Introduction
Immune-mediated inflammatory diseases (IMIDs) are characterized by acute or chronic inflammation resulting from immune dysregulation that can affect any organ system. They have rapidly risen to high prevalence (7–9%) as populations around the globe adopt industrialized lifestyles (El-Gabalawy et al., Reference El-Gabalawy, Guenther and Bernstein2010; Rook et al., Reference Rook, Raison and Lowry2014; Lerner et al., Reference Lerner, Jeremias and Matthias2015). Inflammatory bowel diseases (IBD) – chronic, disabling gastrointestinal IMIDs that include Crohn's disease and ulcerative colitis – conform to these epidemiological trends (Molodecky et al., Reference Molodecky, Soon, Rabi, Ghali, Ferris, Chernoff, Benchimol, Panaccione, Ghosh, Barkema and Kaplan2012; Ponder and Long, Reference Ponder and Long2013). The rapid rise in IMIDs, and IBD in particular, implicates environmental factors. Changes in the microbial environment through host development that are associated with the hygienic modern lifestyle are important risk factors (Velasquez-Manoff, Reference Velasquez-Manoff2012; Rook et al., Reference Rook, Raison and Lowry2014). Indeed, changes in the gut community are common in IBD and other IMIDs (Clemente et al., Reference Clemente, Ursell, Parfrey and Knight2012; Rook et al., Reference Rook, Raison and Lowry2014). These include detrimental shifts in the composition and diversity (dysbiosis) of bacteria (Lozupone et al., Reference Lozupone, Stombaugh, Gordon, Jansson and Knight2012; Sartor and Mazmanian, Reference Sartor and Mazmanian2012; Kostic et al., Reference Kostic, Xavier and Gevers2014) and fungi (Iliev and Leonardi, Reference Iliev and Leonardi2017), as well as declining prevalence of protists (Rossen et al., Reference Rossen, Bart, Verhaar, van Nood, Kootte, de Groot, D'Haens, Ponsioen and van Gool2015) and absence of helminths (Elliott and Weinstock, Reference Elliott and Weinstock2012). We note that while helminths and other eukaryotes associated with mammals are generally considered parasites, their impacts on the host vary across species and host condition (Lukeš et al., Reference Lukeš, Stensvold, Jirků Pomajbíková and Parfrey2015). Therefore, we refer to them as gut symbionts, a neutral term that encompasses parasites, commensals and mutualists (Leung and Poulin, Reference Leung and Poulin2008).
Reintroduction of missing diversity is a promising therapeutic avenue for the treatment of IMIDs. This idea is rooted in the observations that mammals evolved in the presence of helminths and rich microbial exposure and that absence of these exposures in industrialized populations has profound consequences (Velasquez-Manoff, Reference Velasquez-Manoff2012; Parker and Ollerton, Reference Parker and Ollerton2013; Rook et al., Reference Rook, Raison and Lowry2014). The continuous presence of helminths induced changes in the human genome, particularly in genes related to the immune system (Fumagalli et al., Reference Fumagalli, Pozzoli, Cagliani, Comi, Riva, Clerici, Bresolin and Sironi2009); indeed, many of these genetic markers are risk factors for IBD and other IMIDs, suggesting that genetic changes that are beneficial in the presence of helminths are detrimental in their absence (Fumagalli et al., Reference Fumagalli, Pozzoli, Cagliani, Comi, Riva, Clerici, Bresolin and Sironi2009; Mangano and Modiano, Reference Mangano and Modiano2014). Mammals rely upon early microbial exposures – exposures that were reliably present historically but are disrupted today – for proper development of the immune system and other critical host functions (Blaser, Reference Blaser2014; Lloyd-Price et al., Reference Lloyd-Price, Abu-Ali and Huttenhower2016). Helminths are potent regulators of the mammalian immune system, and their therapeutic potential lies primarily in their ability induce type 2 immune responses and inhibit type 17 and type 1 immune response through activation of regulatory immune mechanisms such as regulatory T cells, regulatory B cells, dendritic cells and production of anti-inflammatory cytokines (Allen and Maizels, Reference Allen and Maizels2011; Girgis et al., Reference Girgis, Gundra and Loke2013; Maizels and McSorley, Reference Maizels and McSorley2016).
Helminth inoculation can prevent and/or treat IBD in animal models and some clinical trials without adverse side-effects (reviewed in Fleming and Weinstock, Reference Fleming and Weinstock2015; Helmby, Reference Helmby2015; Wang et al., Reference Wang, Wu, Weng, Zheng, Wu and Lv2017), though positive outcomes are not universal (Fleming and Weinstock, Reference Fleming and Weinstock2015; McKay, Reference McKay2015). Further, there is a large and growing community practicing self-inoculation with helminths for a wide range of inflammatory conditions, and symptoms are reduced in the majority of cases (Cheng et al., Reference Cheng, Jaint, Thomas, Wilson and Parker2015; Liu et al., Reference Liu, Morey, Wilson and Parker2017). However, a lack of efficacy in large trials (Fleming and Weinstock, Reference Fleming and Weinstock2015; Helmby, Reference Helmby2015) and exacerbation of disease documented in some disease models and in the presence of other infectious agents are serious outstanding concerns (McKay, Reference McKay2015). Harnessing the power of helminths requires a deeper understanding of their variable impacts on the immune system, particularly in the context of their interactions with other infectious agents and the rest of the gut microbiota (McKay, Reference McKay2015), and across mammalian hosts that may vary in immune response (Ehret et al., Reference Ehret, Torelli, Klotz, Pedersen and Seeber2017).
The bacterial microbiota residing in the mammalian gut (hereafter referred to as microbiota) also exert a strong influence on the mammalian immune system and can protect against IBD (e.g. Faecalibacterium prausnitzii) or promote disease (e.g. Fusobacterium varium) (Sartor and Mazmanian, Reference Sartor and Mazmanian2012; Kostic et al., Reference Kostic, Xavier and Gevers2014). Therapies include fecal transplantation of the entire microbiota (Anderson et al., Reference Anderson, Edney and Whelan2012) and targeted introduction of species (e.g. Martín et al., Reference Martín, Chain, Miquel, Lu, Gratadoux, Sokol, Verdu, Bercik, Bermudez-Humaran and Langella2014), and antibiotics to target-specific microbiota components (Sartor and Mazmanian, Reference Sartor and Mazmanian2012). The need to understand how helminths interact with the rest of the gut microbiota to modulate host immune response and disease outcomes is particularly pressing in light of our growing appreciation for the complexity of the interactions among gut inhabitants (Clemente et al., Reference Clemente, Ursell, Parfrey and Knight2012), which can yield unexpected immunological outcomes (Zaiss et al., Reference Zaiss, Rapin, Lebon, Dubey, Mosconi, Sarter, Piersigilli, Menin, Walker, Rougemont, Paerewijck, Geldhof, McCoy, Macpherson, Croese, Giacomin, Loukas, Junt, Marsland and Harris2015; Chudnovskiy et al., Reference Chudnovskiy, Mortha, Kana, Kennard, Ramirez, Rahman, Remark, Mogno, Ng, Gnjatic, Amir, Solovyov, Greenbaum, Clemente, Faith, Belkaid, Grigg and Merad2016). In humans, helminth colonization is sometimes associated with shifts in gut microbial diversity (Lee et al., Reference Lee, Tang, Lim, Choy, Kurtz, Cox, Gundra, Cho, Bonneau, Blaser, Chua and Loke2014; Ramanan et al., Reference Ramanan, Bowcutt, Lee, Tang, Kurtz, Ding, Honda, Gause, Blaser, Bonneau, Lim, Loke and Cadwell2016) and sometimes not (Cooper et al., Reference Cooper, Walker, Reyes, Chico, Salter, Vaca and Parkhill2013). Helminth colonization often leads to shifts in the gut microbiota in rodent models (McKenney et al., Reference McKenney, Williamson, Yoder, Rawls, Bilbo and Parker2015; Reynolds et al., Reference Reynolds, Finlay and Maizels2015; Zaiss and Harris, Reference Zaiss and Harris2016), which can influence the susceptibility of hosts to disease (Reynolds et al., Reference Reynolds, Smith, Filbey, Harcus, Hewitson, Redpath, Valdez, Yebra, Finlay and Maizels2014; Zaiss et al., Reference Zaiss, Rapin, Lebon, Dubey, Mosconi, Sarter, Piersigilli, Menin, Walker, Rougemont, Paerewijck, Geldhof, McCoy, Macpherson, Croese, Giacomin, Loukas, Junt, Marsland and Harris2015; Ramanan et al., Reference Ramanan, Bowcutt, Lee, Tang, Kurtz, Ding, Honda, Gause, Blaser, Bonneau, Lim, Loke and Cadwell2016).
