Brain, Allison and Niu 41 susceptibility to ischemia reperfusion injuryReference Rueda-Clausen, Morton, Lopaschuk and Davidge 47 and premature cardiac ageing phenotype.Reference Kuo, Li, Li, Huber, Nathanielsz and Clarke 48 Several underlying mechanisms have been proposed, including an increase in beta(2)-adrenoreceptor and the G(s)alpha/G(i)alpha ratio, and a decrease in heat shock protein 70 and endothelial nitric oxide synthase in the left ventricle,Reference Li, Xiao, Estrella, Ducsay, Gilbert and Zhang 49 or increased expression of insulin-like growth factor 2 (IGF-2) and its receptor IGF-2R in utero growth,Reference Wang, Zhang and McMillen 50 as well as up-regulated IGF-2R-Gαq signaling in low-birthweight lamb.Reference Wang, Tosh and Zhang 51 In the context of exposure to nutritional excess during perinatal life such as maternal obesity and hyperglycemiaReference Lin, Yang, Reece and Yang 52 as well as child obesity, impaired cardiac function,Reference Martins, Vieira, de Souza and Moura 53 are induced later in life. These exposures change the programming of cardiac metabolism, inducing excessive lipid accumulation in myocardial cellsReference Borradaile and Schaffer 54 , Reference Christoffersen, Bollano and Lindegaard 34 and defects in cardiac insulin signaling.Reference Martins, Vieira, de Souza and Moura 53
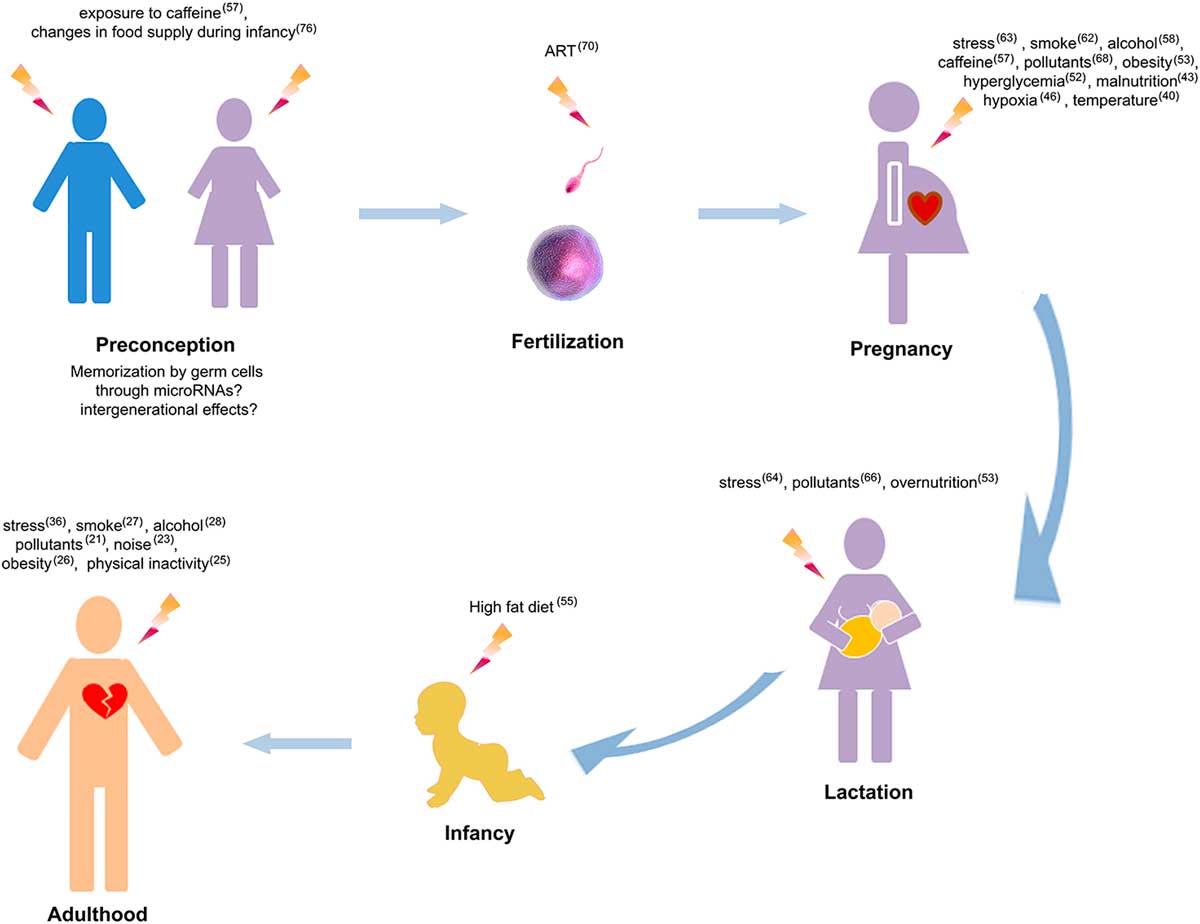
Fig. 1 Lifelong environmental challenges that alter heart functions. Numbers indicate the references of studies associating environmental challenges and cardiac alterations. ART, assisted reproductive technologies.
In the early origin of cardiac dysfunctions, the existence of sensitive developmental windows of exposure had been only addressed in a few studies which highlighted the impact levels according to the time of exposure. In the case of high-fat diet, adult cardiac alterations including increased heart rate were described when the exposure occurred after weaning.Reference Fiorino, Americo and Muller 55 Another study which compared in utero and post-weaning period of exposure to a high-fat high-sugar diet showed that exposure in the post-weaning period has a more profound effect on offspring weight gain and glucose tolerance than maternal overnutrition.Reference King, Norman, Seckl and Drake 56 The analyses of metabolic alterations suggest that post-weaning period is critical in the altered programming of offspring health by nutrition. These data emphasize the importance of optimizing early life nutrition in offspring of both obese and lean mothers. However, the effects of those exposures were studied on rodent where postnatal period correspond developmentally to the late gestation period in human. The critical developmental window of exposure thus, remains to be investigated in human. In the case of caffeine, mice exposed in utero to an equivalent to two to four cups of coffee at an early embryonic stage, develop a greater risk of dilated cardiomyopathy in adulthood. When the exposure occurs at a later embryonic stage, that is to say when