Introduction
Among multicellular organisms, the phylum Nematoda is one of the most abundant and diverse animal groups. Most nematodes are free-living and non-parasitic, but some have acquired parasitism against a wide variety of organisms including animals and plants. According to molecular phylogenetic analyses, there are at least three origins of plant parasitism, 10 origins of parasitism of a wide range of non-vertebrates and five origins of parasitism of vertebrate hosts (Bird et al., Reference Bird, Jones, Opperman, Kikuchi and Danchin2014; Blaxter and Koutsovoulos, Reference Blaxter and Koutsovoulos2014). It will be interesting to clarify the mechanisms by which nematodes in different groups can independently acquire parasitism to a wide variety of hosts. Insect parasitism arose from different groups of nematodes. While at least 10 origins of non-vertebrate parasitism indicated by molecular phylogenetic analyses, at least 20 events of acquisition of insect parasitism have been proposed in another study (Sudhaus, Reference Sudhaus, Makarov and Dimitrijevic2008). Despite not being well understood, the evolution of insect parasitism in nematodes may be related to the abundance of insects, which share habitats with nematodes, permitting numerous opportunities for encounters. In addition, insects with relatively simple immunity may be related. Unlike humans, insects do not have adaptive immunity, and they cope with invading pathogens mainly via innate immunity. There are two types of innate immunity in insects: cellular immunity mediated by haemocytes circulating in the haemocoel and humoral immunity mediated by antimicrobial peptides and phenoloxidase for melanization (Bulet and Stocklin, Reference Bulet and Stocklin2005; Eleftherianos and Revenis, Reference Eleftherianos and Revenis2011). For larger invading multicellular pathogens such as nematodes, cell-mediated immunity is generally effective, and the pathogens can be confined by the surrounding reaction (encapsulation) by haemocytes (Lavine and Strand, Reference Lavine and Strand2002). Although immunity to microbial infections caused by bacteria and fungi has been extensively studied and well understood (Dionne and Schneider, Reference Dionne and Schneider2008; Bier and Guichard, Reference Bier and Guichard2012; Rämet, Reference Rämet2012), that to multicellular animals including nematodes is not (Castillo et al., Reference Castillo, Reynolds and Eleftherianos2011; Cooper and Eleftherianos, Reference Cooper and Eleftherianos2016; Yi et al., Reference Yi, Wu, Lv and Li2016; Wang et al., Reference Wang, Zhuo, Tang, Liu, Wang, Wang, Yu and Wang2017; Brivio et al., Reference Brivio, Toscano, De Pasquale, De Lerma Barbaro, Giovannardi, Finzi and Mastore2018; Lalitha et al., Reference Lalitha, Karthi, Vengateswari, Karthikraja, Perumal and Shivakumar2018).
Several nematodes have been reported to overcome insect immunity. Entomopathogenic nematodes (EPNs) in the genera Steinernema and Heterorhabditis comprise a group of insect parasitic nematodes that form mutualistic associations with Xenorhabdus and Photorhabdus bacteria, respectively, in the family Enterobacteriaceae (Boemare, Reference Boemare and Gaugler2002). EPNs release symbiotic bacteria harboured in the intestine of infective juveniles (IJs) after entering the haemocoel, and the bacteria suppress insect immunity and kill the insects using metabolites and toxins (Dowds and Peters, Reference Dowds, Peters and Gaugler2002). Although the suppression of immunity is largely attributed to the symbiotic bacteria, EPNs lacking symbiotic bacteria are not encapsulated (Han and Ehlers, Reference Han and Ehlers2000; Hallem et al., Reference Hallem, Michelle, Todd and Paul2007; Walter et al., Reference Walter, Dunphy and Mandato2008). Substances secreted from EPNs are reported to have various effects on insect immunity such as killing haemocytes, destroying proteins recognizing nematodes and disrupting prophenoloxidase activation (Balasubramanian et al., Reference Balasubramanian, Hao, Toubarro, Nascimento and Simões2009, Reference Balasubramanian, Toubarro and Simões2010; Li et al., Reference Li, Cowles, Cowles, Gauglerd and Cox-Foster2009b; Toubarro et al., Reference Toubarro, Avila, Montiel and Simoes2013). It is also reported that the cuticle of EPNs lowers the encapsulation ability of haemocytes and phenoloxidase activity (Brivio et al., Reference Brivio, Pagani and Restelli2002; Yi et al., Reference Yi, Wu, Lv and Li2016). This information suggests that EPNs have acquired mechanisms to suppress and/or avoid encapsulation. In addition, mermithid nematodes, a group of insect parasitic nematodes lacking symbiotic bacteria, parasitize a wide variety of insects. Mermithid nematodes are reported to lower encapsulation ability by suppressing haemocyte activity (Li et al., Reference Li, Sun, Wang and Liu2009Reference Li, Sun, Wang and Liua). In addition, other mermithid nematodes avoid encapsulation by removing the outer skin to which haemocytes adhere (Shamseldean et al., Reference Shamseldean, Platzer and Gaugler2007). Some insect-parasitic nematodes also inhibit the encapsulation of the host, but the mechanisms by which nematodes suppress and avoid insect immunity are not well clarified.