The tapeworm Hymenolepis diminuta is a good candidate for helminth therapy (Lukeš et al., Reference Lukeš, Kuchta, Scholz and Pomajbíková2014) as it cannot autoinfect, does not migrate outside of the intestinal lumen, does not harm the host (Roberts, Reference Roberts and Arai1980; McKay, Reference McKay2010), and it is relatively cheap and easy to produce (Smyth et al., Reference Smyth, Morton, Mathew, Karuturi, Haley, Zhang, Holzknecht, Swanson, Lin and Parker2017). Importantly, H. diminuta ameliorates inflammatory disease in many, but not all, animal models [reviewed in (McKay, Reference McKay2015)] and is effective in the majority of self-treating humans (Smyth et al., Reference Smyth, Morton, Mathew, Karuturi, Haley, Zhang, Holzknecht, Swanson, Lin and Parker2017).
In mice, H. diminuta protects against colitis induced by dinitrobenzene sulphonic acid (DNBS) by dampening the inflammatory response via increased production of interleukin IL-10 with involvement of regulatory T cells (McKay, Reference McKay2010; Melon et al., Reference Melon, Wang, Phan and McKay2010; Hernandez et al., Reference Hernandez, Leung and McKay2013), but does not protect against oxazolone-induced chemical colitis (Wang et al., Reference Wang, Fernando, Leung, Phan, Smyth and McKay2010). This protective effect was only found in mice that expel H. diminuta, not in rats, and so is presumed to depend on a strong type 2 immune response (Hunter et al., Reference Hunter, Wang, Hirota and McKay2005). Interestingly, H. diminuta colonization of mothers protects rat neonates against inflammation-induced brain dysfunction by reducing brain cytokine response, and H. diminuta colonization of the weaned pups also prevents cognitive dysfunction in adult rats (Williamson et al., Reference Williamson, McKenney, Holzknecht, BElliveau, Rawls, Poulton, Parker and Bilbo2016). Here, continuous colonization with H. diminuta causes only very minor systemic immune changes in the absence of an immune challenge (Williamson et al., Reference Williamson, McKenney, Holzknecht, BElliveau, Rawls, Poulton, Parker and Bilbo2016).
The immune response of rats to H. diminuta is similar to mice, though muted (McKay Reference McKay2010). Hymenolepis diminuta stably colonizes rats but initially induces a mild type 2 immune response and IL-10 production in the pre-patent period of larval development (the first 18–21 days post-colonization), followed by immunomodulation and a general reduction in immune cells in the patent period (when adult worms are established and reproductive) (McKay, Reference McKay2010; Parfrey et al., Reference Parfrey, Jirků, Šíma, Jalovecká, Sak, Grigore and Jirků Pomajbíková2017). The pre-patent immune response is elevated when excess helminths are administered as only 2–10 H. diminuta establish and the rest are expelled via type 2 immune response (Webb et al., Reference Webb, Hoque and Dimas2007). A major difference between rat and mouse permissiveness to H. diminuta colonization is caused by differential production of type 2-polarizing cytokines in gut epithelial cells: production is high in mice, very low in rats, and intermediate in humans (Lopes et al., Reference Lopes, Reyes, Wang, Leung and McKay2015). Hymenolepis diminuta does not establish long term in humans (Smyth et al., Reference Smyth, Morton, Mathew, Karuturi, Haley, Zhang, Holzknecht, Swanson, Lin and Parker2017), and the immune response to H. diminuta in humans may generally be intermediate to the strong response observed in mice and mild response in rats (Lopes et al., Reference Lopes, Reyes, Wang, Leung and McKay2015).
Hymenolepis diminuta alters the bacterial microbiota of rats during the patent period (McKenney et al., Reference McKenney, Williamson, Yoder, Rawls, Bilbo and Parker2015; Williamson et al., Reference Williamson, McKenney, Holzknecht, BElliveau, Rawls, Poulton, Parker and Bilbo2016; Parfrey et al., Reference Parfrey, Jirků, Šíma, Jalovecká, Sak, Grigore and Jirků Pomajbíková2017), though the changes observed differ between studies suggesting they are at least partially dependent on the microbial pool available for colonization, and the magnitude of microbiota change appears to be greatest with continuous colonization beginning in utero (McKenney et al., Reference McKenney, Williamson, Yoder, Rawls, Bilbo and Parker2015; Williamson et al., Reference Williamson, McKenney, Holzknecht, BElliveau, Rawls, Poulton, Parker and Bilbo2016).
Here we use a rat model to investigate the ability of H. diminuta to protect against colitis induced through application of the haptenizing agent DNBS directly to the colon, which induces acute inflammation that resolves after 3–4 days and resembles human Crohn's disease, a form of IBD. We test the ability of immature H. diminuta to ameliorate the effects of colitis during the pre-patent period, when H. diminuta induces a type 2 immune response and high IL-10 gene expression. We also test mature H. diminuta (patent period) against both moderate (single DNBS application) and severe (two DNBS applications) colitis, a model developed in this study to assess the effects of H. diminuta on longer-term disease. It is worth mentioning that this diseased model is an injury model, the initial inflammatory response to this chemical injury is adaptive and, thus, the helminth does not block all response. We monitor the gut bacterial microbiota over time, as well as measures of rat health and tumour necrosis factor alpha (TNFα) gene expression, a marker of systemic inflammation. We show that mature H. diminuta results in lower inflammation, faster recovery and lesser pathology from severe colitis, but has little impact on moderate colitis. Larval H. diminuta induce elevated IL-10 gene expression, but do not protect against severe colitis. The gut microbiota is disrupted during colitis does not appear to play an overt role in H. diminuta-mediated protection.