germ cells develop, inter-generational (in F2 and F3) hypertrophic cardiomyopathy is induced.Reference Fang, Poulsen, Rivkees and Wendler 57 During this critical developmental period, the high sensitivity of the heart to toxicants is observed in the response to low doses of the toxicant. Indeed, some factors can have no impact at a determined dose level in adulthood whereas it can trigger profound effects when the exposure occurs early in life, such as alcohol. While in adulthood the effects of alcohol consumption on cardiac health depends on the dose, during pregnancy, even relatively low doses of alcohol consumed can be detrimental to long-term cardiac health in the offspring by inducing ventricular hypertrophy and fibrosis in adulthood.Reference Nguyen, Probyn and Campbell 58 Despite public health warnings, younger women continue to smoke during pregnancy.Reference Reitan and Callinan 59 Gestational exposure to nicotine induces higher risk of perinatalReference Butler, Goldstein and Ross 60 and obstetric complications.Reference Coste, Job-Spira and Fernandez 61 Fetal exposure to nicotine induces alteration in the cardiac conduction system with disrupted heart rate and electrical conductionReference Ton, Biet, Delabre, Morin and Dumaine 62 which has been suggested as an underlying mechanism for sudden death. A number of studies showed that fetal heart is sensitive to maternal stress.Reference Makino, Matsuda and Yoneyama 63 Stress experienced postnatally, during maternal separation, increases risk of heart diseases in animalsReference Sanders and Anticevic 64 (e.g. increased heart rate response) and humansReference Alastalo, Raikkonen and Pesonen 65 (e.g. increased risk of developing coronary heart disease) as observed in children born in Helsinki between 1934 and 1944 and who were separated from their parents. The developing cardiovascular system is a sensitive target of many environmental pollutants, including dioxins,Reference Aragon, Kopf, Campen, Huwe and Walker 66 , Reference Thackaberry, Nunez, Ivnitski-Steele, Friggins and Walker 67 and a plasticizer, the Bisphenol A (BPA)Reference Chapalamadugu, Vandevoort, Settles, Robison and Murdoch 68 which induce long-term alterations in offspring heart such as hypertrophy. Furthermore, when associated with a second hit during life (like unbalanced diet), prenatal exposure to toxicant has been shown to produce a more pronounced phenotype in adulthood. For example, when coupled with postnatal overfeeding, BPA treatment produced a stronger phenotype with significant increase in inter-ventricular septal thickness indicating that BPA pre-treatment alters the trajectory of cardiac function differentially from overfeeding alone that may be adaptive v. maladaptive.Reference MohanKumar, Rajendran and Vyas 69
