Introduction
There are many kinds of red herrings, misuses of history, untestable hypotheses, hypotheses that are accepted without test, concepts that persist out of context.—David K. Jacobs (Reference Jacobs1996: p. 614)
Although Recent crinoids are dominated by motile taxa displaying crawling and sometimes swimming abilities, they exclusively occupy benthic ecological niches (Macurda and Meyer Reference Macurda and Meyer1974; Meyer and Macurda Reference Meyer and Macurda1977, Reference Meyer and Macurda1980; Meyer et al. Reference Meyer, LaHaye, Holland, Arenson and Strickler1984; Baumiller and Messing Reference Baumiller and Messing2007). In the geologic past, however, some taxa are thought to have been adapted to nektonic, planktonic, and pseudo-planktonic modes of life (Seilacher and Hauff Reference Seilacher and Hauff2004; Hess Reference Hess2010; Hagdorn Reference Hagdorn2011). Notably, the most dramatic morphologic innovations and expansion into unexplored niches, considered as macroevolutionary responses to increased benthic predation, occurred during the early Mesozoic (Baumiller et al. Reference Baumiller, Salamon, Gorzelak, Mooi, Messing and Gahn2010; Gorzelak et al. Reference Gorzelak, Salamon and Baumiller2012). However, a planktonic mode of life, although rare, is also thought to have characterized some Paleozoic crinoids. One example are scyphocrinoids (Scyphocrinitidae sensu Jaekel Reference Jaekel1918) (Fig. 1A). This stratigraphically important group of camerate crinoids is widely distributed in strata spanning the upper Silurian (Pridoli) to the Lower Devonian (Lochkovian) (e.g., Donovan and Lewis Reference Donovan and Lewis2009; Valenzuela-Ríos and Liao Reference Valenzuela-Ríos and Liao2012; Racki et al. Reference Racki, Baliński, Wrona, Małkowski, Drygant and Szaniawski2012; Corriga et al. Reference Corriga, Corradini, Haude and Walliser2014; Donovan and Miller Reference Donovan and Miller2014; Haude et al. Reference Haude, Corriga, Corradini, Walliser, Wiese, Reich and Arp2014). Scyphocrinoids have a stem as long as 3 m, an ~10-cm-high calyx, with large interradial areas comprising fixed small plates and pinnules (for Scyphocrinites see Ubaghs Reference Ubaghs, Ubaghs, Moore and Teichert1978a: Fig. 292; for other closely related lobolith-bearing genera, such as Marhoumacrinus, Carolicrinus, and Camarocrinus see Hess Reference Hess, Hess, Ausich, Brett and Simms1999: Fig. 112; Haude et al. Reference Haude, Corriga, Corradini, Walliser, Wiese, Reich and Arp2014: Fig. 8). The arms, as much as ~30 cm long, become free above the tertibrachials; the primibrachials and secundibrachials with their pinnules are all fixed in the calyx. Its most unusual morphologic feature, not observed in any other crinoid, is a large, typically ~10–20 cm in diameter (maximum recorded size ~30 cm), bulbous, chambered organ at the distal end of the stem, the so-called lobolith (Brett Reference Brett1981). Two types of loboliths have been recognized: (1) the subspherical “cirrus loboliths,” with many unequal chambers made of numerous branching cirri (Fig. 1B); and (2) the assumed to be more advanced “plate loboliths,” with four to seven large chambers, that are outwardly open near the first bifurcation of a main cirrus within a characteristic collar area, a curved, bilaterally symmetric root trunk, and a simplified wall structure composed of two to three layers of thin plates (Fig. 1C) (for a detailed morphologic description, see Haude Reference Haude1972).