Although in vitro and aposymbiotic rearing of some EPNs is possible, most of the parasitic nematodes are typically difficult to culture independently of their host, hindering research efforts. The bacterial-feeding, non-parasitic nematode C. elegans is a powerful model organism for research because of the ease of culturing and handling, the well-established molecular and genetic techniques, and the richness of cellular and genomic information. In the wild, C. elegans forms phoretic relationships, in which nematodes use another species to aid dispersal to new sites (Bovien, Reference Bovien1937), with several different invertebrates (Petersen et al., Reference Petersen, Hermann, Barg, Schalkowski, Dirksen, Barbosa and Schulenburg2015). Interestingly, the nematode belongs to a same clade (Clade V or Clade 9) (Bird et al., Reference Bird, Jones, Opperman, Kikuchi and Danchin2014; Blaxter and Koutsovoulos, Reference Blaxter and Koutsovoulos2014) as entomopathogenic Heterorhabditis nematodes (Blaxter et al., Reference Blaxter, De Ley, Garey, Liu, Scheldeman, Vierstraete, Vanfleteren, Mackey, Dorris, Frisse, Vida and Thomas1998) and a major group of gastrointestinal parasites of vertebrates such as Haemonchus contortus. The phylogenetically close relationships of C. elegans with both invertebrate and vertebrate parasites and its invertebrate-associated nature suggest that the nematode has some basic parasitic mechanisms, making it a potentially useful model for understanding the evolution of parasitism. Conversely, insects, which have relatively simple immune systems compared with vertebrates, may be useful for studying the host–nematode interaction. However, the immune response of insects to C. elegans has not yet been studied. The purpose of the present study is to clarify insect immune responses, especially cellular encapsulation, against C. elegans and other insect non-parasitic nematodes in the larvae of the greater wax moth Galleria mellonella. We focused on the interaction between C. elegans and G. mellonella larvae because C. elegans is a model nematode and phylogenetically close to parasitic nematodes, and G. mellonella is a popular insect host for studying both insect and mammalian pathogens including EPNs (Poinar, Reference Poinar1979; Tanada and Kaya, Reference Tanada and Kaya1993; Ramarao et al., Reference Ramarao, Nielsen-Leroux and Lereclus2012). In addition, we examined the effects of injected C. elegans on the insect host.
Materials and methods
Nematodes and insects
Caenorhabditis elegans N2 was obtained from the Caenorhabditis Genetics Center (University of Minnesota, St. Paul, MN, USA). Nematodes were maintained on nematode growth medium (NGM) plates (1.7% agar) seeded with Escherichia coli strain OP50 (Stiernagle, Reference Stiernagle and Hope1999) at 20 °C. Caenorhabditis elegans first-stage (L1) and dauer larvae were used in experiments. The dauer larval stage is equivalent to the infective stage of EPNs. L1 larvae were compared with dauer larvae. Nematodes that harboured eggs were collected from a Rich NGM plate (Stiernagle, Reference Stiernagle and Hope1999) and treated with sodium hypochlorite and NaOH (Stiernagle, Reference Stiernagle and Hope1999) to induce egg release, and the collected eggs were left overnight in an incubator at 20 °C. Hatched axenic L1 larvae were subjected to the modified Baermann method (Whitehead and Hemming, Reference Whitehead and Hemming1965) to obtain active L1 larvae. Dauer larvae of C. elegans were collected from NGM plates approximately 20 days after starting nematode culture. Nematodes were treated with 1% sodium dodecyl sulphate for 15 min, washed with distilled water 5–6 times and subjected to the modified Baermann method to eliminate non-dauer nematodes and obtain active dauer larvae.
