1. Introduction
The Rodinia supercontinent was assembled between 1.3 and 0.9 Ga, before breaking up between 850 and 740 Ma (Li et al. Reference Li, Bogdanova, Collins, Davidson, De Waele, Ernst, Fitzsimons, Fuck, Gladkochub, Jacobs, Karlstrom, Lu, Natapov, Pease, Pisarevsky, Thrane and Vernikovsky2008). Several blocks that were formerly part of Rodinia record multiple episodes of anorogenic magmatism at 850–740 Ma, including South China, Tarim, North America, India, Southern Africa and Australia (Powell et al. Reference Powell, Preiss, Gatehouse, Krapez and Li1994; Park et al. Reference Park, Buchan and Harlan1995; Preiss, Reference Preiss2000; Frimmel et al. Reference Frimmel, Zartman and Späth2001; Ling et al. Reference Ling, Gao, Zhang, Li, Liu and Cheng2003; Li et al. Reference Li, Bogdanova, Collins, Davidson, De Waele, Ernst, Fitzsimons, Fuck, Gladkochub, Jacobs, Karlstrom, Lu, Natapov, Pease, Pisarevsky, Thrane and Vernikovsky2008; Wang et al. Reference Wang, Li, Li, Li and Zhang2011; Xu et al. Reference Xu, He, Zhang, Zhang, Wang and Cai2013; Zhang et al. Reference Zhang, Zou, Li and Wang2013; McClellan & Gazel, Reference McClellan and Gazel2014; Wan et al. Reference Wan, Zeng, Asimow, Zeng, Peng, Xu, Wei, Liu, Lu and Chang2019).
The Qilian Orogen in the northeastern Tibetan Plateau is an important part of the Qin–Qi–Kun Central China Orogenic System. It is bordered by the North China Craton to the northeast, the South China Craton to the southeast and the Tarim Craton to the northwest (Fig. 1a) (Xiao et al. Reference Xiao, Windley, Yong, Yan, Yuan, Liu and Li2009; Song et al. Reference Song, Niu, Su and Xia2013). The orogen is subdivided into the South Qilian Belt (SQB), Central Qilian Block and North Qilian Belt, with the SQB thought to preserve evidence of 786–713 Ma magmatism (Ma et al. Reference Ma, Jia, Li, Ma, Lei, Ren and Xu2017; Bai et al. Reference Bai, Zhuan, Mao, Chen and Li2019; Wang et al. Reference Wang, Li, Hu, Yang, Guo, Yan, Ge and Ji2019; Ji et al. Reference Ji, Li, Huang, Wang and Wang2020). However, the petrogenesis and tectonic setting of this magmatism remain unclear, with two contrasting models proposed to explain these events to date. The first of these suggests that magmatism occurred in a continental rift setting as a result of mantle plume activity associated with the final break-up of Rodinia (Bai et al. Reference Bai, Zhuan, Mao, Chen and Li2019; Ji et al. Reference Ji, Li, Huang, Wang and Wang2020). The second model suggests that magmatism occurred in arc-type tectonic settings (Ma et al. Reference Ma, Jia, Li, Ma, Lei, Ren and Xu2017; Wang et al. Reference Wang, Li, Hu, Yang, Guo, Yan, Ge and Ji2019). Further research is needed to precisely constrain the geochemical characteristics and affinities of these magmatic events, and their geodynamic setting.

Fig. 1. (a) Tectonic framework of China and location of the study area (modified after Song et al. Reference Song, Niu, Su and Xia2013). (b) Tectonic subdivision of the northeastern Tibetan Plateau, showing the tectonic location of the SQB (modified after Lu et al. Reference Lu, Li, Zhang and Niu2008). Igneous rocks age data sources: 738–733 Ma (Mao et al. Reference Mao, Zhang, Yang, Song, Wu and Zuo1997); 751 ± 4 Ma (Su et al. Reference Su, Hu, Zhang and Fu2004); 776–774 Ma (Tseng et al. Reference Tseng, Yang, Wan, Liu, Wen, Lin and Tung2006); 764–675 Ma (Song et al. Reference Song, Liu, Li, Zhang, Liang and Zhen2016); 713 ± 4 Ma (Wang et al. Reference Wang, Li, Hu, Yang, Guo, Yan, Ge and Ji2019); 786 ± 5 Ma (Ji et al. Reference Ji, Li, Huang, Wang and Wang2020); 824–713 Ma (Xu et al. Reference Xu, Wang, Chen, He, Wu and Gao2008); 738 ± 11 Ma (this study); 740–736 Ma (Bai et al. Reference Bai, Zhuan, Mao, Chen and Li2019); 730 ± 3 Ma (Ma et al. Reference Ma, Jia, Li, Ma, Lei, Ren and Xu2017); 800 Ma (Li et al. Reference Li, Lu, Wang, Xiang and Zhen2003); 744 ± 28 Ma (Lu et al. Reference Lu, Yu, Jin, Li and Zhen2002); 780–768 Ma (Yang et al. Reference Yang, Wu, Zhang, Shi, Meng, Wooden and Yang2006); 795–748 Ma (Chen et al. Reference Chen, Liu, Sun and Liou2009); 796 ± 41 Ma (Ren et al. Reference Ren, Zhang, Liu, Zhou and Feng2011); 733 ± 6 Ma (Ren et al. Reference Ren, Liu, Zhou, Feng, Zhang, Dong and Qin2010). (c) Geological map of the Xialanuoer area (modified after Qin, Reference Qin2018) showing sample locality.