Assisted reproductive technologies (ARTs) are widely used in infertility treatment. Emerging evidence indicates that ART is a risk factor for heart diseases in offspring.Reference Valenzuela-Alcaraz, Crispi and Bijnens 70 , Reference Scherrer, Rexhaj, Allemann, Sartori and Rimoldi 71 Hypotheses attribute the effect of ART on cardiovascular health to embryo manipulation in suboptimal culture conditions or ART-related fetal insults, or other parental factors. For instance, the advanced parental age was suggested to be a risk factor of heart malformation in the offspring.Reference Materna-Kiryluk, Wisniewska and Badura-Stronka 72 , Reference Su, Yuan, Huang, Olsen and Li 73 Strikingly, parental exposures not only influences cardiac health at an inter-generational level, but also at a trans-generational level.Reference Keverne 74 While mother contribution is well described through the transmission of biomolecules (nutrients or hormones), environmental influences (temperature) or behavior (anxiety) to their offspring, the paternal contribution has been for a long time not considered and under debate. Recently, increasing evidence is pointing out the role of paternal contribution to offspring health.Reference Hughes 75 For example, the Overkalix cohort study (in Sweden) showed that men who had experienced famine before puberty were less likely to have grandsons with cardiovascular disease than men who had plenty to eat.Reference Kaati, Bygren and Edvinsson 76 In inter- and trans-generational transmission of acquired traits, epigenetic marks have been highlighted as underlying molecular bases. The discovery of epigenetic mechanisms including micro-RNAsReference Bartel 77 that induce specific phenotypes without change in DNA sequence improved our understanding of DOHaD field while for many years the early developmental period was considered to be controlled by the genetic program. Indeed, a single genome gives rise in development to over 100 different types of cells. The same genome is present in all of these cells but their functions are remarkably different. This is achieved through different epigenetic programming in each of these cell types. As such, the early epigenome adds powerful layers of diversity to the biologic predisposition generated by the genome.
Micro-RNAs in heart development and diseases
Heart development is orchestrated by epigenetic processes. The major epigenetic features include DNA methylation, post-translational histone modifications and non-coding RNAs, including small non-coding RNAs (e.g. piRNAs and micro-RNAs). In close interaction with DNA methylation and histone post-translational modifications, non-coding RNAs regulate gene expression. These mechanisms regulate the critical steps of heart development involving coordinated cellular proliferation, migration, differentiation and programmed cell death, structural remodeling,Reference Martinez, Gay and Zhang 78 and the control of metabolic homeostasis through the regulation of glucose and lipid metabolism, and insulin signaling.Reference Sandovici, Smith and Nitert 79 Recent studies have revealed important roles for micro-RNAs in heart developmentReference Yan and Jiao 80 and diseaseReference Latronico and Condorelli 81 – Reference Trajkovski, Hausser and Soutschek 83 (Table 1).