Material and methods
Animal use
Each experiment was carried out with outbred female Wistar rats from 2 to 3 litters per experiment obtained when 13 weeks old and 180–220 g from Charles River Laboratories (Envigo RMS B.V., Horst, Netherlands; the supplier Anlab s.r.o., Prague, Czech Republic). All rats were group-housed under a controlled temperature (22 °C) and photoperiod (12:12-h light–dark cycle) and were provided unlimited access to rat chow and tap water. Throughout each experiment rat health and morbidity were recorded in regular intervals. Rats in poor condition during the experiment were euthanized by cervical dislocation to minimize suffering if they showed the following signs: complete loss of appetite, extreme weight loss, extreme apathy and lack of activity and very dull coat, in accordance with the legislative regulations of the Czech government and European Union. All rats were euthanized by cervical dislocation at the end of the experiment, also in accordance with all regulations.
Culture of H. diminuta, colonization doses and animal colonizations
Hymenolepis diminuta was cultured under laboratory conditions using grain beetles (Tenebrio molitor) as the intermediate host and outbred rats as the definitive host and reservoirs for colonization. Grain beetles were fed rat feces containing H. diminuta eggs to establish the colonization. Doses of H. diminuta were prepared by dissecting the infectious stages, cysticercoids, from grain beetles under hygienic laboratory conditions. Each infectious dose was washed three times with sterile phosphate buffered saline (pH 7.4). Animals were colonized by oesophageal gavage with 10 cysticercoids. Colonization was confirmed during the patent period using a modified Sheather's flotation method (SpG 1.3) to look for eggs (Fig. 1, experiments 1A and 1B). All colonized rats started to shed eggs between 16 and 19 days post-colonization, indicating that mature and reproductive adult worms were established in the rat and marking the beginning of the patent period. We also confirmed the presence of larval H. diminuta (for experiment 1C) or adult H. diminuta (for experiments 1A and 1B) in each rat during dissection of sacrificed animals. All rats in the H. diminuta treatment group harboured between two and six H. diminuta individuals in the small intestine at the time of dissection.

Fig. 1. Designs of experiments testing the impact of Hymenolepis diminuta on colitis. Experiment 1A: effect of mature H. diminuta (patent period of colonization) on moderate colitis. N = 3 per group. Experiment 1B: effect of mature H. diminuta (patent period of colonization) on severe colitis N = 10 per group. Experiment 1C: effect of H. diminuta larval stages (pre-patent period of colonization) on severe colitis N = 10 per group.
Experimental setup and colitis induction
We conducted a series of experiments to test the effect of H. diminuta on colitis (see Fig. 1 and Fig. S1 for the experimental design). We initially tested the effect of adult H. diminuta (patent period of colonization) on a moderate model of DNBS (Sigma Aldrich, St. Louis, MO, USA) induced colitis established by Wallace et al. (Reference Wallace, Le, Carter, Appleyard and Beck1995) (Fig. 1, Experiment 1A). DNBS was rectally injected while animals were anesthetized with isoflurane (Forane® 100 mL, AbbVie s.r.o., Prague, Czech Republic) using anaesthesia equipment (Oxygen Concentrator JAY-10-1.4, Longfian Scitech Co. LTD, Baoding, China; Calibrated Vaporizer Matrx VIP 3000, Midmark, Dayton, OH, USA). Rectal injection was by a 10-cm long and 3.3 mm diameter catheter (catheter type Nelaton, Dahlhausen s.r.o., Kuřim, Czech Republic) and advanced such that the tip of catheter was approximately 8-cm proximal to the anus. We injected 0.5 mL of 50% (vol/vol) ethanol containing DNBS in concentration 58 mg mL−1. In all cases the control rats were injected with 0.5 mL of 50% (vol/vol) ethanol only. We observed a measurable, but modest, decrease in inflammation and no impact on rat health overall in accordance with previous results (Hunter et al., Reference Hunter, Wang, Hirota and McKay2005). We next established a severe model of colitis (Fig. S1) modelled after Martín et al. (Reference Martín, Chain, Miquel, Lu, Gratadoux, Sokol, Verdu, Bercik, Bermudez-Humaran and Langella2014) in order to investigate the impact of H. diminuta on colitis and gut microbiome over longer time periods. To induce severe colitis, DNBS was administered twice: a full dose of DNBS (0.5 mL of 50% ethanol containing 58 mg mL−1 DNBS), and a second half dose of DNBS (0.5 mL of 50% ethanol containing 29 mg mL−1 DNBS) 3 days later (Fig. S1). We then conducted experiments to test the effect of mature and immature H. diminuta on severe colitis (Fig. 1; experiments 1B and 1C, respectively).
At the start of each experiment rats were randomly assigned to experimental treatment cages in pairs or groups of three, taking care to change cage mates compared to the initial month long acclimatization period to minimize initial microbiota similarity within a cage. During the experimental period, rats were held in isolator cages and incoming air was filtered through HEPA filters; they were given unlimited access to autoclaved rat chow and water. Rats were acclimated to their new cages for seven days prior to H. diminuta colonization or colitis induction in the case of severe colitis optimization. Each experiment included two treatment groups with balanced numbers: control with colitis only and H. diminuta colonized plus colitis (Fig. 1; experiments 1A, N = 3 per group; 1B, N = 10 per group; 1C, N = 10 per group), or control and colitis (Fig. S1, N = 7 per group). Differential mortality led to unbalanced numbers by the end of experiments 1B and 1C (Table 1).
Table 1. Clinical response to colitis with and without Hymenolepis diminuta

a Indicates first colitis induction. Colitis induced a second time at 3 days post-colitis in severe experiments.
b Decreasing number of individuals over course of experiment indicates mortality due to sacrifice of animals in poor health condition.
Collection of blood and fecal samples
During each experiment we collected: (i) blood and spleen samples for analyses of cytokine gene expression, (ii) fecal samples for microbiological analyses and (iii) clinical data (see Fig. 1 and Fig. S1 for time intervals of collection). Spleen samples were collected during dissection of rats in experiment 1A only. During the collection of blood samples and clinical data were done under isoflurane anaesthesia as above. Blood samples were collected from ocular blood plexus, with 150–200 µL added to 0.5 mL EDTA Minicollect® tubes (Greiner Bio-one GmbH, Kremsmünster, Austria), vortexed in EDTA tubes and transferred to 750 µL RiboEx LSTM (GeneAll Biotechnology, Seoul, Korea) on ice. Similarly, 150-250 μg of spleen tissue was collected and trasferred to 750 μl of RiboEx LSTM (Gene All). Blood and spleen samples were then processed for cytokine gene expression (see section ‘Analyses of cytokine gene expression’). Fecal samples were collected at the same time by transferring fecal pellets to sterile 1.5 mL microcentrifuge tubes, or when animals had diarrhoea swabs of fecal material were collected and placed in sterile 1.5 mL microcentrifuge tubes. Fecal samples were stored at −20 until DNA extraction. In experiment 1A, we also collected spleen tissue by dissection following sacrifice, which was preserved and RNA extracted to assay cytokine expression.
Clinical activity
Colitis was quantified using clinical parameters of weight loss, stool consistency and haematochezia throughout the experiments. Clinical parameters were not collected in a blinded fashion so we restrict discussion to cases with overt differences between treatment groups. Stool consistency was evaluated semi-quantitatively using scale 1 to 5, while the grade 5 corresponds to normal consistency of feces of healthy animal and grade 1 to watery diarrhoea (4-normal consistency, but feces are softer; 3-consistency of feces corresponds to consistency of dense yoghurt, 2-consistency corresponds to more liquid yoghurt). In the case of haematochezia, we assessed visually presence or absence of blood in the rat feces (yes/no). We also qualitatively observed other clinical signs of colitis, including apathy and dull coat.