This study presents new zircon U–Pb–Hf isotopic and whole-rock geochemical data for the Xialanuoer gabbros of the central SQB. Combining these new data with the results of previous research allows the Mid-Neoproterozoic tectonic setting of the SQB to be better constrained, and provides insights into the Mid-Neoproterozoic tectonic evolution of the Qilian – Qaidam – East Kunlun region of the northeastern Tibetan Plateau.
2. Geological background
The Qilian Orogen is bounded by the Altyn fault to the northwest, extends to the east into the Qinling Orogen, and borders the Quanji and Alxa blocks to the south and north, respectively (Fig. 1b).
The North Qilian Belt represents a subduction-related accretionary complex formed by the Palaeozoic closure of the Proto-Tethys North Qilian Ocean between the Alxa and Central Qilian blocks (Xia et al. Reference Xia, Li, Yu and Wang2016; Wang et al. Reference Wang, Xu, Santosh, Xu, Deng and Fu2017; Peng et al. Reference Peng, Yu, Li, Zhang, Liu, Li and Santosh2019). It comprises Neoproterozoic to Early Palaeozoic ophiolite sequences, high-pressure (HP) metamorphic belts, island arc volcanic rocks and granitoid plutons, Silurian flysch and Devonian molasse deposits, and Carboniferous to Triassic sedimentary cover sequences (Yang et al. Reference Yang, Du, Cawood and Xu2009; Xu et al. Reference Xu, Du, Cawood, Guo, Huang and An2010; Yu et al. Reference Yu, Zhang, Qin, Sun, Zhao, Cong and Li2015; Qin et al. Reference Qin, Zhang, Feng, Zhang, Gao and Chen2021).
The Central Qilian Block is a Precambrian microcontinent comprising Meso–Neoproterozoic greenschist- to amphibolite-facies metasedimentary rocks, metamorphosed plutons and dolomitic marbles, all of which are covered by Palaeozoic sedimentary sequences (Hou et al. Reference Hou, Zhang, Zhang, Zhao and Zhu2005; Xu et al. Reference Xu, Zhang and Liu2007; Tung et al. Reference Tung, Yang, Liu, Zhang, Yang, Shau and Tseng2013).
The SQB is dominated by the Neoproterozoic–early Paleozoic tectonic melange (Balonggonggaer Formation), which comprises mainly schists and greywackes (Ji et al. Reference Ji, Yu, Li, Huang and Wang2018; Qin, Reference Qin2018; Li et al. Reference Li, Wang, Li, Meert, Peng and Zhang2019). Although the maximum depositional age of the schists in the Balonggonggaer Formation is constrained by the youngest detrital zircon U–Pb age peaks at 824–720 Ma (Li et al. Reference Li, Wang, Li, Meert, Peng and Zhang2019; Qin, Reference Qin2018), the existence of older rocks can't be ruled out. Moreover, the belt contains small volumes of locally exposed Mid-Neoproterozoic magmatic rocks that are dominated by 786–713 Ma basalts and gabbros (Ma et al. Reference Ma, Jia, Li, Ma, Lei, Ren and Xu2017; Bai et al. Reference Bai, Zhuan, Mao, Chen and Li2019; Wang et al. Reference Wang, Li, Hu, Yang, Guo, Yan, Ge and Ji2019; Ji et al. Reference Ji, Li, Huang, Wang and Wang2020). The SQB also contains voluminous Palaeozoic (457–418 Ma) granitoids associated with minor volcanic rocks (Liao et al. Reference Liao, Hu, Zhang, Yu and Guo2014; Niu et al. Reference Niu, Huang, Deng, Xu, Chen, Ji and Li2016; Zhang et al. Reference Zhang, Xu, Gong, Fen, Han, Zhang, Zhou and Huang2016; DL Li et al. Reference Li, Sun, Zhao, Sun, Yang, Tian, Li and Yang2018 a) that formed as a result of the early Palaeozoic closure of the Proto-Tethys South Qilian Ocean (Fu et al. Reference Fu, Yan, Wang, Buckman, Aitchison, Niu, Cao, Guo, Li, Li and Li2018; Yan et al. Reference Yan, Fu, Aitchison, Niu, Buckman and Cao2019).
3. Sample descriptions
This study focuses on a gabbroic pluton that crops out ∼80 km northwest of Delingha in the central SQB in the Xialanuoer area. The pluton is relatively small (1 km × 0.5 km) and elliptical and preserved in the Balonggonggaer Formation (Fig. 1c). A total of seven samples (DLH-1 to 7) were collected from the gabbroic pluton; details of sampling locations and mineral assemblages are provided in Table 1. All of the samples are metagabbro with medium- to fine-grained granular textures and massive structures. They are composed of olivine (10–20 %), clinopyroxene (40–50 %), plagioclase (35–45 %) and minor minerals of sphene and chlorite. Granular olivine and subhedral plagioclase are included in subhedral clinopyroxene (Fig. 2), suggesting the earlier crystallization of olivine and plagioclase. Clinopyroxene edges are often fragmented and, although the gabbros have undergone greenschist-facies metamorphism, they retain clear primary magmatic textures.