The EPN H. bacteriophora TT01 (kindly provided by Dr Ann Burnell, National University of Ireland-Maynooth) was maintained using G. mellonella larvae. Axenic IJs of H. bacteriophora were obtained as previously described (Han and Ehlers, Reference Han and Ehlers2000). Briefly, Axenic H. bacteriophora L1 larvae hatched from surface-sterilized eggs were monoxenically cultured on lipid agar plate seeded with Photorhabdus temperata, and IJs obtained by the culture were surface-sterilized to obtain axenic IJs.
The plant-parasitic nematode Meloidogyne incognita and the fungivorous nematode Aphelenchus avenae were used as a comparison. Meloidogyne incognita was maintained with Solanum lycopersicum (Pritz: Kaneko Seeds Co., Ltd., Gunma, Japan) grown in a growth chamber at 27 °C (a 16 h light/8 h dark photoperiod) (Yoshiga and Umezaki, Reference Yoshiga and Umezaki2016). Freshly hatched second-stage juveniles were used for the experiments. Aphelenchus avenae was cultured on Botrytis cinerea growing on autoclaved barley grain medium at 20 °C. Mixed-stage nematodes after extraction using the modified Baermann method were used for experiments.
Galleria mellonella was cultured on an artificial diet [240 g of honey, 200 g of glycerol, 100 mL of water, 320 g of wheat bran, 100 g of dry yeast (Ebios, Asahi Food & Healthcare, Ltd) and 40 g of bee wax (White Pellet, Wako, Osaka, Japan)]. Bee wax and honey were mixed and heated in a microwave before being mixed with the other ingredients. Insects were maintained at 30 °C, and the last instar larvae were used for the experiments.
Encapsulation assay
An aliquot of 50 µL of nematode suspension containing approximately 100 L1 or dauer larvae of C. elegans in sterile M9 buffer (3 g of KH2PO4, 6 g of Na2HPO4, 5 g of NaCl, 1 mL of 1 M MgSO4, H2O to 1 L) was injected into a G. mellonella larva using a 1 mL tuberculin syringe with a 26 G × 1/2″ needle (SS-01T2613S: Terumo Corporation, Tokyo, Japan). Insects were dissected under a dissecting microscope 24 and 72 h after nematode injection, the insect carcass was carefully washed with M9 buffer to recover injected nematodes, and nematodes were counted under a stereoscopic microscope as unencapsulated, partially encapsulated or completely encapsulated. For comparison, nematodes treated via autoclaving (120 °C, 1.2 atm, 20 min) or exposure to chloroform (5 min soak following by extensive washing with distilled water) or 1 pp Abamectin (Abamectin standard, Wako Pure Chemical Industries, Ltd., Osaka, Japan), a nematicide that inhibits a glutamate-gated ion channel in the invertebrate neural system resulting in nematode inactivation, were also injected. Abamectin-treated C. elegans were prepared by soaking nematodes in a final concentration of 1 ppm abamectin followed by several washes with M9 buffer. As a comparison, approximately 100 individuals of axenic H. bacteriophora IJs, second-stage M. incognita and mixed-stage A. avenae were injected. Five insects were used for each treatment, and experiments were repeated three times. Three insects were used for each treatment, and experiments were repeated three times.
Changes in the number of nematodes in insects
An aliquot of 50 µL of a nematode suspension containing approximately 100 live L1 or dauer larvae of C. elegans was injected into a G. mellonella larva. Insects were dissected 1, 3 and 5 (dauer larvae) or 7 days (L1 larvae) after the injection of nematodes. Nematodes were counted under a stereoscopic microscope. As a comparison, the number of nematodes on a 6 cm Rich NGM plate seeded with E. coli OP50 was counted after nematode inoculation. Fifteen insects and 15 Rich NGM plates were used at each time point, and the experiment was repeated three times.