Table 1 Micro-RNAs associated to cardiac pathophysiology

HF: heart failure, ARRH: arrhythmia, CAD: coronary artery disease, CHD: congenital heart disease, VALV: valvular disease, FIBRO: fibrosis, HYPER: hypertrophy, MI: myocardial infarction, DIA: diabetic heart disease, MC: myocarditis.
Non exhaustive list of micro-RNAs that have been described in animal and/or human models of cardiac diseases. Orange color squares indicates micro-RNAs that were reported in specific cardiac disease. The reference of the study highlighting the involvement of micro-RNA in specific cardiac disease is indicated in each orange square.
Micro-RNA biogenesis and mode of action
While only 1–2% of the genome encode for proteins, the main part gives rise to non-coding RNAs (ncRNAs) including micro-RNAs. Micro-RNAs originate from sequences disseminated throughout the genome, from introns or intergenic regions. Mature micro-RNAs are small nucleotide sequences (19–24 nucleotides in length). Some micro-RNAs are involved in pathways that are conserved throughout phylogeny while micro-RNA diversity correlates with speciation. The number of micro-RNA and targets of micro-RNAs reveals morphological complexities observed in animals.Reference Lee, Risom and Strauss 84 Importantly, given that ~60% of the human genes are under their control, micro-RNAs are master regulators at the post-transcriptional levelReference Friedman, Farh, Burge and Bartel 85 and play important roles in many biological processes, including development, differentiation, proliferation, apoptosis, metabolism and tissue remodeling.
Micro-RNA biogenesis is based on a series of steps that go from transcription of primary micro-RNAs (pri-micro-RNAs) to the biologically active, mature micro-RNAs (Fig. 2). First, DNA sequences are transcribed into pri-micro-RNAs mainly through the action of RNA polymerase II,Reference Lee, Kim and Han 86 and to a less extent by RNA Polymerase III.Reference Borchert, Lanier and Davidson 87 The transcribed pri-micro-RNAs are then cleaved by DROSHA and its cofactor DiGeorge critical region 8 (DGCR8) in the nucleus generating micro-RNA precursors about 60–70 nt called pre-micro-RNAs.Reference Han, Lee, Yeom, Kim, Jin and Kim 88 These pre-micro-RNAs shuttle into the cytoplasm by interacting with the nuclear pore complex Exportin-5.Reference Yi, Qin, Macara and Cullen 89 Once in the cytoplasm, the hairpin structures, known as pre-micro-RNAs, undergo further processing by the DICER–TAR RNA-binding protein 2 (TRBP) complex to produce fully processed RNA duplexes (around 22 nucleotides in length) comprising the mature micro-RNAs and the micro-RNAs* (also known as passenger strands).Reference Wilson, Tambe, Kidwell, Noland, Schneider and Doudna 90 Each micro-RNA strand is incorporated into an RNA-induced silencing complex (RISC) comprising an Argonaute (AGO) protein that binds to the target mRNA and degrades the passenger strand.Reference Maniataki and Mourelatos 91 The mature micro-RNA guides the RISC to target sequences located mainly in the 3’UTR of the target messenger RNA leading to an inhibition of its translation or to its degradation. In addition to their classical roles, micro-RNAs regulate gene expression through promoter targeting and translational activation.Reference Place, Li, Pookot, Noonan and Dahiya 92 , Reference Bruno, Karam and Huang 93 Binding to the 5’UTR and to exon sequence have also been described.Reference Atambayeva, Niyazova and Ivashchenko 94 Interestingly, the micro-RNA biogenesis machinery is regulated by hypoxia, hormonal and dietary changes.Reference Davis and Ross 95 – Reference Kulshreshtha, Ferracin, Negrini, Calin, Davuluri and Ivan 97 More recently, extracellular, circulating micro-RNAs have been described as highly stable, and as potential blood-based biomarkers for diseases,Reference Siddeek, Inoubli and Lakhdari 98 including heart diseases. We will focus this review on the involvement of micro-RNAs in heart health and disease and especially their involvement in heart disease programming.