Table 1. Summary of the sample locality, lithology and mineral assemblage for the Mid-Neoproterozoic gabbros in the Xialanuoer area


Fig. 2. Photographs and photomicrographs of the Mid-Neoproterozoic gabbros from the Xialanuoer area. ol = olivine; pl = plagioclase; cpx = clinopyroxene.
4. Analytical methods
All of the analyses were undertaken at the State Key Laboratory of Continental Dynamics of Northwest University, Xi’an, China. Zircon grains were extracted from gabbro samples using standard separation methods before hand-picking under a binocular microscope. These zircon grains were mounted in epoxy resin before polishing to expose zircon interiors. The zircon grains were then imaged using reflected and transmitted light optical microscopy and cathodoluminescence (CL) using a Gatan MonoCL3 CL instrument coupled with a scanning electron microscope.
In situ U–Pb dating was performed using laser ablation – inductively coupled plasma – mass spectrometry (LA-ICP-MS) employing a MicroLasTM Beam Delivery Systems Analyte Excite 193 nm ArF excimer LA system coupled to a Perkin Elmer/SCIEX Elan 6100 ICP-MS instrument. The ablated materials were delivered to the torch in the ICP-MS by high-purity helium gas to ensure efficient aerosol transport. A NIST 610 silicate glass standard was used to tune the ICP-MS to ensure maximum signal sensitivity for high masses (Pb, Th, U; better than 2000 cps μg−1 g−1). The analysis used a beam diameter of 32 μm and a laser frequency of 6 Hz, and the NIST 610 U+ and Th+ ion-signal intensity ratio (using 238U and 232Th ≈ 1) was used as an indicator of complete vaporization (Günther & Hattendorf, Reference Günther and Hattendorf2005), with oxide formation monitored using ThO+/Th+ (<0.5 %). Laser ablation employed time-resolved analysis using single laser spots, with the analysed elements acquired using a peak jumping mode. Dwell times were 10 ms for Th, 15 ms for U, Pb and Ti, and 6 ms for all other elements. Dual-pulse and analogue counting detector modes were automatically converted according to intensity during analysis with pulse/analogue (P/A) factors for each element auto-tuned to ensure best accuracy and efficiency prior to routine analysis. 207Pb/206Pb, 206Pb/238U, 207Pb/235U and 208Pb/232Th ratios were calculated using the GEMOC-developed GLITTER 4.0 software package from Macquarie University, with integrated ratio drift during individual runs corrected to remove both instrumental mass bias and depth-dependent elemental and isotopic fractionations by calibration against a matrix-matched Harvard zircon 91500 standard analysed using the same operating conditions (Wiedenbeck et al. Reference Wiedenbeck, Allé, Corfu, Griffin, Meier, Oberli, Quadt, Roddick and Spiegel1995). GJ-1 is also a standard sample with a recommended 206Pb/238U isotopic age of 603.2 ± 2.4 Ma (XM Liu et al. Reference Liu, Gao, Diwu, Yuan and Hu2007). The concentrations of U, Th, Pb and other trace elements were calibrated using 29Si as an internal standard and the NIST 610 standard as an external standard. The ages were calculated using ISOPLOT/Excel version 4.15 (Ludwig, Reference Ludwig2003). The common Pb correction was carried out using the Excel program ComPbCorr#3 (Andersen, Reference Andersen2002). Age uncertainties are quoted at the 95 % confidence level.
Zircon Lu–Hf isotopic compositions were obtained by laser ablation – multicollector – ICP-MS (LA-MC-ICP-MS) employing a Nu Plasma HR instrument coupled to a GeoLas 2005 193 nm ArF excimer LA system. This analysis used an applied energy density of 15–20 J cm−2, a laser pulse repetition rate of 8 Hz, and a beam diameter of 44 μm. High-purity helium gas was used as carrier gas to transport ablated particles from the sample chamber to the MC-ICP-MS instrument. Masses of 172Yb, 173Yb, 175Lu, 176(Hf + Yb + Lu), 177Hf, 178Hf, 179Hf and 180Hf were collected simultaneously by Faraday cups with the isobaric interference of 176Lu on 176Hf corrected by measuring the intensity of the interference-free 175Lu isotope and using the recommended 176Lu/175Lu ratio of 0.02669 to calculate 176Lu/177Hf values. The interference of 176Yb on 176Hf was similarly corrected by measuring an interference-free 172Yb isotope and using a 176Yb/172Yb ratio of 0.5886 to calculate corrected 176Hf/177Hf ratios (Chu et al. Reference Chu, Taylor, Chavagnac, Nesbitt, Boella, Milton, German, Bayon and Burton2002). Measured 176Hf/177Hf ratios were normalized to 179Hf/177Hf = 0.7325. Data quality was assessed by the alternating analysis of 91500 and GJ-1 standard zircon grains as unknowns yielding 176Hf/177Hf ratios of 0.282304 ± 0.000026 (n = 14, 2σ) for 91500 and 0.282013 ± 0.000016 (n = 16, 2σ) for GJ-1, both of which are consistent and within the uncertainties of the recommended 176Hf/177Hf ratios for these standards (Wu et al. Reference Wu, Yang, Xie, Yang and Xu2006). The resulting data were reduced using a 176Lu decay constant of 1.867 × 10−11 yr−1 (Albarède et al. Reference Albarède, Scherer, Blichert-Toft, Rosing, Simionovici and Bizzarro2006). Present-day 176Hf/177Hf = 0.282785 and 176Lu/177Hf = 0.0336 chondritic values (Bouvier et al. Reference Bouvier, Vervoort and Patchett2008) were used to calculate ϵ Hf(t) values with single-stage Hf model ages (T DM) calculated relative to the depleted mantle using present-day 176Hf/177Hf and 176Lu/177Hf values of 0.28325 and 0.0384, respectively (Griffin et al. Reference Griffin, Pearson, Belousova, Jackson, Van Achterbergh, O’Reilly and Shee2000). Two-stage model ages (T C DM) were calculated by projecting initial zircon 176Hf/177Hf ratios back to the depleted mantle growth curve using a value of 176Lu/177Hf = 0.015 for the average continental crust (Griffin et al. Reference Griffin, Wang, Jackson, Pearson and O’Reilly2002).