Measurement of the body length and width of nematodes
After injecting 50 µL of a nematode suspension containing approximately 100 surface-sterilized C. elegans L1 larvae into a G. mellonella larva, the insect was placed in a 9 cm Petri dish without food and dissected 3 days later. The body length and width of nematodes recovered from the insect were measured using an Olympus SZX16 microscope system with a DP21 digital camera (Olympus, Tokyo, Japan). As a comparison, nematodes grown on a 9 cm Rich NGM plate seeded with E. coli OP50 were measured 3 days after the inoculation of 100 surface-sterilized L1 larvae. Fifteen nematodes were used for each measurement, and the experiment was repeated three times.
Effects of nematode injection on the insect
The survival of insects after the injection of 50 µL of M9 buffer containing no nematodes, 100 live C. elegans L1 larvae, 100 live C. elegans dauer larvae, 100 heat-killed L1 larvae or 100 heat-killed dauer larvae was measured 1, 3, 7 and 10 days after injection. Nematodes were heat-killed by soaking the nematode suspension in a water bath at 60 °C for 10 min to minimize the structural damage. The internal (intestine, fat body and other organs and tissues) and external appearances of dead insects were observed under a stereoscopic microscope. Ten insects were used for each treatment, and experiment was repeated three times. Nematodes excrete ammonia, which is highly toxic to organisms, as nitrogen waste (Lee and Atkinson, Reference Lee and Atkinson1977). We assumed that increased ammonia levels in the insect haemocoel during nematode propagation may cause insect death. Ammonia concentrations in insects after no treatment and nematode injection were compared. The ammonia concentration in insects was measured using an ammonia assay kit [EnzyChrom™ Ammonia/Ammonium Assay Kit (ENH3-100), BioAssay Systems, California, USA].
Statistical analysis
For the encapsulation rate of nematode and survival rate of insects, the percentage data were transformed to arcsin and were compared using one-way analysis of variance (ANOVA). For the propagation of nematode, mean values were compared using one-way ANOVA. The statistical analysis was performed by Tukey's test. Differences in the body sizes of nematodes were statistically analysed using Student's t-test. All tests were considered significant at P < 0.05.
Results
Neither H. bacteriophora nor C. elegans is encapsulated in insects
When injected into the G. mellonella haemocoel, intact axenic H. bacteriophora IJs were alive and unencapsulated, but most of the nematodes killed by autoclaving or chloroform treatment were severely encapsulated by the haemocytes after 24 h (Fig. 1A and B). No melanization was observed during the encapsulation. Similarly, when C. elegans L1 or dauer larvae were injected, most intact nematodes were alive and not attached to haemocytes or encapsulated, but autoclaved larvae were severely encapsulated without melanization (Fig. 1C–F).

Fig. 1. Haemocyte reaction to Heterorhabditis bacteriophora and Caenorhabditis elegans in the haemocoel of Galleria mellonella larvae. Heterorhabditis bacteriophora recovered from a G. mellonella larva 24 h after the injection of intact (A) and autoclaved (B) infective juveniles were presented together with C. elegans recovered from a G. mellonella larva 24 h after the injection of intact first-stage larvae (C), dauer larvae (D), autoclaved L1 larvae (E) and autoclaved dauer larvae (F). Note that the size differences between the treatments after the injection of L1 (C and E) or dauer larvae (D and F) are caused by the heat denaturation and growth of nematodes.
The percentages of encapsulated C. elegans exposed to various treatments before injection were compared (Fig. 2A and B). All of the autoclaved L1 and dauer larvae were entirely encapsulated within 24 h after injection. Conversely, both intact L1 and dauer larvae of C. elegans were hardly encapsulated even at 72 h after injection. Most of the abamectin-treated nematodes were not encapsulated 72 h after inoculation in both nematode stages, and active undulatory movement of C. elegans did not contribute to the avoidance of encapsulation.