Absolute weight values are used for experiment 1A because rats were sacrificed over time, and thus not available for repeated measurements. Change in weight was assessed using percent weight loss calculated compared with the weight on the day before colitis induction for experiments 1B, 1C and S1. Percent weight change was compared between treatment groups on each day with Welch's t-tests followed by Benjamini–Hochberg correction to an α value of 0.05. Analyses conducted in R (R_Core_Team, 2013) and visualized using Statistica 12.0 software package (Dell technologies, TE, USA).
Analyses of cytokine relative gene expression
Total RNA from blood samples was extracted using HybridR Blood RNA Kit (GeneAll Biotechnology, Seoul, South Korea) and then reverse transcribed using High Capacity RNA-to-cDNA Kit (Thermo Fisher Scientific, Waltham, MA, USA). Total RNA from spleen was extracted using HybridR RNA Kit (GeneAll Biotechnology, Seoul, South Korea) then reverse transcribed as above. Real-time PCR reactions were prepared using master-mix HOT FIREPol® Probe qPCR Mix Plus (Solis Biodyne, Tartu, Estonia). Expression of cytokines were measured using Taqman gene expression assay for rats with specific primers and probes spanning exons, all ordered from Thermo Fisher Scientific: tumour necrosis factor (TNFα; amplicon length 92 bp), IL-10 (IL-10; amplicon length 70 bp), IL-4 (amplicon length 85 bp), IL-13 (amplicon length 73 bp), IL-1β (amplicon length 74 bp) and ubiquitin C (UBC; amplicon length 88 bp). A Light Cycler LC480 (Roche, Basel, Switzerland) as used for qPCR analysis and relative expressions of cytokines was normalized to UBC using the mathematical model of Pfaffl (Reference Pfaffl2001). Normalized C t values were compared between experimental and control animals on each day by Welch's t-tests followed by Benjamini–Hochberg correction to an α value of 0.05. Maximum normalization was used for graphical visualization of cytokines’ relative expressions for better illustration.
Microbial DNA extraction, amplification and analyses
Total DNA was purified using PSP® SPIN Stool DNA Plus Kit (Stratec Biomedical, Birkenfeld Germany) according to the manufacturer's protocol. The 16S ribosomal DNA was amplified using the following primers that target the V4 region of 16S rRNA in Bacteria and Archaea: barcoded 515f (5′–GTGYCAGCMGCCGCGGTAA–3′) and 806r (5′–GGACTACNVGGGTWTCTAAT–3′) (protocol modified from http://www.earthmicrobiome.org/emp-standard-protocols/16s/). Amplifications were conducted using 25 µL reactions containing Phusion flash polymerase (manufacturer) and 1 µL of genomic DNA. The PCR conditions were an initial denaturation step at 94 °C for 3 min, followed by 25 cycles of 94 °C for 45 s, 50 °C for 60 s, and 72 °C for 90 s, followed by a final extension step at 72 °C for 10 min. PCR products were visualized on a gel and quantified using Picogreen (Thermofisher) according to the manufacturer's protocol. Subsequently, 50 ng of each sample PCR product were pooled. The final pool was cleaned using the Ultraclean PCR cleanup kit (MO BIO Laboratories,Carlsbad, CA, USA) and sent for sequencing at Integrated Microbiome Resource at Dalhousie University. The pool was sequenced on the Illumina MiSeq platform with paired end 2 × 300 sequencing and a separate 13-nucleotide index read. Each experiment was processed and sequenced separately.
Sequence processing
Each dataset was separately demultiplexed in QIIME version 1.9 (Caporaso et al., Reference Caporaso, Kuczynski, Stombaugh, Bittinger, Bushman, Costello, Fierer, Pena, Goodrich, Gordon, Huttley, Kelley, Knights, Koenig, Ley, Lozupone, McDonald, Muegge, Pirrung, Reeder, Sevinsky, Tumbaugh, Walters, Widmann, Yatsunenko, Zaneveld and Knight2010b), then trimmed, clipped and quality filtered using the Fastx Toolkit (http://hannonlab.cshl.edu/fastx_toolkit) to 250 bp with a minimum quality threshold of Q19. The four datasets were then combined and processed into operational taxonomic units (OTUs) using Minimum Entropy Decomposition (MED, Eren et al., Reference Eren, Morrison, Lescault, Reveillaud, Vineis and Sogin2014) with the minimum substantive abundance (-m) parameter set to 250, yielding 4659 unique OTUs. Taxonomy was then assigned to the representative sequence for each MED node by matching it to the SILVA 128 (Quast et al., Reference Quast, Pruesse, Yilmaz, Gerken, Schweer, Yarza, Peplies and Glockner2013) database clustered at 99% similarity in QIIME using the closed reference OTU picking pipeline with uclust V1.2.22q (Edgar, Reference Edgar2010). OTUs that matched exactly a reference sequence in SILVA inherited the reference accession, taxonomy and sequence. Taxonomy was assigned to OTUs that did not match exactly a reference sequence using assign_taxonomy.py (QIIME) at 99% sequence similarity, and then at 97% for sequences that had no match at 99%. A matrix of read counts per sample per OTU (hereby referred to as OTU table) was transcribed into biom format. Chimeric, chloroplast, mitochondrial, sequences unassigned at the domain level, and eukaryotic sequences were filtered out. For each sample we removed all OTUs with less than 0.01% abundance for that sample to minimize potential cross-contamination across wells. Lastly, samples with fewer than 1000 reads were removed. The final OTU table across all studies consisted of 4622 unique sequences and 41 942 523 reads, with a mean of 58 012 reads per sample. Representative sequences were aligned with PyNAST (Caporaso et al., Reference Caporaso, Bittinger, Bushman, DeSantis, Andersen and Knight2010a) in QIIME and a phylogenetic tree was generated using RAxML's EPA placement algorithm (Berger et al., Reference Berger, Krompass and Stamatakis2011) and CAT model, with the SILVA 128 tree as a guide tree. Sequencing data and full MiMARKs compliant metadata are accessioned at the European Bioinformatics Institute, accession number PRJEB25354.
Analysis and visualization of sequencing data
Analyses and visualizations of sequencing data were conducted using R version 3.4.1 (Team, Reference Team2016). Rarefaction, α diversity, ordinations for β diversity and distance matrices were calculated using Phyloseq (McMurdie and Holmes, Reference McMurdie and Holmes2013). We use the Chao 1 index of richness (Chao, Reference Chao1984) for α diversity analyses. β diversity was calculated using unweighted UniFrac (Lozupone and Knight, Reference Lozupone and Knight2005) and Bray–Curtis (Bray and Curtis, Reference Bray and Curtis1957). Both beta diversity metrics gave similar results and we present Bray–Curtis, which takes into account relative abundance. For α and β diversity analyses, the data were rarefied to the minimum sample count for the particular sample set in question, which corresponds to 36 000 for experiment 1A, 19 000 for experiment 1B, 25 000 for experiment 1C and 5000 for experiment S11. PERMANOVA and β dispersion analyses were done with vegan (Oksanen et al., Reference Oksanen, Blanchet, Friendly, Kindt, Legendre, McGlinn, Minchin, O'Hara, Simpson, Solymos, Stevens, Szoecs and Wagner2017). Differential abundance analyses were done with DESeq2 (Love et al., Reference Love, Huber and Anders2014) on non-rarefied data. Plots were made with ggplot2 (Wickham, Reference Wickham2009) in R. We used an α value of 0.01 for DESeq, which tends to be conservative and an α of 0.05 for t-tests comparing α diversity between treatment groups over time, PERMANOVA, and β dispersion analyses; all P values were Benjamini–Hochberg corrected.