Fig. 2 Micro-RNAs biogenesis. Micro-RNAs biogenesis involves different steps. Pri-micro-RNAs are transcribed from inter- and intra-genic regions. They are then processed into pre-micro-RNAs and double-stranded micro-RNAs. The mature single-stranded micro-RNAs binds to the UTR ends of targets messenger RNAs (mRNAs) to regulate their levels.
Micro-RNAs regulate cardiac development and function
Advances in micro-RNA analysis such as next generation high depth sequencing techniques have highlighted their critical role in heart functions. As revealed by conditional knock out of Dicer in cardiac tissue, defects in micro-RNA biogenesis affect both embryonic development and postnatal cardiac maintenance and function.Reference da Costa Martins, Bourajjaj and Gladka 99 – Reference Bernstein, Kim and Carmell 102 One important role of micro-RNAs in heart development and function is linked to their ability to regulate the cardiac transcriptional pathways that maintain a restricted and specific pattern of gene expression.Reference Wang 103 Experimental studies on animals and analyses of human samples address specific roles of different micro-RNAs.
Several clinical and experimental studies have described the importance of dysregulation of micro-RNAs in the origin of cardiac disorders (Table 1), such as coronary artery disease,Reference Rottiers and Naar 104 cardiac fibrosis and hypertrophy.Reference Yang, Li and Wang 105 Among them, a few micro-RNAs, including miR-1, miR-133, miR-208 and miR-499 which regulate cell proliferation, differentiation and apoptosis in cardiac tissue from the early developmental stages, have been described as key players in heart disease development. Their alterations have been reported in cardiac hypertrophy, myocardial infarction, cardiac arrhythmia and heart failure.Reference Wang and Cai 106 MiR-1, the first micro-RNA that has been implicated in heart development, exerts its action through the targeting of multiple pathways. For instance, miR-1 negatively regulates proliferation and mediates cell cycle withdrawal by targeting heart and neural crest derivatives expressed 2 (HAND2),Reference Zhao, Samal and Srivastava 107 cell division cycle 42 (CDC42)Reference Qian, Wythe and Liu 108 and the serine/threonine kinase PIM-1.Reference Chen, Yin and Jiang 109 MiR-1 levels are also key player in cardiomyocyte differentiation and cell fate decision by down-regulating Notch ligand delta 1 (DLL1).Reference Kwon, Han, Olson and Srivastava 110 In addition, miR-1 regulates contractility of cardiomyocyte by controlling calcium homeostasisReference Ali, Huang and Maher 111 and electrical conduction.Reference Zhao, Ransom and Li 112 Indeed, it down-regulates Iroquois Homeobox 4 and 5, IRX4 and IRX5 which are involved in the regulation of the cardiac repolarisation gradientReference Besser, Malan and Wystub 113 and genes that are involved in gap junction and iron channel such as GJA1,Reference Curcio, Torella and Iaconetti 114 or the cardiac L-type calcium channel gene CACNA1C (CAV1.2).Reference Rau, Freyermuth and Fugier 115 MiR-1 has also displayed its important role in diabetic cardiac dysfunction. In a mouse model of streptozotocin-induced diabetes, miR-1 down-regulation may be involved in the development of cardiac hypertrophy.Reference Costantino, Paneni, Luscher and Cosentino 116 qA study performed on diabetic patients’ cohorts highlights the potential of serum miR-1 as a biomarker of myocardial steatosis.Reference De Gonzalo-Calvo, Van der Meer and Rijzewijk 117
Furthermore, micro-RNAs have been highlighted as sensitive biomarkers of cardiac dysfunction and prognostic markers for diseases. Indeed, changes in their profile in diabetic individuals with normal ejection fraction are detected before clinical manifestations of heart diseases,Reference Rawal, Ram and Coffey 118 , Reference Zampetaki, Kiechl and Drozdov 119 and are dynamic in response to therapeutic treatment (i.e. diabetic cardiac microangiopathy).Reference Flowers, Aouizerat and Abbasi 120 Just as gene polymorphism, micro-RNAs polymorphism can represent risk for heart diseases. For instance, meta-analyses performed on a total of 13 related studies involving 8120 patients and 8364 controls suggest that polymorphism in miR-146a (rs2910164), microR-196a2 (rs11614913) and miR-499 (rs3746444) are associated with coronary heart disease risk.Reference Liu, You, Zhou and Zhang 121