Fig. 2. Percentages of nematodes encapsulated in the haemocoel of Galleria mellonella larvae. Percentages of encapsulated Caenorhabditis elegans first-stage (L1) (A) or dauer (DL) larvae (B) in a G. mellonella larva 24 and 72 h after the injection of intact, abamectin-treated or autoclaved nematodes. Percentages of encapsulated intact Meloidogyne incognita and Aphelenchus avenae in a G. mellonella larva 24 and 48 h after nematode injection (C).
Similar to C. elegans, low percentages of other insect–non-parasitic nematodes such as the plant-parasitic M. incognita and fungal-feeding A. avenae were encapsulated (Fig. 2C).
Caenorhabditis elegans can propagate in the live insect haemocoel
After injecting C. elegans L1 or dauer larvae, nematodes grew in the insect haemocoel, and adults and hatched L1 nematodes were observed on day 3. The number of nematode increased by nearly 200-fold (2000 nematodes/insect after the inoculation of 100 nematodes) 5 and 7 days after the inoculation of dauer and L1 larvae, respectively (Fig. 3A, B and D). In comparison, when nematodes were cultured on a plate seeded with E. coli, the number of nematodes had expanded by 1200-fold (12 000 nematodes/plate) 7 days after L1 inoculation (Fig. 3C). The nematodes that grew in the insect haemocoel were slightly smaller than those grown on E. coli plates (Fig. 3E and F), but they harbour eggs and appear to have sufficient size to produce offspring. Other insect–non-parasitic nematodes such as M. incognita and A. avenae did not grow in the insect (data not shown).

Fig. 3. Propagation and body sizes of Caenorhabditis elegans after the injection of nematodes into Galleria mellonella larvae. Number of C. elegans nematodes in a G. mellonella larva 1, 3 and 7 days after the injection of first-stage larvae (L1) (A) and 1, 3 and 5 days after the injection of dauer larvae (DL) (B). Number of nematodes on a plate seeded with E. coli OP50 1, 3 and 7 days after the inoculation of C. elegans L1 larvae (C). The same letter indicates no statistical difference (Tukey's test, P < 0.05). Nematodes released from a ventrally dissected G. mellonella larva 10 days after the injection of C. elegans L1 larvae (D). Comparison of the body length (E) and width (F) of C. elegans adults cultured with E. coli OP50 and G. mellonella larvae. An asterisk indicates a significant difference (Student's t-test, P < 0.05).
The insect injected with C. elegans dies
Insects started to die 7 and 3 days after the injection of live C. elegans L1 and dauer larvae, respectively, and insect survival gradually decreased thereafter (Fig. 4A). Black discoloured spots were often observed in the epidermis of the dead insects (Fig. 4Bb), but inner structures such as the fat body and intestine of the dead insects were not apparently damaged (Fig. 4Bc). No mortality was observed in insects injected with heat-killed nematodes, buffer without nematodes or the non-treated control at 10 days post-injection.