Results
Impact of mature H. diminuta on moderate colitis
Mature H. diminuta reduce inflammation following colitis induction, but do not alter disease progression. Systemic inflammation as measured by TNFα gene expression in the blood is significantly lower 2 days after colitis induction in the rats harbouring mature H. diminuta (day 21; t-test: P = 0.015, df = 2; Fig. 2A), but not 1 or 3 days after colitis induction (Fig. 2A). We see elevated IL-4 and IL-13 gene expression in the presence of H. diminuta in splenic tissue at day 9 (Fig. 2C and D), indicating a type 2 immune response. However, IL-10 does not differ between groups (Fig. 2E), likely because the peak in IL-10 gene expression is over by 9 days post-colonization (Figs 3B and 4B). There is a non-significant trend towards faster recovery in rats with H. diminuta. Haematochezia (blood in stool) is absent at 3 days post-colitis (0 of three rats) and mean fecal consistency is 3.7 for rats with H. diminuta, indicating the return to solid stools (Table 1). In comparison, within the control group 2/3 rats have haematochezia and all rats still have diarrhoea (fecal consistency of 2; Table 1). Weight did not differ between the two treatment groups (Fig. 2D).

Fig. 2. Effect of mature Hymenolepis diminuta on moderate colitis. Hymenolepis diminuta treatment group in black triangle (N = 3) and control group in grey diamonds (N = 3); both groups were sampled on the same day, but are offset for visualization. Colonization and colitis induction are labelled with vertical dashed lines. (A) TNFα relative gene expression relative to ubiquitin C housekeeping gene, both measured from peripheral blood. (B) Rat body weight. (C–E) cytokine gene expression relative to ubiquitin C housekeeping gene measured from splenic tissue collected at days 0, 9, 17, 22 after sacrifice, from three animals each time. (C) IL-4 relative gene expression. (D) IL-13 relative gene expression. (E) IL-10 relative gene expression. Differences between groups calculated with Welch's t-tests followed by Benjamini–Hochberg correction. Error bars are standard error. * P = 0.05–0.01, ** P = 0.01–0.001, *** P < 0.001. See Table 1 for corresponding clinical data.

Fig. 3. Effect of mature Hymenolepis diminuta on severe colitis. Hymenolepis diminuta treatment group in black triangle (N = 10) and control group in grey diamonds (N = 10); both treatment groups were sampled on the same day, but are offset for visualization. Several rats were sacrificed due to poor condition during the experiment; see Table 1. (A) TNFα gene expression relative to ubiquitin C housekeeping gene. (B) IL-1β gene expression relative to ubiquitin C housekeeping gene. (C) IL-10 relative gene expression relative to ubiquitin C housekeeping gene. (D) % weight loss following colitis induction calculated by comparing with weight at day 20. Differences between groups calculated with Welch's t-tests followed by Benjamini–Hochberg correction. (A–C) error bars are standard error, (D) error bars are standard deviation. *P = 0.05–0.01, **P = 0.01–0.001, ***P < 0.001. See Table 1 for corresponding clinical data.
Model of severe colitis
We established a model of severe colitis in order to study the effects of H. diminuta on longer-term inflammation, clinical outcomes and the microbiota (Fig. S1), inspired by a mouse model of severe DNBS-induced colitis (Martín et al., Reference Martín, Chain, Miquel, Lu, Gratadoux, Sokol, Verdu, Bercik, Bermudez-Humaran and Langella2014). DNBS was injected rectally 3 days apart, and the second injection is a half dose. The resulting colitis persists 9–10 days and is characterized by significantly elevated inflammation (measured by TNFα relative gene expression) that peaks at 6 days post-colitis (dpc; here and throughout refers to days post-initial colitis; for severe colitis a second DNBS injection is given 3 days dpc), and persists through 10 days (Fig. S1B). Severe colitis is accompanied by significant weight loss (roughly 15% of total body weight; Fig. S1B). All animals with induced colitis had haematochezia and diarrhoea through 6 dpc (Table S1). Rats also showed other clinical signs of disease including apathy, fur coat with no gloss and ragged backs. Animals were recovering at 10 dpc: none had haematochezia, mean fecal consistency increased to 2.7 (Table S1), and fur and activity levels were returning to normal.
Impact of mature H. diminuta on severe colitis
Mature H. diminuta appears to ameliorate the effects of severe colitis (Fig. 1, experiment 1B). We observe significantly lower inflammation as measured by TNFα relative expression in the rats colonized with H. diminuta at 6 dpc (day 27; Welch's t-test: corrected P = 0.001, df = 10.7) and 8 dpc (day 29; Welch's t-test: corrected P = 0.006, df = 9.7), and a trend towards reduced inflammation at 4 dpc (day 25; Welch's t-test: corrected P = 0.069, df = 14.6; Fig. 3A). We also observe significantly lower expression of pro-inflammatory cytokine IL-1β at 6 and 8 dpc (days 27 and 29; Fig. 3B). Expression of IL-10 peaks shortly after H. diminuta colonization and is not elevated during colitis (Fig. 3C). Reduced inflammation during colitis is accompanied by significantly less severe weight loss (Fig. 3D). Weight loss for rats with H. diminuta peaks at 4 dpc (day 25) with an average loss of 12% body weight followed by weight gain, on average (Fig. 3D). Weight loss for control animals receiving only DNBS peaks at 8 dpc (day 29) with an average weight loss of nearly 18% of body weight (Fig. 3D). Weight loss is significantly different between treatment groups at 6 dpc, with an average weight loss of 9% in the H. diminuta group vs 17.7% in the control group (day 27; average loss for Welch's t-test: corrected P = 0.016, df = 11.5) and 8 dpc (day 29; Welch's t-test: corrected P = 0.016, df = 13.8). Rats with H. diminuta recover more quickly. At 6 dpc all rats with H. diminuta are free of haematochezia, while half of the rats in the control group have hematochezia (χ 2 test: P = 0.02). The average fecal consistency is also higher in the rats with H. diminuta (Table 1), and they exhibited other signs of recovery, with more activity and a gradual return to glossy coat beginning at 6 dpc. We note that the effects of colitis appear to have been less severe overall in this experiment as the control group returns to solid stools and no haematochezia faster than in experiment 1C or during optimization of colitis (Table 1 and Table S1), though weight loss trends are similar across experiments (Figs 3D and 4D, and Fig. S1C).