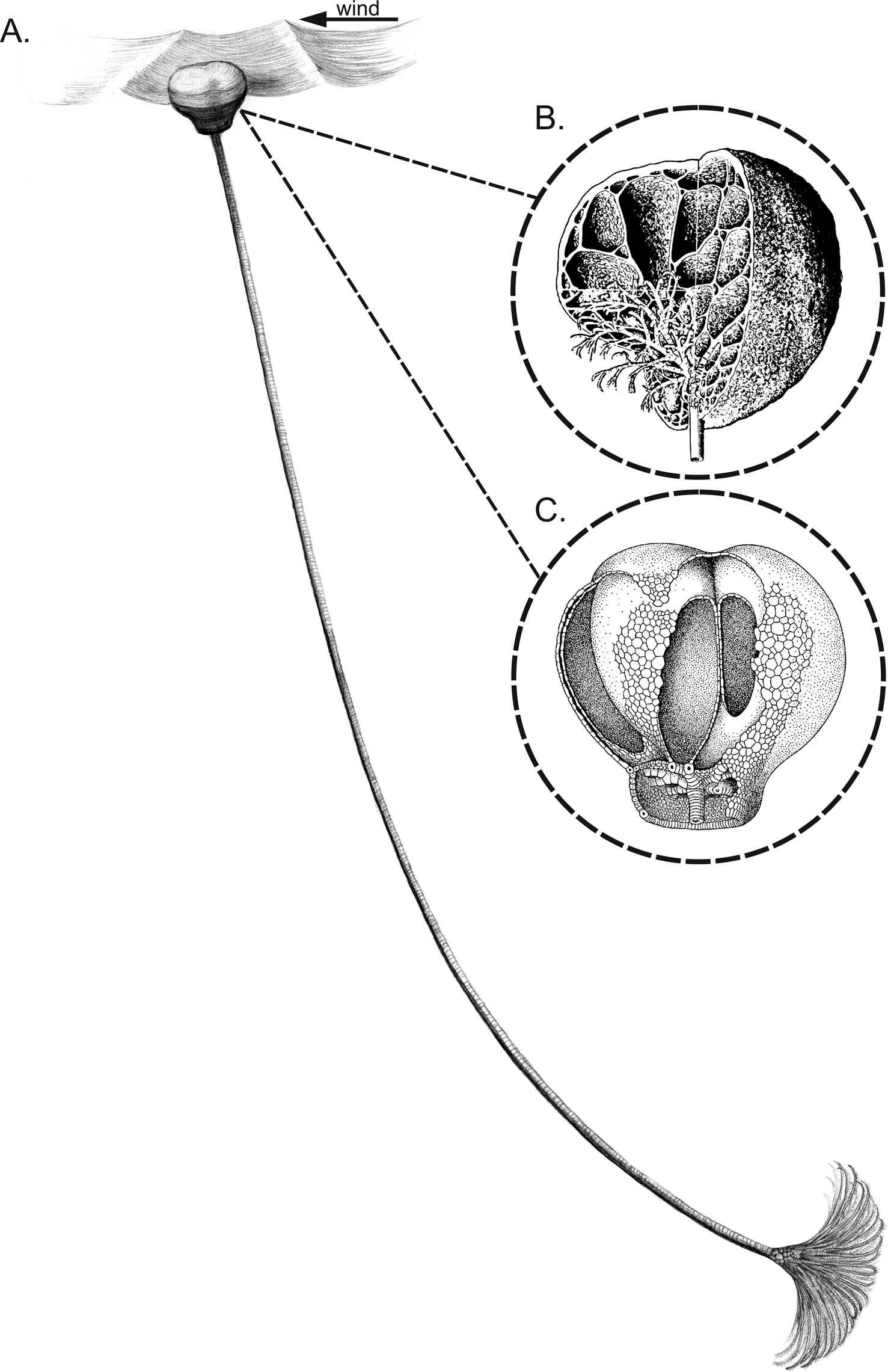
Figure 1. A, Example of traditional reconstruction of scyphocrinoid as a pelagic, floating crinoid along with reconstructions of cirrus (B) and plate (C) loboliths (B and C taken from Hess [2010] after Haude [1972] and Hess [1999]).