Whole-rock geochemical analysis used fresh whole-rock samples that were trimmed to remove weathered surfaces, cleaned with deionized water, crushed, and finally milled using a tungsten carbide ball mill to pass a ∼200 mesh. Major and trace element concentrations were determined by X-ray fluorescence spectrometry (XRF) employing a Rikagu RIX 2100 instrument and ICP-MS employing an Agilent 7500a instrument, respectively. Analyses of United States Geological Survey BHVO-1, AGV-1 and BCR-2 standards yielded analytical precision and accuracy values that are better than 5 % and 10 % for major and trace elements, respectively (Y Liu et al. Reference Liu, Gao, Diwu, Yuan and Hu2007).
5. Results
5.a. Zircon LA-ICP-MS U–Pb dating
A total of 27 zircon grains from gabbro sample DLH-1 were analysed, 13 of which were concordant (90–110 %) and are reported in Table 2. The zircon grains are prismatic with aspect ratios of ∼2:1 (Fig. 3). The majority have slight to dark CL luminescence along with internal textures dominated by grown zoning and high Th/U ratios (0.44–2.7) (Table 2; Fig. 3), all of which are indicative of a magmatic origin (Rubatto, Reference Rubatto2002; Corfu et al. Reference Corfu, Hanchar, Hoskin and Kinny2003). Nine analyses with concordant 206Pb/238U ages of 757 to 720 Ma yield a weighted average 206Pb/238U age of 738 ± 11 Ma (MSWD = 2.6, n = 9) that represents the timing of crystallization of the gabbros. A further four captured zircon grains yield older ages of 2397 to 1295 Ma (Table 2; Fig. 3).
Table 2. LA-ICP-MS zircon U–Pb dating results for zircon grains from the Xialanuoer gabbros


Fig. 3. Representative CL images and U–Pb concordia diagram of zircon grains from the Xialanuoer gabbros. The small yellow and large blue circles are locations for U–Pb dating and Hf-isotope analyses, respectively. Error ellipses represent 1σ uncertainties.
5.b. Whole-rock major and trace element compositions
The major and trace element concentrations of the six representative gabbro samples analysed in this study (DLH-2 to 7) are given in Table 3. These samples have slightly high loss-on-ignition (LOI) values (0.86–1.13 wt %), so major element concentrations were recalculated to 100 % volatile-free totals. The Xialanuoer gabbros contain 45.50–50.18 wt % SiO2 and heterogeneous Al2O3 (14.46–16.85 wt %), Fe2O3t (11.14–15.83 wt %), MgO (7.37–10.47 wt %), CaO (7.71–9.09 wt %), TiO2 (1.68–2.00 wt %), Na2O (2.43–4.12 wt %) and K2O (0.75–0.93 wt %) contents. They are metaluminous with A/CNK ratios of 0.71–0.75. They also have a rather restricted range of Mg# values (56–58). Owing to the potential mobility of large-ion lithophile elements (LILEs; e.g. K, Na, Rb, Sr, Ba and Cs), the samples were classified with respect to immobile elements such as high-field-strength elements (HFSEs) and rare-earth elements (REEs). The majority of the gabbros are classified as subalkaline basalt (barring two samples classified as andesite or basalt) in a Nb/Y vs Zr/TiO2 classification diagram, consistent with the compositions of contemporaneous mafic rocks in the SQB (Fig. 4a). The Xialanuoer gabbros and contemporaneous mafic rocks are both classified as calc-alkaline and basaltic in a Ta/Yb vs Ce/Yb diagram (Fig. 4b).