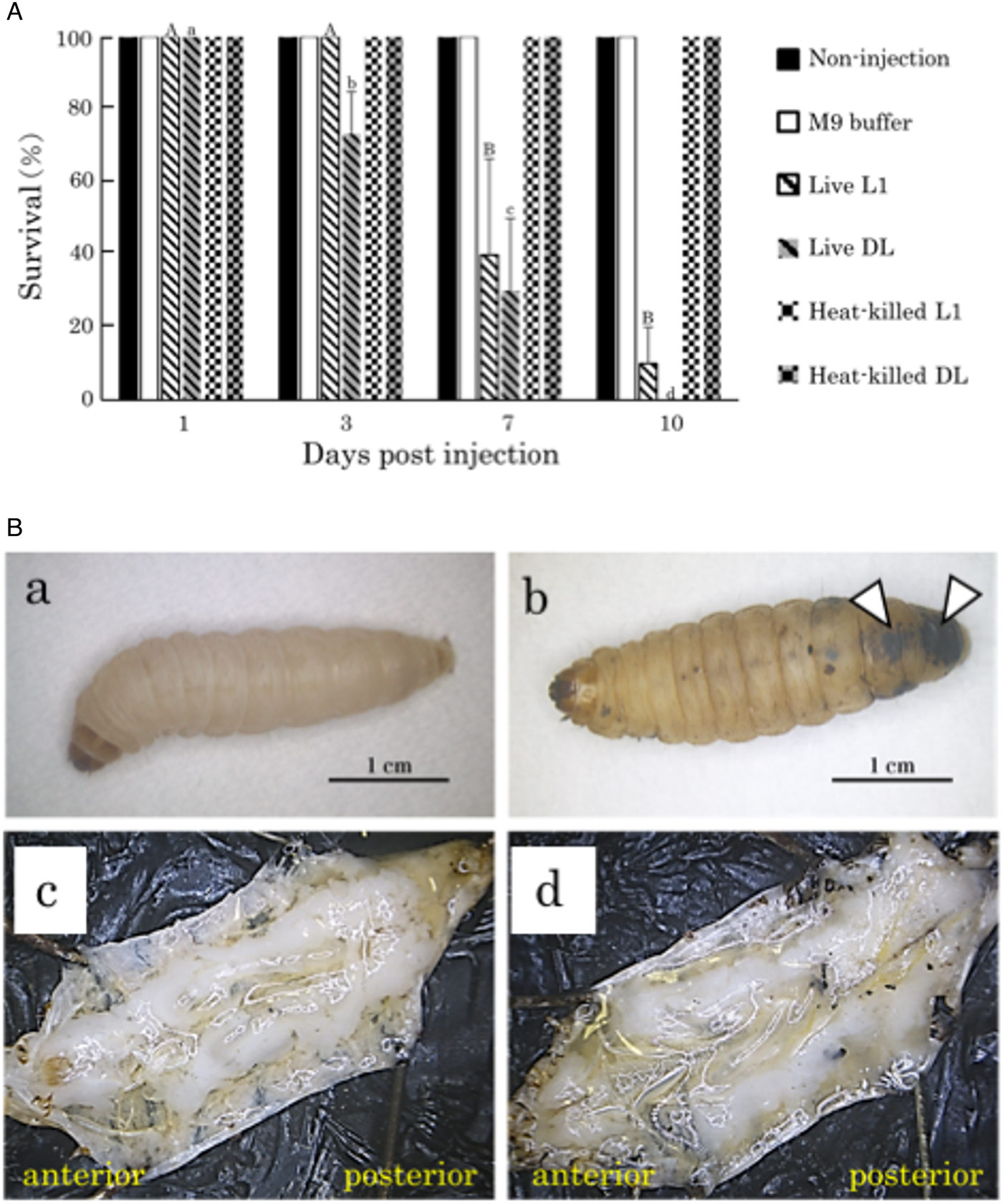
Fig. 4. Effects of Caenorhabditis elegans propagation on Galleria mellonella larvae. (A) Survival rate of G. mellonella larvae 1, 3, 7 and 10 days after the injection of 100 live or heat-killed C. elegans first-stage (L1) or dauer (DL) larvae. The survival rate of insects was calculated based upon the numbers of insects. The capital and lowercase letters indicate the statistical differences of insects injected with live L1 and dauer larvae, respectively. The same letter indicates no statistical difference (Tukey's test, P < 0.05). (B) External and internal appearances of dead G. mellonella larvae 8 days after the injection of live C. elegans L1 larvae. Galleria mellonella larvae injected with nematodes (b, d) displayed black discoloured spots (arrowheads), but no apparent damage on the fat body and other tissues was observed in comparison to non-treated controls (a, c).
We measured ammonia concentrations in the insect haemolymph after nematode inoculation, but no differences were observed (untreated insect, 475 µ m ± 322; nematode-injected insects, 461 µ m ± 502).
Discussion
Evasion of insect immunity
In the present study, we examined the cellular immune response of G. mellonella against the non-parasitic bacterial-feeding nematode C. elegans and the parasitic nematode H. bacteriophora. It was surprising that not only the EPN but also C. elegans were alive and rarely encapsulated by the insect haemocytes in the G. mellonella haemocoel when intact nematodes were injected. In addition, other insect non-parasitic nematodes such as the plant-parasitic nematode M. incognita and fungivorous nematode A. avenae were also not encapsulated in the insect haemocoel. The fact that heat-denatured nematodes were strongly encapsulated indicates that G. mellonella has sufficient ability to recognize and encapsulate foreign substances. These results suggest both parasitic and non-parasitic intact nematodes are not easily encapsulated and killed in G. mellonella.