Loboliths have been the subject of much controversy. They have been variously interpreted as: (1) representing another class of echinoderms (e.g., Barrande and Waagen Reference Barrande and Waagen1887; Sun and Szetu Reference Sun and Szetu1947), (2) brood-pouches (Haeckel Reference Haeckel1896; Jahn in Schuchert Reference Schuchert1904), (3) genital organs (Jaekel Reference Jaekel1904), (4) holdfasts (Springer Reference Springer1917; Ray Reference Ray1980; Brett Reference Brett1984), (5) parasitic cysts induced by myzostomids (Haeckel Reference Haeckel1896; Kirk Reference Kirk1911; Abel Reference Abel1920; Ehrenberg Reference Ehrenberg1926), and (6) buoyancy/floating organs (e.g., Hall Reference Hall1879; Schuchert Reference Schuchert1904; Bather Reference Bather1907; Haude Reference Haude1972, Reference Haude1992; Hess Reference Hess, Hess, Ausich, Brett and Simms1999, Reference Hess2010; Prokop and Petr Reference Prokop and Petr2001; Seilacher and Hauff Reference Seilacher and Hauff2004; Haude et al. Reference Haude, Corriga, Corradini, Walliser, Wiese, Reich and Arp2014). This last hypothesis implies that scyphocrinoids were planktonic and tow-net filtrators: their buoyant lobolith was moved along by currents, dragging along the crown, which was suspended below (Fig. 1A). Notably, this interpretation has been adopted in the last two editions of the Treatise on Invertebrate Paleontology (Ubaghs Reference Ubaghs, Ubaghs, Moore and Teichert1978b; Hess Reference Hess2010).
Arguments used to support the planktonic hypothesis include: (1) functional macro-morphology (e.g., bulbous shape and chambered structure of lobolith, long stem, and dense filtration fan), (2) taphonomy (e.g., wide geographic distribution of loboliths, which are typically found without the crown), (3) paradigm analysis (approximation to a mechanical paradigm, combined with recognition of an evolutionary trend from a cirrus lobolith to a plate lobolith, indicating increasing functional efficiency of lobolith), and (4) theoretical buoyancy calculations (for a review, see Hess Reference Hess, Hess, Ausich, Brett and Simms1999, Reference Hess2010). However, although buoyancy of scyphocrinoids is indeed theoretically possible (as inferred from published buoyancy calculations), as we show in this paper, other arguments used to support the planktonic hypothesis are flawed and not persuasive.
Two different models for a planktonic mode of life have been suggested for scyphocrinoids. According to the first, widely accepted interpretation (e.g., Haude Reference Haude1972, Reference Haude1992; Hess Reference Hess, Hess, Ausich, Brett and Simms1999, Reference Hess2010; Haude et al. Reference Haude, Corriga, Corradini, Walliser, Wiese, Reich and Arp2014), a positively buoyant lobolith of scyphocrinoids passively drifted at the water's surface. A more elaborate hypothesis suggests that these crinoids were able to actively adjust their buoyancy, allowing them to float at any depth below the water's surface, taking advantage of an interface of two water bodies in a stratified sea (Seilacher and Hauff Reference Seilacher and Hauff2004).
A prerequisite for becoming planktonic is the development of a leak-tight buoyant structure and a gas-generating mechanism. After more than a century since the idea of scyphocrinoid lobolith as a floating organ was introduced, many questions remain regarding its development, form, and function. Perhaps the most germane is that “the generation of gas to fill the bulb [lobolith] has so far not been explained in a satisfactory way and the issue of whether it actually occurred appears unresolvable at present” (Hess Reference Hess, Hess, Ausich, Brett and Simms1999: p. 98). Here, we synthesize and critically evaluate previous arguments implying a planktonic mode of life of scyphocrinoids, provide new evidence from stereom microstructure and theoretical calculations that challenge that interpretation, and suggest that this enigmatic crinoid group was benthic.
Materials and Methods
Eleven well-preserved loboliths (~3–15 cm in diameter) from three museum collections were selected for microstructural analyses (Figs. 2–4). The specimens came from two regions. The majority of loboliths (six plate and two cirrus loboliths) are from the uppermost Silurian (Pridoli) of the Tafilalt area near Erfoud, Morocco (collections of the Museum für Naturkunde Berlin, Leibniz Institut, and the Department of Earth Sciences, Laboratory of Palaeontology and Biostratigraphy, University of Silesia). Three plate loboliths are from the lowermost Devonian of Ada, Oklahoma, USA, with precise locality unknown (collections of the Orton Geological Museum, Ohio State University).