Table 3. Major- (wt %) and trace-element (ppm) compositions of the Xialanuoer gabbros

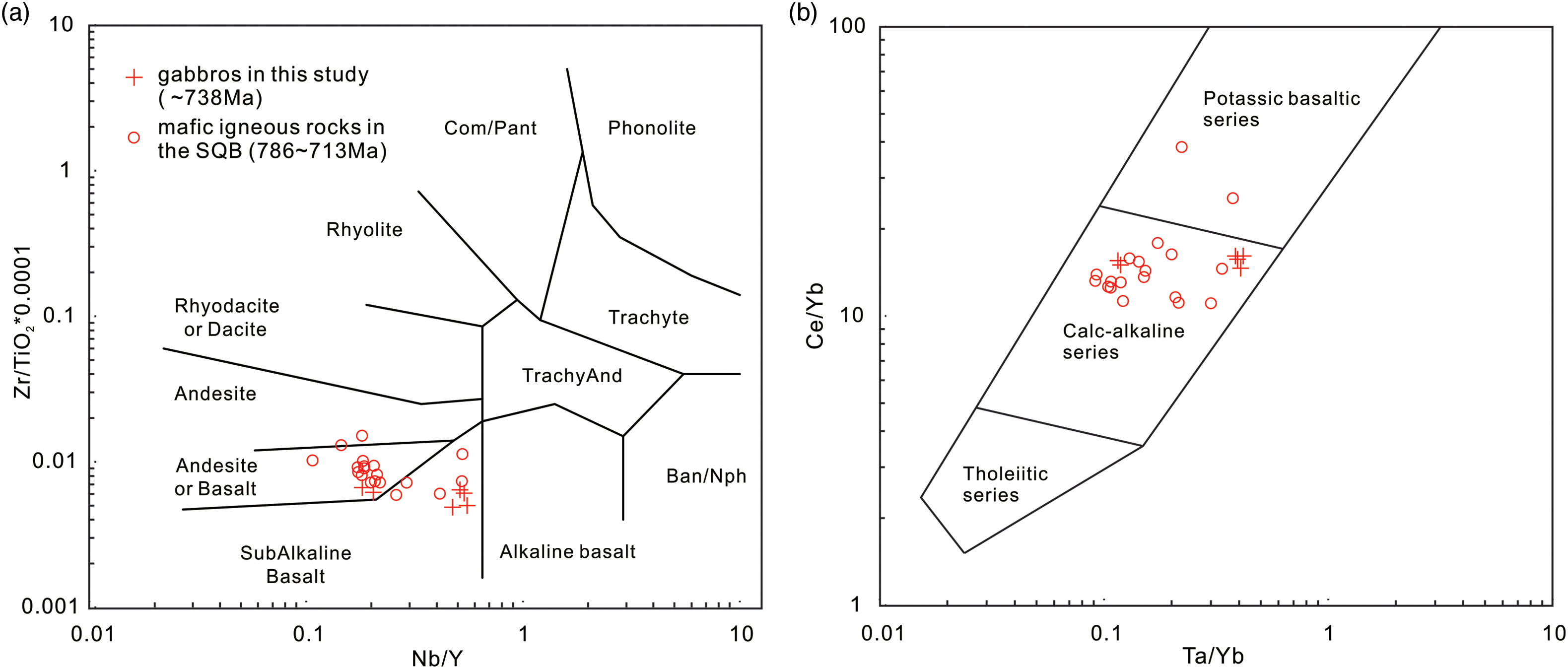
Fig. 4. Rock classification diagrams for the mafic rock samples. (a) Nb/Y vs Zr/TiO2 diagram (Winchester & Floyd, Reference Winchester and Floyd1977). (b) Ta/Yb vs Ce/Yb diagram (Pearce, Reference Pearce and Thorpe1982). Mid-Neoproterozoic mafic igneous rocks from the SQB (Ma et al. Reference Ma, Jia, Li, Ma, Lei, Ren and Xu2017; Bai et al. Reference Bai, Zhuan, Mao, Chen and Li2019; Wang et al. Reference Wang, Li, Hu, Yang, Guo, Yan, Ge and Ji2019; Ji et al. Reference Ji, Li, Huang, Wang and Wang2020).
The Xialanuoer gabbros have relatively low total REE contents (ΣREE = 61.94–111.19 ppm), have chondrite-normalized REE patterns that are enriched in light REEs (LREEs; 51.34–93.13 ppm) relative to heavy REEs (LREE/HREE = 4.49–5.16, LaN/YbN = 4.56–5.27) and have no significant Eu anomalies (Eu/Eu* = 0.97–1.03) (Fig. 5a). The Xialanuoer gabbros are also slightly depleted in REEs relative to contemporaneous mafic rocks in the SQB. They also have primitive-mantle-normalized multi-element variation diagram patterns that are enriched in LILEs (i.e. Rb, Ba and K) and depleted in HFSEs (i.e. Nb and Ta) (Fig. 5b).

Fig. 5. (a) Chondrite-normalized REE patterns. (b) Primitive-mantle-normalized incompatible-element abundances. Chondrite and primitive-mantle values are from Sun & McDonough (Reference Sun and McDonough1989).
5.c. Zircon Hf isotope compositions
The results of zircon Hf isotope analysis are given in Table 4 and shown in Figure 6. The 757–720 Ma zircon grains from sample DLH-1 have very negative ϵ Hf(t) values (−13.8 to −6.2), and old Hf model ages (T DM = 1854–1574 Ma; T C DM = 2498–2046 Ma). The 2078–1295 Ma zircon grains have restricted ϵ Hf(t) values (−14.7 to −0.7), with older Hf model ages (T DM = 2557–2362 Ma; T C DM = 2985–2620 Ma). However, a 2397 Ma zircon yields a relatively positive ϵ Hf(t) value of +3.8 and ancient Hf model ages (T DM = 2578 Ma; T C DM = 2690 Ma).