Micro-RNAs at the origins of cardiac disorders induced by environment, lifestyle and diet
Deregulation of micro-RNAs profile has been suggested as a potential mechanism at the origin of heart diseases induced by environment and lifestyle.Reference Lock, Botting, Tellam, Brooks and Morrison 122 In cardiac alterations induced by fetal hypoxia, a few micro-RNAs have been identified. Among these, miR-210 was highlighted at the origin of fetal rat cardiomyocytes death induced by hypoxia through the suppression of glucocorticoid receptor (NR3C1).Reference Martinez, Ma, Dasgupta, Meng and Zhang 123 MiR-210 is regulated by the binding of hypoxia-inducible factor 1-alpha (HIF-1-α) to its promoter, and due to its consistent and powerful response to hypoxia, miR-210 is termed as the master micro-RNA regulating the cellular response to hypoxia.Reference Chan, Banerjee, Choi and Sen 124 , Reference Huang and Zuo 125 Besides miR-210, other micro-RNAs such as miR-2285, miR-34, miR-192, miR-449, miR-200 families and miR-199a-214 cluster, have been associated with hypoxia, for example in high-altitude adaptationReference Guan, Long and Ma 126 or in pathological condition.Reference El Azzouzi, Leptidis and Dirkx 127 Changes in micro-RNA profile have been proposed as underlying mechanisms in the cardiac alterations driven by maternal exposure to dietary challenges, through the deregulation of cell death, growth and proliferation in the fetal heart.Reference Maloyan, Muralimanoharan and Huffman 128 In animals exposed to high-fat diet, a number of pathways altering cardiomyocyte metabolism have been described. For instance, mice exposed to high-fat diet exhibit cardiac lipotoxicity through the dysregulation of a major cellular sensor of energy availability, AMP-activated protein kinase (AMPK), and its upstream regulators, the calcium-binding protein 39 (CAB39) and the liver kinase B1 (LKB1).Reference Kuwabara, Horie and Baba 129 MiR-451 has been shown to regulate this LKB1/AMPK pathway and cardiac lipotoxicity through the targeting of CAB39.Reference Kuwabara, Horie and Baba 129 Similarly, dysregulated glycogen synthase kinase 3 (GSK3B) and glucose transporter 4 (GLUT4), involved in the insulin signaling pathway, have been associated with alterations in miR-29c, miR-21a-3p, miR-29c-3p, miR-144–3p and miR-195a-3p levels in the heart of animals exposed to high-fat diet.Reference Guedes, Franca and Lino 130 Exposure to maternal hyperglycemia, induces cardiovascular dysfunctions in offspring. Evidence suggests that those dysfunction are due to altered levels of enhancer of zeste homolog 2 (EZH2) driven by miR-101 in fetal endothelial cells.Reference Floris, Descamps and Vardeu 131 Interestingly, miR-101 and EZH2 have been highlighted in other models of fetal programming of adult diseases (e.g. infertility) driven by early exposure to endocrine disruptors.Reference Siddeek, Lakhdari and Inoubli 132 Not only in utero exposure affects cardiac micro-RNAs in short term,Reference Maloyan, Muralimanoharan and Huffman 128 but also in long term.Reference Fernandez-Twinn, Blackmore and Siggens 133 As such, in mice, a maternal obesogenic diet during gestation and lactation induces miR-133a alteration in the offspring heart at 8 weeks of age associated with cardiac hypertrophy.Reference Fernandez-Twinn, Blackmore and Siggens 133 Furthermore, as highlighted by a recent study, the impact of in utero exposure to dietary challenges on long-term cardiac function depends on the timing of the exposure during development.Reference Wing-Lun, Eaton and Hur 134 In this study, the comparison between prenatal exposure to maternal obesity and/or postnatal exposure to a Western diet on micro-RNA expression profiles showed that many more