Recognition of nematodes by the insect immune system is not well understood (Yu and Kanost, Reference Yu and Kanost2004; Sanda et al., Reference Sanda, Muhammad, Ali and Hou2018), but parasitic nematodes reportedly induce lower immune gene transcription in insects, including axenic Heterorhabditis nematodes (worms lacking symbiotic Photorhabdus bacteria) in Manduca sexta (Eleftherianos et al., Reference Eleftherianos, Joyce, ffrench-Constant, Clarke and Reynolds2010; Castillo et al., Reference Castillo, Reynolds and Eleftherianos2011) and Drosophila (Hallem et al., Reference Hallem, Michelle, Todd and Paul2007) and filarial parasites in Aedes aegypti mosquitoes (Erickson et al., Reference Erickson, Xi, Mayhew, Ramirez, Aliota, Christensen and Dimopoulos2009). Contrarily, the immune genes of Armigeres subalbatus mosquitoes are upregulated upon infection by Brugia filariae (Aliota et al., Reference Aliota, Fuchs, Mayhew, Chen and Christensen2007, Reference Aliota, Fuchs, Rocheleau, Clark, Hillyer, Chen and Christensen2010; Mayhew et al., Reference Mayhew, Bartholomay, Kou, Rocheleau, Fuchs, Aliota, Tsao, Huang, Liu, Hsiao, Tsai, Yang, Perna, Cho, Christensen and Chen2007; Kariuki et al., Reference Kariuki, Hearne and Beerntsen2010). Further extensive transcriptomic analyses revealed that the genes induced by nematode infection are associated with stress responses, lipid homoeostasis, metabolic processes, neuronal functions and possibly recognition proteins (Castillo et al., Reference Castillo, Creasy, Kumari, Shetty, Shokal, Tallon and Eleftherianos2015; Yadav et al., Reference Yadav, Daugherty, Shetty and Eleftherianos2017). Our present results suggest that even intact non-parasitic nematodes are not well recognized by the G. mellonella immune system, nor do they activate insect cellular immune responses. There is a possibility that some different responses such as stress responses in the presence of the nematodes may occur in the G. mellonela haemocoel, but these responses may not be directly related to immune responses because the intact nematodes were neither encapsulated nor killed. Factors that are important for recognition of intact nematodes to evoke encapsulation remain unclear, but mechanisms that recognize intact and autoclaved nematodes may be different.
The importance of the body surface of EPNs for evading insect immunity has been suggested (Mastore and Brivio, Reference Mastore and Brivio2008). In our study, G. mellonella could not encapsulate intact and paralysed nematodes, but it did encapsulate autoclaved and chloroform-treated nematodes. Autoclaving and chloroform treatment appear to damage body surface of nematodes and thus the intact body surface appears to be important for recognition and encapsulation of nematodes. The fact that various lineages of nematodes have acquired parasitism to insects (Sudhaus, Reference Sudhaus, Makarov and Dimitrijevic2008, Reference Sudhaus2010; Bird et al., Reference Bird, Jones, Opperman, Kikuchi and Danchin2014; Blaxter and Koutsovoulos, Reference Blaxter and Koutsovoulos2014) implies that some basic systems in nematodes contribute to the evasion of insect immunity. Conversely, some insects are reported to encapsulate parasitic nematodes (Aliota et al., Reference Aliota, Fuchs, Mayhew, Chen and Christensen2007, Reference Aliota, Fuchs, Rocheleau, Clark, Hillyer, Chen and Christensen2010; Li et al., Reference Li, Cowles, Cowles, Gaugler and Cox-Foster2007; Mayhew et al., Reference Mayhew, Bartholomay, Kou, Rocheleau, Fuchs, Aliota, Tsao, Huang, Liu, Hsiao, Tsai, Yang, Perna, Cho, Christensen and Chen2007; Kariuki et al., Reference Kariuki, Hearne and Beerntsen2010), and the free-living nematode Panagrolaimus rigidus is encapsulated in G. mellonella (Brivio et al., Reference Brivio, Mastore and Moro2004). These reports together with our results suggest that the ability to recognize and encapsulate nematodes may differ among insect species/groups and between nematode–insect combinations. However, very limited information regarding interaction between non-insect-parasitic nematodes and insects is available. Further research on the immune responses against non-parasitic nematodes in different insects may help to elucidate the evolution of immunity and evasion of insect immunity.