Table 4. Hf-isotope compositions of zircon grains from the Xialanuoer gabbros


Fig. 6. Zircon Hf-isotope compositions of the Xialanuoer gabbros.
6. Discussion
6.a. Age of the Xialanuoer gabbros and contemporaneous regional magmatism
Due to intense Caledonian tectonism, a large volume of Neoproterozoic igneous rocks that were originally present in this area have been tectonically disrupted and can hardly be recognized. A few Neoproterozoic igneous rocks crop out in the western and eastern SQB, including small volumes of 786–713 Ma basalts (Bai et al. Reference Bai, Zhuan, Mao, Chen and Li2019; Wang et al. Reference Wang, Li, Hu, Yang, Guo, Yan, Ge and Ji2019; Ji et al. Reference Ji, Li, Huang, Wang and Wang2020) and minor ca. 730 Ma gabbros (Ma et al. Reference Ma, Jia, Li, Ma, Lei, Ren and Xu2017). The Xialanuoer gabbroic pluton is located in the central SQB and preserved in the Balonggonggaer Formation. Previous research has suggested that the pluton was formed during the early Palaeozoic (BGMRQH, 1997), but our new zircon U–Pb dating indicates that the gabbros were actually emplaced at 738 ± 11 Ma. This is contemporaneous with 824–675 Ma magmatism in the North Qilian, Central Qilian, Quanji, North Qaidam and East Kunlun regions of the northeastern Tibetan Plateau, as summarized in Table 5. Although the majority of this mafic magmatism occurred at 796–713 Ma, basalts in the Central Qilian Block suggest that this Mid-Neoproterozoic mafic magmatism began at ca. 824 Ma (Xu et al. Reference Xu, Wang, Chen, He, Wu and Gao2008).
Table 5. Summary of published zircon U–Pb ages for the ca. 824–675 Ma magmatic rocks in the northeastern Tibetan Plateau

* SHRIMP: sensitive high-resolution ion microprobe; TIMS: thermal ionization mass spectrometry.
6.b. Petrogenesis
The Xialanuoer gabbros have variable but low Ni (108–159 ppm) and Cr (56.5–99 ppm) contents and lower Mg# values (56–58) (Table 3) than those expected for primary basaltic magmas (Ni > 400 ppm, Cr > 1000 ppm and Mg# > 73) (Wilson, Reference Wilson1989), suggesting the magmas that formed these gabbros underwent fractional crystallization prior to their emplacement. Moreover, no significant Eu anomalies (Eu/Eu* = 0.97–1.03) are observed, reflecting that the gabbros underwent insignificant fractionation and/or accumulation of plagioclase.
The negative zircon ϵ Hf(t) values of the ca. 738 Ma gabbros (Fig. 6) reflect formation from either melts derived from asthenospheric mantle that underwent crustal contamination prior to emplacement or from an enriched region of the lithospheric mantle. Crustal contamination can significantly affect the geochemical compositions of mafic magmas, causing enrichments in LILEs, Zr and Hf, and depletions in Nb and Ta (Jenner et al. Reference Jenner, Foley, Jackson, Green, Fryer and Longerich1993). The Xialanuoer gabbros have negative Nb–Ta anomalies but no positive Zr–Hf anomalies (Fig. 5b). Titanium is generally immobile during a variety of geological processes, with negative Ti anomalies often used as evidence of crustal contamination (Bienvenu et al. Reference Bienvenu, Bougault, Joron, Treuil and Dmitriev1990; Rudnick & Gao, Reference Rudnick and Gao2003). The studied samples have no negative Ti anomalies (Fig. 5b), suggesting that these gabbros record minimal crustal contamination. In addition, their Lu/Yb ratios (0.14–0.15) are lower than those expected for continental crust (0.16–0.18) (Rudnick & Gao, Reference Rudnick and Gao2003). Their Nb/Ta ratios (average of 15.85) are higher than crustal average values (12–13) (Barth et al. Reference Barth, McDonough and Rudnick2000) but closer to the values expected for the mantle (15.9 ± 0.6) (Pfänder et al. Reference Pfänder, Münker, Stracke and Mezger2007), suggesting derivation from a parental magma generated by partial melting of mantle material that underwent insignificant crustal contamination prior to emplacement.
Asthenosphere–lithosphere interaction plays a key role in the genesis of continental basalts (Turner & Hawkesworth, Reference Turner and Hawkesworth1995), and Nb/La ratios can be used to discriminate between magmas derived from the asthenospheric mantle and the sub-continental lithospheric mantle (SCLM). Asthenospheric mantle-derived melts are generally characterized by high Nb/La ratios (0.9–1.3) (Sun & McDonough, Reference Sun and McDonough1989), whereas SCLM-derived melts have lower Nb/La ratios that are similar to those expected for continental crust (Wang et al. Reference Wang, Li, Li, Pisarevsky and Wingate2014). The Xialanuoer gabbros have low Nb/La ratios (average of 0.72) that are similar to those expected for SCLM-derived melts (Wang et al. Reference Wang, Li, Li, Pisarevsky and Wingate2014), and their low Th/Nb and Th/La ratios (averages of 0.12 and 0.08, respectively) are consistent with derivation from enriched mantle of type-I (EMI-type) mantle (Saunders et al. Reference Saunders, Rogers, Marriner, Terrell and Verma1987; Weaver, Reference Weaver1991; Ernst & Buchan, Reference Ernst and Buchan2003). Combining the very negative zircon ϵ Hf(t) values (−13.8 to −6.2) with the trace element geochemical characteristics of the Xialanuoer gabbros suggests that they probably formed from melts derived from an EMI-type enriched region of the lithospheric mantle.