cardiac micro-RNA implicated in various cardiac pathologiesReference Wing-Lun, Eaton and Hur 134 are altered in response to a relatively short period of postnatal overnutrition, rather than by a longer period of prenatal overnutrition.Reference Wing-Lun, Eaton and Hur 134 Furthermore, cardiac micro-RNAs not only regulate functions, but also regulate metabolism at the body level. Indeed, miR-208 which cardiac levels are increased in high-fat diet mice, regulates the mediator complex subunit 13 (Med13) involved in the regulation energy balance in the heart.Reference Grueter, Van Rooij and Johnson 135 Cardiac inhibition of miR-208 is sufficient to confer resistance to obesity in high-fat diet mice.Reference Grueter, Van Rooij and Johnson 135 This observation highlights a novel role of cardiac micro-RNA, as a hormone-like controller on whole-body metabolism. In the effects driven by in utero exposure to environmental toxicants (such as BPA) on cardiac remodeling, a study performed in monkeys highlighted the involvement of miR-205 and miR-224.Reference Chapalamadugu, Vandevoort, Settles, Robison and Murdoch 68 The cellular pathways involved have been more detailed in a model of cardiomyocyte cell line exposed to a persistent organic pollutant, the polychlorinated biphenyls (PCBs). As revealed in this in vitro model, the deregulation of micro-RNAs involved in cardiomyocyte differentiation is a potential underlying mechanism in PCB driven cardiac dysfunction.Reference Zhu, Yu and Zhu 136
Micro-RNAs, a target for the reversal of heart disease programming?
Given their key function in gene regulation, micro-RNAs provide promising therapeutic targets.Reference Christopher, Kaur, Kaur, Kaur, Gupta and Bansal 137 Advances in micro-RNA chemistry and delivery technologies allowed the development of powerful tools for in vivo regulation of micro-RNAs.Reference Rupaimoole and Slack 138 In the treatment of post-myocardial infarction, cardiac remodeling and dysfunction, hypertrophy, heart failure, atherosclerosis and myocarditis,Reference Montgomery, Hullinger and Semus 139 , Reference Rayner, Sheedy and Esau 140 animal models of in vivo delivery of micro-RNA mimics or anti-miRs show the potential of micro-RNA based therapy. For instance, the inhibition of micro-RNAs (miR-128,Reference Zeng, Li, Wen and Bi 141 miR-92a,Reference Jiang, Ji and Luo 142 miR-26a,Reference Zhang, Qin and Zhang 143 miR-15Reference Hullinger, Montgomery and Seto 144 or miR-34Reference Bernardo, Gao and Winbanks 145 families) involved in cardiomyocyte cell death induced by myocardial ischemia reduces infarct size after ischemia-reperfusion and augments the recovery of heart function. Pharmacological inhibition of miR-140 which is involved in mitochondrial fission reduces infarct size after acute myocardial infarction in mice.Reference Li, Li and Jiao 146 Inhibition of miR-21Reference Bonci 147 or miR-25Reference Wahlquist, Jeong and Rojas-Munoz 148 prevents heart failure development in mice by restoring cardiac function, reducing fibrosis and normalizing cardiomyocyte cell size. Conversely, micro-RNAs such as miR-210Reference Hu, Huang and Li 149 which protects cardiomyocytes and vasculature, when injected intra intramyocardially, reduce cell death and improve cardiac function and angiogenesis after acute myocardial infarction.Reference Wang, Zhang and Li 150 Research into the role of micro-RNAs in heart disease holds great promise for future therapeutic applications. However, many questions on their delivery and duration of action in human remain. Thus, modification in lifestyle and diet remains the first choice in the micro-RNAs modulation. Interestingly, exercise training improves cardiac autonomic control, cardiac function and arrhythmogenesis in rats with preserved ejection fraction heart failure.Reference Andrade, Arce-Alvarez and Toledo 151 Recently, physical exercise has been shown to modulate cardiac micro-RNAs which are possibly involved in exercise mediate cardioprotection. Modification in these micro-RNAs levels driven by exercise can be detected