Impact of immature H. diminuta on severe colitis
Previous work has shown that the initial immune response to H. diminuta is critical for protective effects against models of inflammatory disease in mice (Hunter et al., Reference Hunter, Wang, Hirota and McKay2005; McKay, Reference McKay2010). Thus, we tested the effect of H. diminuta on severe colitis in the pre-patent period of colonization larval stages and later immature adults are present and are the most immunogenic (McKay, Reference McKay2010; Parfrey et al., Reference Parfrey, Jirků, Šíma, Jalovecká, Sak, Grigore and Jirků Pomajbíková2017). Colitis was induced 4 days after H. diminuta inoculation, and again 3 days later (Fig. 1C). Inflammation is significantly lower in the H. diminuta treatment group, as measured by TNFα and IL-1β relative gene expression between experimental and control rats 1 dpc (day 6; Welch's t-test: corrected P < 0.05; Fig. 4A and B). There is also strongly significant elevation of IL-10 gene expression 1 dpc, which corresponds to 6 days post-colonization, indicating a short activation of type 2 immune response (Fig. 4C). There was no difference in weight loss or clinical parameters between groups (Fig. 4D and Table 1).
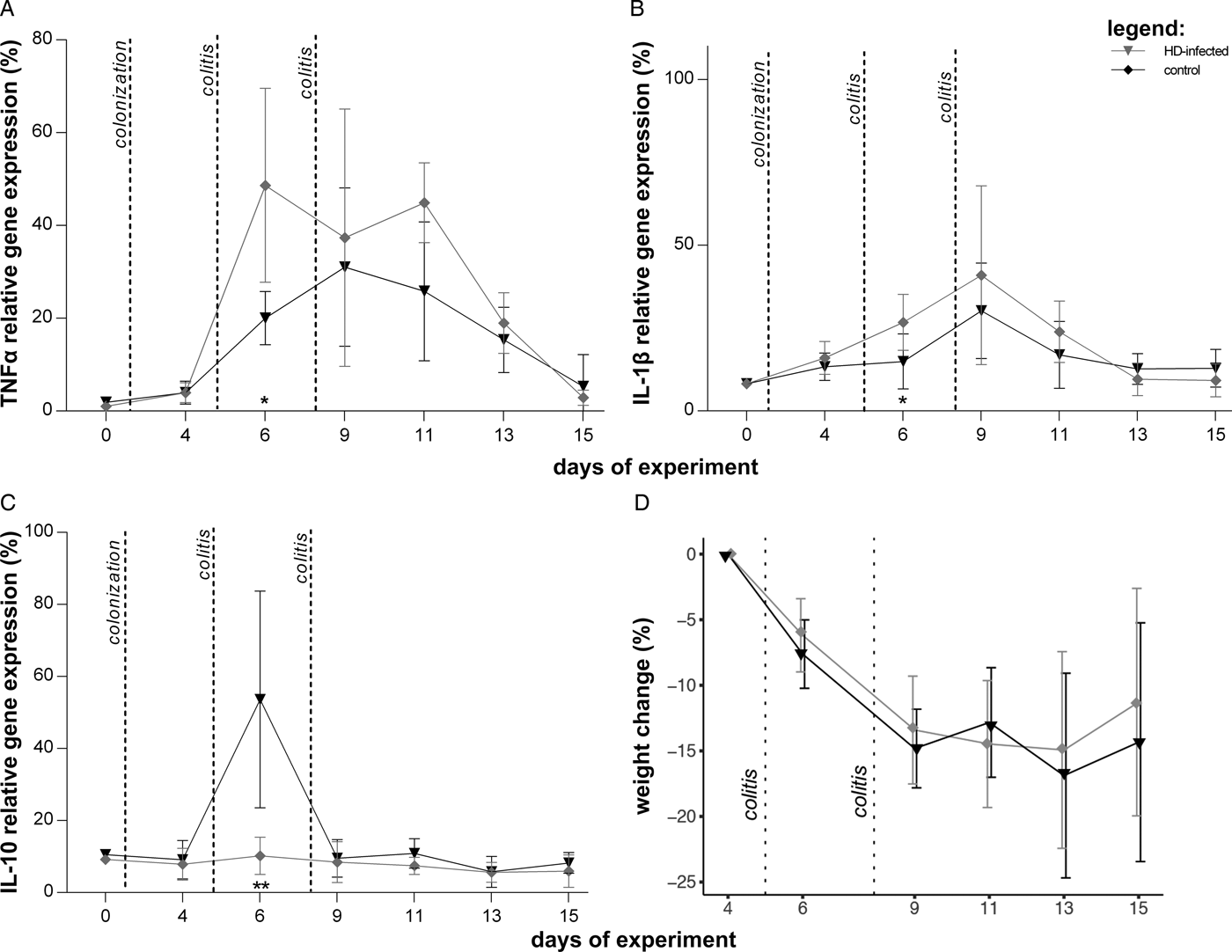
Fig. 4. Effect of immature Hymenolepis diminuta on severe colitis. Hymenolepis diminuta treatment group in black triangle (N = 10) and control group in grey diamonds (N = 10); both treatment groups were sampled on the same day, but are offset for visualization. Several rats were sacrificed due to poor condition during the experiment, see Table 1. (A) TNFα gene expression relative to ubiquitin C housekeeping gene. (B) IL-1β gene expression relative to ubiquitin C housekeeping gene. (C) IL-10 relative gene expression relative to ubiquitin C housekeeping gene. (D) % weight loss following colitis induction calculated by comparing with weight at day 4. Other notes as in Fig. 3.
Gut microbiota
Given the apparent protective effect of mature H. diminuta against severe colitis (experiment 1B), we focused microbial analyses on this experiment. Initially, both treatment groups have similar α diversity (at day 4; the first day of extensive sampling) (Fig. 5A). At the beginning of the patent period, and prior to colitis induction, rats colonized with H. diminuta have significantly lower diversity (Fig. 5A). Diversity drops for all groups with colitis induction, and rebounds more quickly in the H. diminuta treatment group, though diversity remains below pre-colitis levels 10 dpc (Fig. 5A). Substantial variation across individuals means the difference between treatment groups post-colitis is not significant. As richness is lower during active colitis, we see correlations between low α diversity and the markers of colitis, including weight loss, haematochezia, elevated TNFα expression and diarrhoea.

Fig. 5. Change in the gut microbiota in experiment 1B: effect of mature Hymenolepis diminuta on severe colitis. (A) Chao 1 metric of richness over time. Thin lines are individual rats; thick line represents the mean. Significant differences between treatments assessed by Welch's t-tests followed by Benjamini–Hochberg correction represented by *. Dashed vertical lines represent DNBS colitis induction. (B) OTUs that are significantly differentiated between H. diminuta colonized and uncolonized control treatment groups across the whole experiment. (C) OTUs that are significantly differentiated between sample type: fecal pellets (normal stool) and fecal swabs (diarrhoea). Differential relative abundance estimated with DESeq2 followed by Benjamini–Hochberg correction to an α of 0.01. Each circle is one differentially abundant OTU. OTUs are coloured by class and arranged according to genus.