6.c. Tectonic setting
The tectonic setting of the Mid-Neoproterozoic magmatic activity in the SQB remains controversial, with some studies suggesting this magmatism occurred in a continental rift setting (Bai et al. Reference Bai, Zhuan, Mao, Chen and Li2019; Ji et al. Reference Ji, Li, Huang, Wang and Wang2020) and others inferring that it occurred in an active continental arc setting (Ma et al. Reference Ma, Jia, Li, Ma, Lei, Ren and Xu2017; Wang et al. Reference Wang, Li, Hu, Yang, Guo, Yan, Ge and Ji2019).
Arc-type and within-plate basalts can be distinguished using Zr/Sm and Ti/V ratios (Zhou et al. Reference Zhou, Li, Ge and Li2007). In general, within-plate basalts have high Zr/Sm (>25) and Ti/V ratios (>20). The majority of the Xialanuoer gabbros and contemporaneous mafic rocks in the SQB have high Zr/Sm (25–38) and Ti/V (24–56) ratios (Ma et al. Reference Ma, Jia, Li, Ma, Lei, Ren and Xu2017; Bai et al. Reference Bai, Zhuan, Mao, Chen and Li2019; Wang et al. Reference Wang, Li, Hu, Yang, Guo, Yan, Ge and Ji2019; Ji et al. Reference Ji, Li, Huang, Wang and Wang2020) that are consistent with the values expected for within-plate basalts. Continental rift basalts have Th/Ta and Ta/Hf values of 1.6–4.0 and 0.1–0.3, respectively (Wang et al. Reference Wang, Zhang and Xiu2001). The Xialanuoer gabbros and contemporaneous mafic rocks have Th/Ta and Ta/Hf ratios of 1.66–to 3.65 and 0.09–0.28, respectively (Ma et al. Reference Ma, Jia, Li, Ma, Lei, Ren and Xu2017; Bai et al. Reference Bai, Zhuan, Mao, Chen and Li2019; Wang et al. Reference Wang, Li, Hu, Yang, Guo, Yan, Ge and Ji2019; Ji et al. Reference Ji, Li, Huang, Wang and Wang2020), indicative of formation in a continental rift setting. Plotting the Xialanuoer gabbros and mafic rocks on Zr vs Zr/Y, Zr vs TiO2, Ti/100 – Zr – Y * 3, and Nb * 2 – Zr/4 – Y diagrams yields similar results (Fig. 7a–d).

Fig. 7. Mid-Neoproterozoic gabbros in this study and 796–675 Ma mafic igneous rocks from the South Qilian (Ma et al. Reference Ma, Jia, Li, Ma, Lei, Ren and Xu2017; Bai et al. Reference Bai, Zhuan, Mao, Chen and Li2019; Wang et al. Reference Wang, Li, Hu, Yang, Guo, Yan, Ge and Ji2019; Ji et al. Reference Ji, Li, Huang, Wang and Wang2020), North Qilian (Mao et al. Reference Mao, Zhang, Yang, Song, Wu and Zuo1997; Song et al. Reference Song, Liu, Li, Zhang, Liang and Zhen2016), Central Qilian (Xu et al. Reference Xu, Wang, Chen, He, Wu and Gao2008), North Qaidam (Chen et al. Reference Chen, Liu, Sun and Liou2009) and East Kunlun regions (Ren et al. Reference Ren, Liu, Zhou, Feng, Zhang, Dong and Qin2010, Reference Ren, Zhang, Liu, Zhou and Feng2011), plotted in the (a) Zr vs Zr/Y, (b) Zr vs TiO2 (Pearce & Norry, Reference Pearce and Norry1979), (c) Ti/100 − Zr − Y * 3 (Pearce & Cann, Reference Pearce and Cann1973) and (d) Nb * 2 − Zr/4 − Y tectonic discrimination diagrams (Meschede, Reference Meschede1986).
The 796–675 Ma mafic rocks in the northeastern Tibetan Plateau formed in a within-plate setting (Fig. 7a–d), again consistent with a continental rift setting (Xu et al. Reference Xu, Wang, Chen, He, Wu and Gao2008; Chen et al. Reference Chen, Liu, Sun and Liou2009; Ren et al. Reference Ren, Liu, Zhou, Feng, Zhang, Dong and Qin2010, Reference Ren, Zhang, Liu, Zhou and Feng2011; Song et al. Reference Song, Liu, Li, Zhang, Liang and Zhen2016; Xia et al. Reference Xia, Li, Yu and Wang2016; Ma et al. Reference Ma, Jia, Li, Ma, Lei, Ren and Xu2017; Bai et al. Reference Bai, Zhuan, Mao, Chen and Li2019; Ji et al. Reference Ji, Li, Huang, Wang and Wang2020). The Mid-Neoproterozoic magmatism in the SQB therefore represents an extension of the magmatism identified in the Quanji and Central Qilian blocks. This ∼600 km long igneous belt extends from the northwestern and central parts of the SQB to the southeastern SQB, through the western Danghenanshan and on to eastern Tianjun (Fig. 1b).