Propagation in the insect haemocoel
We demonstrated that C. elegans can grow and propagate in the haemocoel of live G. mellonella larvae. Although the axenic EPN S. carpocapsae can grow in G. mellonella larvae (Han and Ehlers, Reference Han and Ehlers2000; Kikuta et al., Reference Kikuta, Kiuchi, Aoki and Nagata2008; Fuchi et al., Reference Fuchi, Ono, Kondo and Yoshiga2016), this is the first report and demonstration, to the best of our knowledge, of the propagation of axenic and non-parasitic nematodes in insects. Because no bacterial infestation was observed in the insect haemolymph in which nematodes were propagating and insect tissues were not apparently damaged during the propagation, C. elegans appears to obtain nutrients from the insect haemolymph. The insect haemolymph is rich in amino acids and sugars (mainly trehalose), and it contains proteins and lipids (Nation, Reference Nation2008) and appears to have sufficient nutritional richness to support the growth of both parasitic and bacterial-feeding nematodes. The result that adults were observed 3 days after dauer larvae inoculation is indicative of the recovery from the dauer stage (to survive unfavourable condition) to propagative stages, further suggesting that the insect haemocoel is a favourable environment for C. elegans. Because C. elegans is phylogenetically similar to insect parasitic Heterorhabditis nematodes and Caenorhabditis nematodes are often associated with invertebrates (Kiontke et al., Reference Kiontke, Félix, Ailion, Rockman, Braendle, Pénigault and Fitch2011), C. elegans can be a good model for studying the evolution of insect parasitism.
Insect mortality
In this study, we found that insects died when C. elegans larvae were injected into the haemocoel of G. mellonella larvae. Autopsy revealed black discoloured spots in the epidermis of the dead insects, whereas the inner structure of dead insects after nematode inoculation was not damaged. The black discoloured spots may be caused by phenoloxidase activity, but the relationship between black discoloured spots and insect death is currently unclear. We suspected that increased ammonia content due to nematode propagation in the insect haemolymph was a cause of insect death. However, ammonia concentrations were not elevated even after nematode propagation. EPNs kill host insects via symbiotic bacteria harboured in their intestines (Forst and Clarke, Reference Forst, Clarke and Gaugler2002), but axenic EPNs in the genus Steinernema can also kill insects even without their mutualistic bacteria (Burman, Reference Burman1982; Ehlers et al., Reference Ehlers, Wulff and Peters1997; Han and Ehlers, Reference Han and Ehlers2000; Fuchi et al., Reference Fuchi, Ono, Kondo and Yoshiga2016). The cause of pathogenicity remains unclear, but the secretion of venom proteins by S. carpocapsae was recently reported (Lu et al., Reference Lu, Macchietto, Chang, Barros, Baldwin, Mortazavi and Dillman2017). In our preliminary experiments, injection of the culture medium of axenic C. elegans did not kill G. mellonella (data not shown), suggesting that different mechanisms underlie the killing of G. mellonella by C. elegans.
Author ORCIDs
Toyoshi Yoshiga, 0000-0002-7050-2618.
Acknowledgments
The authors would like to thank the Caenorhabditis Genetics Center (CGC) for providing a nematode strain and Enago (www.enago.jp) for the English language review of the manuscript.
Financial support
This work was supported by Grant-in-Aid for Challenging Exploratory Research (T.Y., grant number 23658051) and Grant-in-Aid for Scientific Research (C) (T.Y, grant number 18K05676).
Conflict of interest
None.
Ethical standard
Not applicable.