Hymenolepis diminuta and control treatment groups harbour compositionally distinct communities in the pre-patent period and before colitis is induced (Table 2). Sampling problems at day 0 mean that our first samples come from 4 days post-colonization, preventing robust determination of whether communities differ because of H. diminuta colonization or happened to be different prior to colonization. We note that cage mates were reassigned during the experimental acclimation period, 7 days prior to the H. diminuta colonization (day-7) with the aim of homogenizing microbiota between treatments. Similarity within cages explains 21% of the variation overall community composition variation before colitis, while colonization status explains 7% of variation and 66% is unexplained (R 2 values from Adonis; Table 2). Communities change over time before and after colitis induction (Fig. S2), and were also observed to change over time in a previous study (Parfrey et al., Reference Parfrey, Jirků, Šíma, Jalovecká, Sak, Grigore and Jirků Pomajbíková2017). Thus, overall Adonis analyses are conducted with experiment day as a strata, and therefore experiment day is not a significant factor. Differences among cages become stronger and differences by colonization status are maintained as time progresses before colitis is induced (days 4–20; Fig. S2 and Table S2). However, similarity among cagemates and colonization treatment is disrupted by colitis: neither are significant explanatory factors on individual days past day 22 (1 dpc; Table S2). Colonization status remains a significant explanatory factor post-colitis and overall (Adonis, P = 0.001), but explains only ~2% of the overall variation in community composition (Table 2). These differences by colonization status are largely driven by differential abundance of common members of the rodent gut microbiota, including Bacteroides, Bacteroidales S24-7 group, Butryicimonas (Bacteroides) and Ruminococcaceae and Lachnospiraceae (Clostridia) (LRT test within DESeq2, Fig. 5B); these clades contain some OTUs that are enriched with H. diminuta and some enriched in the uncolonized treatment (Fig. 5B and Table S4). Lactococcus, Lactobacillus and Escherichia are less abundant in the group with H. diminuta (Fig. 5B). Sample type (fecal pellets, representing normal stool or fecal swabs from animals with diarrhoea), explained 20% of variation in the whole experiment, while sample type and haematochezia explained roughly 20% variation in the post-colitis period (Table 2). Taxa are enriched during the diarrhoea that accompanies colitis include Escherichia, Bacteroides and Streptococcus (Fig. 5C).
Table 2. Adonis analysis of community composition during experiment 1B: the effect of mature H. diminuta on severe colitis

a H. diminuta refers to Hymenolepis diminuta colonized or uncolonized controls.
b Sample type: refers to swabs collected for animals with diarrhoea and loose stools, or pellets.
c Day: Adonis analysis run with experiment day as a strata to account for changes by day. Sequential model with factors added in the order above.
We see contrasting microbiota composition patterns when severe colitis is induced in the pre-patent period when H. diminuta are immature (Fig. 1, experiment 1C) compared with mature (Fig. 1, experiment 1B). Here the microbiota between H. diminuta colonized and uncolonized control rats becomes more distinct following colitis induction. Colonization status is a significant explanatory factor of community composition throughout the experiment, but explains only ~2% of the variation before colitis induction at day 4 and overall (Table S3). The variability explained by colonization status increases to ~5% post-colitis induction (Table S3). This is largely due to a significant enrichment of Akkermansia (Verrucomicrobiaceae) in H. diminuta colonized rats (DESeq2: log2 fold increase = 5.8; P < 0.001); a substantial increase of Akkermansia occurred in seven of 10 rats from four out of five cages (Fig. S3B). Akkermansia is rare in experiment 1B (Fig. 1), but is enriched in uncolonized rats following colitis (Table S4). As in the experiment with mature H. diminuta, we see that α diversity drops following colitis induction and then incompletely rebounds (Fig. S3A). There are no significant differences associated with colonization status (Fig. S3A).
Discussion
Helminths and their products are promising therapeutic avenues for combatting the rise in IMIDs (Cheng et al., Reference Cheng, Jaint, Thomas, Wilson and Parker2015; Fleming and Weinstock, Reference Fleming and Weinstock2015; McKay, Reference McKay2015; Maizels and McSorley, Reference Maizels and McSorley2016). Yet, the results are not universally positive with some clinical trials showing no effect, and reports that helminths can exacerbate other diseases in model systems (Osborne et al., Reference Osborne, Monticelli, Nice, Sutherland, Siracusa, Hepworth, Tomov, Kobuley, Tran, Bittinger, Bailey, Laughlin, Boucher, Wherry, Bushman, Allen, Virgin and Artis2014; McKay, Reference McKay2015). Further, an emerging body of literature makes clear that helminths often alter the rest of the gut ecosystem, including the bacterial microbiota (Reynolds et al., Reference Reynolds, Finlay and Maizels2015), and these interactions can affect disease outcomes (e.g. Zaiss et al., Reference Zaiss, Rapin, Lebon, Dubey, Mosconi, Sarter, Piersigilli, Menin, Walker, Rougemont, Paerewijck, Geldhof, McCoy, Macpherson, Croese, Giacomin, Loukas, Junt, Marsland and Harris2015). Thus, investigating additional systems in which helminths protect against inflammatory disease will enhance understanding of the range of mechanisms and clinical outcomes, and how these might be altered by co-infection or the other components of the gut ecosystem (Reynolds et al., Reference Reynolds, Finlay and Maizels2015). Similarly, it is important to explore additional candidates and existing candidates in diverse context, particularly those that do not harm their host (Lukeš et al., Reference Lukeš, Kuchta, Scholz and Pomajbíková2014).
We show here that the benign tapeworm H. diminuta appears to protect rats from severe colitis, resulting in lower inflammation, less severe weight loss, and faster recovery (Fig. 3; Table 1). Further, we find that H. diminuta does not protect against moderate colitis (Fig. 2). This is consistent with a previous study showing no protective effect of H. diminuta in either the pre-patent or patent period in rats (Hunter et al., Reference Hunter, Wang, Hirota and McKay2005). We do see a modest reduction in TNFα expression, indicating that H. diminuta does reduce inflammation even if it does not result in clinical improvement (Fig. 2A). Moderate colitis consists of a single rectal application of 58 mg DNBS, and is the standard used in most studies (e.g. Wallace et al., Reference Wallace, Le, Carter, Appleyard and Beck1995; Hunter et al., Reference Hunter, Wang, Hirota and McKay2005). Moderate colitis is ameliorated in mouse models by H. diminuta and their products (McKay, Reference McKay2010). We prolonged this model of colitis following Martín et al. (Martín et al. Reference Martín, Chain, Miquel, Lu, Gratadoux, Sokol, Verdu, Bercik, Bermudez-Humaran and Langella2014) by including a second rectal injection containing a half dose of DNBS to enable study of clinical and microbiota changes over a longer time period. The resulting colitis lasts for 8–10 days compared with 3 days, as measured by elevated TNFα expression and clinical recovery (Fig. S1, Table S1). Recovery was assessed by cessation of diarrhoea and haematochezia, stabilization of weight, and return to normal activity levels.
The protective effect of H. diminuta is observed only when rats harbour mature H. diminuta adults at the time of severe colitis induction, and not when they harbour immature H. diminuta (pre-patent period of colonization). There is no difference in the clinical outcomes between DNBS only group and DNBS + H. diminuta in the pre-patent period of colonization (Fig. 4). The pre-patent period is the time when larval helminths as establishing themselves in the small intestines, and when they induce a type 2 immune response (Roberts, Reference Roberts and Arai1980; Webb et al., Reference Webb, Hoque and Dimas2007). Thus, it is likely that immunosuppression induced by H. diminuta adults or their excretory/secretory products are responsible for protection against colitis, and not the initial immune response.