in the blood, representing potential biomarkers of cardiorespiratory fitness.Reference Denham and Prestes 152 Apoptosis-related micro-RNAs and their downstream proteins in heart can be influenced by swimming training.Reference Zhao and Ma 153 Circulating micro-RNA levels (miR-1, miR-133 and miR-206) are elevated with marathon runningReference Lew, Pearson, Schwenke and Katare 154 and with moderate exercise in pathological conditions like myocardial infarction (miR-1 and miR-214) which are associated with the normalization of Ca2+ handling and left ventricular compliance in infarcted hearts.Reference Melo, Barauna and Neves 155
Recent evidence showed that hydrogen sulfide (H2S) has the potential to protect the heart against diseases.Reference Barr, Shimizu, Lambert, Nicholson and Calvert 156 , Reference Khatua, Adela and Banerjee 157 H2S is a gaseous mediator along with nitric oxide and carbon monoxide, and is produced endogenously from cysteine metabolism in mammalian tissues. Low plasma levels of H2S have been described in type 2 diabetic patients,Reference Jain, Bull and Rains 158 in high-fat diet treated miceReference Peh, Anwar, Ng, Atan, Kumar and Moore 159 and streptozotocin-treated rats.Reference Jain, Bull and Rains 158 Recent evidence showed that H2S has the potential to protect the heart against diseases.Reference Barr, Shimizu, Lambert, Nicholson and Calvert 156 , Reference Khatua, Adela and Banerjee 157 Exogenous H2S treatment mitigates cardiomyopathy induced by diabetes and protects the heart from I/R injury. Interestingly, H2S has been demonstrated to regulate micro-RNAsReference Kesherwani, Nandi, Sharawat, Shahshahan and Mishra 160 in cardiomyocyte cell lines. The preconditioning of myocardial I/R with H2S decreases miR-1-mediated apoptosis.Reference Kang, Hong and Xiao 161 However, in the context of early programming of heart diseases, little is known about the potential of micro-RNAs in the reversal of the pathology. In a rat model of adult cardiac hypertrophy induced by maternal undernutrition, specific cardiac micro-RNA profiles are exhibited and are associated with genes involved in inflammation and cardiovascular development. When the offspring is treated with growth hormone, the cardiac hypertrophy is reversed,Reference Gray, Li, Patel, Reynolds and Vickers 162 accompanied by an up-regulation of cardiac let-7 highlighting the potential of let-7 micro-RNA family in the reversal of cardiac pathophysiology induced by maternal undernutrition. Interestingly, recent study showed that short caloric restriction in adulthood can restore cardiac function in mice that were exposed postnatally to overnutrition.Reference Li, Guenancia and Rigal 163 However, the role of micro-RNAs in the reversal of cardiac alteration programming by nutritional approach remains to be investigated.
Future perspective
How environmental challenges during early life can alter and shape offspring cardiac functions through alterations in micro-RNAs is an intense area of research. However, several questions still need to be elucidated. While evidence exists for the influence of early life insults on micro-RNAs and pathophysiology in adipose tissue, brain, liver and pancreas, evidence for the existence of a causative link between environmental challenges, nutritional constraints, or lifestyle challenges, and micro-RNAs and developmental programming of cardiovascular diseases is still limited. Furthermore, the sensitive developmental windows of exposure and the role of micro-RNAs in gender differences need to be clarified for the different types of challenges. Finally, the targeting of micro-RNAs through nutritional, exercise or pharmacological approaches would provide valuable information that would allow better advice for early prevention, and promote appropriate changes to maximize later life health benefits.
Acknowledgments
The authors acknowledge Suzan Arslan for her valuable help in English editing.
Financial Support
This work was supported by the Centre Hospitalier Universitaire Vaudois (CHUV).
Conflicts of Interest
None.