6.d. Tectonic implication
The Xialanuoer gabbros provide further constraints on the composition of Precambrian basement rocks in the SQB and the crustal evolution of this region. The captured ca. 2397 and 2078–1295 Ma zircon grains in the gabbro have one positive ϵ Hf(t) (+3.8) and multiple negative (−14.7 to −0.7) ϵ Hf(t) values, respectively (Table 4; Fig. 6), indicating the probable existence of Early Precambrian basement rocks in the SQB and providing evidence of Palaeoproterozoic crustal growth and Mesoproterozoic reworking.
Previous studies have indicated that the Central Qilian Block and surrounding terranes were involved in the Neoproterozoic amalgamation and break-up of Rodinia (Song et al. Reference Song, Niu, Su and Xia2013; Tung et al. Reference Tung, Yang, Liu, Zhang, Yang, Shau and Tseng2013; Xu et al. Reference Xu, Song, Su, Li, Niu and Allen2015; Yan et al. Reference Yan, Aitchison, Fu, Guo, Niu, Xia and Li2015; Wang et al. Reference Wang, Xu, Santosh, Xu, Deng and Fu2017; Zhang et al. Reference Zhang, Song, Yang, Su, Niu, Allen and Xu2017; S Li et al. Reference Li, Sun, Zhao, Sun, Yang, Tian, Li and Yang2018; Qin et al. Reference Qin, Feng, Chen, Chen, Zou, Liu, Jiao, Zhou, Pan and Gao2018), an event associated with magmatism (Table 5). These continental rift-related magmatic rocks are widespread in the Qilian – Qaidam – East Kunlun region of the northeastern Tibetan Plateau and record the lithospheric extension and thinning. The Duoruonuoer Group in the Central Qilian Block, the schists of the Balonggonggaer Formation in the SQB, and the Heitupo-Hongtiegou-Zhoujieshan Formations of the Quanji Group in the Quanji Block provide the sedimentary record of Mid-Neoproterozoic continental rifting within the northeastern Tibetan Plateau (Li et al. Reference Li, Lu, Wang, Xiang and Zhen2003; Ji et al. Reference Ji, Yu, Li, Huang and Wang2018; Qin, Reference Qin2018; Li et al. Reference Li, Wang, Li, Meert, Peng and Zhang2019).
Widespread Mid-Neoproterozoic continental rift-type sedimentation and anorogenic magmatism is also recorded in other Rodinia blocks, including the Tarim (Xu et al. Reference Xu, He, Zhang, Zhang, Wang and Cai2013; Zhang et al. Reference Zhang, Zou, Li and Wang2013), South China (Ling et al. Reference Ling, Gao, Zhang, Li, Liu and Cheng2003; Wang et al. Reference Wang, Li, Li, Li and Zhang2011; Wan et al. Reference Wan, Zeng, Asimow, Zeng, Peng, Xu, Wei, Liu, Lu and Chang2019), Australia (Powell et al. Reference Powell, Preiss, Gatehouse, Krapez and Li1994; Wingate et al. Reference Wingate, Campbell, Compston and Gibson1998; Preiss, Reference Preiss2000), North America (Park et al. Reference Park, Buchan and Harlan1995; McClellan & Gazel, Reference McClellan and Gazel2014) and Southern Africa blocks (Frimmel et al. Reference Frimmel, Zartman and Späth2001). These major global rifting events triggered Rodinian disintegration.
7. Conclusions
-
(1) The Xialanuoer gabbros in the SQB formed at ca. 738 Ma, and was contemporaneous with the widespread mafic magmatism in the northeastern Tibetan Plateau.
-
(2) The Xialanuoer gabbros underwent little to no crustal contamination and show geochemical characteristics of within-plate basalts. Their parental magma was probably sourced from an EMI-type enriched region of the lithospheric mantle. They formed in a continental rift setting, rather than an arc-related setting.
-
(3) In the SQB, crustal growth and reworking likely occurred in the Palaeoproterozoic and Mesoproterozoic.
-
(4) The Central Qilian, Quanji, Qaidam and East Kunlun blocks that make up the present northeastern Tibetan Plateau played a significant role during the break-up of Rodinia.
Notes
Acknowledgements
We are very grateful to editor-in-chief Prof. Peter Clift, Prof. Fernando Corfu, Prof. Frederico Castro Jobim Vilalva and Prof. Qian Liu for their constructive suggestions and comments, which led to significant improvement of the manuscript. We would also like to thank Mr ShaoYi Ban for his assistance during the field work, and Mr Huadong Gong for laboratory assistance. This research was jointly supported by the National Science and Technology Major Project (No. 2016ZX05003006), the Natural Science Foundation of Shaanxi Province, China (No. 2021JQ-630 and 2021JQ-631), the Youth Fund of Shandong Bureau of China Metallurgical Geology Bureau (No. SDYJ-QNKY202004), the Doctoral Research Startup Project of Yan’an University (No. YDBK2019-68 and YDBK2019-69), and the Innovation and Entrepreneurship training programme project for undergraduates of Yan’an University (No. D2020121 and D2020122).
Declaration of interest
The authors declare that they have no conflicts of interest.