The mechanism of protection was not determined here, but likely involves regulation and suppression of the rat immune system by mature H. diminuta. In mice, the protective effects of H. diminuta are tied to the induction of a type 2 immune response that expels the worm and are dependent on STAT6 (the IL-4/IL-13 transcription factor) and IL-10 (McKay and Khan, Reference McKay and Khan2003; Hunter et al., Reference Hunter, Wang, Hirota and McKay2005; McKay, Reference McKay2010). Rats mount a qualitatively similar type 2 immune response when colonized with excess worms (Webb et al., Reference Webb, Hoque and Dimas2007; McKay, Reference McKay2010), and we have previously documented an increase in IL-10 expression in the patent period (Parfrey et al., Reference Parfrey, Jirků, Šíma, Jalovecká, Sak, Grigore and Jirků Pomajbíková2017). Thus, we hypothesize that the brief anti-inflammatory activity observed in the pre-patent period results from a type 2 immune response and associated increase in IL-10 expression (Fig. 4; Parfrey et al., Reference Parfrey, Jirků, Šíma, Jalovecká, Sak, Grigore and Jirků Pomajbíková2017) which overlaps with the elevation of IL-4 and IL-13 (Fig. 2C and D), but that it is too weak to protect against disease (Fig. 4). However, in mice the anti-colitic effect of H. diminuta is not solely due to induction of the type 2 immune response and IL-10 gene expression; H. diminuta colonization also regulates and suppresses the immune system via induction of regulatory T-cells, B-cells and alternatively activated macrophages (Persaud et al., Reference Persaud, Wang, Reardon and McKay2007; McKay, Reference McKay2010; Reyes et al., Reference Reyes, Wang, Fernando, Graepel, Leung, van Rooijen, Sigvardsson and McKay2015). The intestinal epithelium also plays a direct role in the response to H. diminuta in mice and protection from colitis (Reyes et al., Reference Reyes, Fernando, Lopes, Leung, Mancini, Matisz, Wang and McKay2016). The McKay laboratory has shown that a high molecular weight fraction from adult H. diminuta and excretory/secretory products are immunosuppressive, and that these products can protect against induced colitis (e.g. Wang and McKay, Reference Wang and McKay2005; Johnston et al., Reference Johnston, Wang, Catarino, Ball, Phan, MacDonald and McKay2010; Reyes et al., Reference Reyes, Fernando, Lopes, Leung, Mancini, Matisz, Wang and McKay2016). Similarly, helminth products from other species are commonly found to be immunoregulatory and mitigate inflammatory disease (Maizels and McSorley, Reference Maizels and McSorley2016). For example, Johnston et al., also found HdHMW elevated IL-10 expression, but that the anti-colitic effect of HdHMW was not dependent on IL-10 (Johnston et al., Reference Johnston, Wang, Catarino, Ball, Phan, MacDonald and McKay2010) consistent with study Melon et al. (Reference Melon, Wang, Phan and McKay2010), while Persaud et al., showed that the anti-colitic effect of H. diminuta is dependent on CD4 + (predominately T-cells) cells, though worm expulsion is not (Persaud et al., Reference Persaud, Wang, Reardon and McKay2007). Consistent with the expectation that H. diminuta adults are protecting rats via immunosuppression, we previously documented immunoregulation during the patent period of H. diminuta colonization in rats, consisting of reduced lymphocyte numbers (Parfrey et al., Reference Parfrey, Jirků, Šíma, Jalovecká, Sak, Grigore and Jirků Pomajbíková2017). Future studies elucidating the mechanisms that underlie H. diminuta’s protective effects against severe colitis in rats will further the development of H. diminuta, or its products, as a therapy against IMIDs. Future work should investigate inflammation at the site of injury or disease as well as systemic inflammation, which was investigated here.
We find that the microbiota changes in response to H. diminuta colonization. In each experiment we see a drop in diversity at colitis induction followed by increasing diversity during recovery. Community composition does not return to the pre-colitis state and diversity does not fully rebound, suggesting that the microbiota takes longer than ten days to recover and/or that colitis pushes the microbiota to an alternative state. Overall, changes in community composition are small and are not consistent across experiments. We find that compositional differences associated with H. diminuta are largely disrupted by severe colitis in the presence of mature H. diminuta (Fig. 5), when we see suppression of clinical symptoms (Fig. 3). However, differences between treatment groups become stronger in experiment 1C (Fig. 1) when colitis was induced in the pre-patent period (Fig. S3), and clinical symptoms do not respond to H. diminuta (Fig. 4). Further, we do not see consistent taxa associated with H. diminuta across experiments here even though experimental conditions are consistent (Fig. 5, Figs S3 and S4). The taxonomic changes observed in response to H. diminuta here also differ from other studies with H. diminuta in rats (McKenney et al., Reference McKenney, Williamson, Yoder, Rawls, Bilbo and Parker2015; Parfrey et al., Reference Parfrey, Jirků, Šíma, Jalovecká, Sak, Grigore and Jirků Pomajbíková2017). For example, we observe a drop in Lactobacillus in H. diminuta colonized animals (Fig. 5, Fig. S3), but Reynolds et al., showed through extensive experimental work that Lactobacillus is associated with, and facilitates, infection with the helminth Heligmosomoides polygryus (Reynolds et al., Reference Reynolds, Smith, Filbey, Harcus, Hewitson, Redpath, Valdez, Yebra, Finlay and Maizels2014). This points to the importance of the microbial pool available for colonization in establishing the microbiota. We note that we obtained all rats from the same supplier in the same condition, but we did not test the initial microbiota of newly acquired rats; future studies should do so. Moving forward, it will be important to investigate the functional significance of microbiota changes in H. diminuta, for example using SCFAs, especially when they vary taxonomically. We note that the clinical severity of colitis varies across experiments and is generally severe in experiment 1B compared with 1C and the optimization of colitis (Table 1 and Table S1). It is possible that some of this variation is due to microbiota variation across experiments. Future work should explicitly test the impact of microbiota composition as a cause of variation in protection; doing so with natural vs laboratory conditions, as done in Williamson et al. (Reference Williamson, McKenney, Holzknecht, BElliveau, Rawls, Poulton, Parker and Bilbo2016), would provide additional relevance.
Supplementary material
The supplementary material for this article can be found at https://doi.org/10.1017/S0031182018000896
Acknowledgements
Thanks to Jordan Lin, Cody Foley, Cassandra Jensen, Marcus Campbell, Oldřiška Hložková and Zuzana Lhotská for laboratory assistance. Radek Šíma, Mirka Soldánová and Pascale Vonaesch for advice on immunological and statistical analyses. This manuscript was improved following comments from two anonymous reviewers.
Financial support
Human Frontier Science Program Young Investigators grant (RGY0078/2015) to K.J.P. and L.W.P.
Conflicts of interest
None.
Ethical standards
This study was carried out in strict accordance with the recommendations in the Czech legislation (Act No. 166/1999 Coll., on veterinary care and on change of some related laws, and Act No. 246/1992 Coll., on the protection of animals against cruelty). The present experiments and protocols were approved by the Committee on the Ethics of Animals Experiments of the Biology Centre of the Czech Academy of Sciences (České Budějovice, Czech Republic; permit number: 1/2014) and by the Resort Committee of the Czech Academy of Sciences (Prague, Czech Republic).