Figure 2. Microstructure of scyphocrinoid loboliths. A, B, Spiny ornamentation of distal plates from the outermost lobolith wall. C, D, Magnifications of spines. E, Suture line between two plates. F, Outermost microperforate stereom layer. G, H, Outermost microperforate fascicular-like stereom layer near the collar. I, Relicts of labyrinthic stereom inside plate. J, Three-layered loosely packed lobolith wall composed of plates displaying the homogenous labyrinthic microfabrics. K, External plates composed of coarse and dense labyrinthic stereom. L, External plates composed of coarse and dense labyrinthic stereom. A–I, SEM images. J–L, Optical microscope images, cross sections. Scale bars, 1 mm (A), 0.5 mm (B, J–L), 0.2 mm (C–E), 0.1 mm (F, G, I), 0.05 mm (H).

Figure 3. Microstructure of scyphocrinoid loboliths in cross sections under optical (A, C, E, G) and cathodoluminescence (B, D, F, H) microscopy. A, B, Two layers of plate lobolith composed of relicts of homogenous labyrinthic stereom. C, D, External lobolith wall revealing relicts of labyrinthic stereom. E, F, External plate composed of coarse labyrinthic stereom (LS) and outermost microperforate stereom layer (MSL). G, H, Cirrus-type lobolith wall composed of loosely packed cirri displaying relicts of homogenous coarse labyrinthic microfabrics. Arrows in A and C show the outermost lobolith spines composed of microperforate stereom layer. Scale bars, 0.5 mm.

Figure 4. Distribution of stereom microstructure in scyphocrinoid loboliths.

Figure 5. A schematic of a scyphocrinoid as a tow-net filtrator submerged in a fluid, flowing from left to right, with a vertical velocity gradient indicated by the arrows on the left. At equilibrium, with the sum of the vertical and horizontal forces (denoted by “F” with subscripts) equal 0, the scyphocrinoid is moving to the right with an absolute velocity U filter (= U lobolith). The drag force on the lobolith, acting to the right, is proportional to the lobolith's relative velocity (U current − U lobolith)2, while an equal and opposite drag force acts on the filter and is proportional to its relative velocity (U filter − U ambient)2. The position of a filter relative to the lobolith expressed by the angle of its stalk relative to horizontal, θ, is the inverse tangent of the ratio of the downward force on the filter to drag force on the filter (θ = arctan (F down-filter /F drag-filter)). For a detailed discussion of the variables and governing equations see “Materials and Methods.”
The stereom microstructure of selected ossicles from different parts of the loboliths was investigated using a scanning electron microscope (SEM), a Philips XL-20 at the Institute of Paleobiology of the Polish Academy of Sciences in Warsaw. Additionally, 36 variously oriented thin sections, polished down to ~25 μm and coated with carbon, were examined under an optical cathodoluminescence (CL) microscope equipped with a hot cathode and a Kappa video camera at the Institute of Paleobiology of the Polish Academy of Sciences in Warsaw. The following parameters were used for CL microscopy: an electron energy = 14 keV and a beam current = 0.05–0.07 mA. Previous studies have proven that CL microscopy is especially useful in assessing diagenesis and also in revealing primary microstructural details of differently preserved echinoderm ossicles from a range of stratigraphic intervals and rock types (Gorzelak and Zamora Reference Gorzelak and Zamora2013, Reference Gorzelak and Zamora2016; Gorzelak et al. Reference Gorzelak, Głuchowski and Salamon2014, Reference Gorzelak, Głuchowski, Brachaniec, Łukowiak and Salamon2017; Gorzelak Reference Gorzelak2018). Some quantitative data, such as thickness of trabecular bars and diameters of pores, were also obtained using ImageJ from CL microphotographs (30 measurements per each microstructurally distinct area).
The specimens, samples, and thin sections are housed at the Museum für Naturkunde Berlin, Leibniz Institut (acronyms: MB.E.11672, MB.E.11673, MB.E.11674), Department of Earth Sciences, Laboratory of Palaeontology and Stratigraphy, University of Silesia, Sosnowiec (acronyms: GIUS 2-3684-3688), and the Orton Geological Museum, Ohio State University (acronyms: OSU54131-E, OSU54131-B, OSU54131-F).
The effectiveness of the hypothesized tow-net mode of feeding of scyphocrinoids was tested analytically and computationally using biomechanical principles (Figs. 5–7; see eqs. 1–7). The objective of the modeling approach presented herein is to determine a relative velocity of the crinoid filtration fan (i.e., velocity between the filtration fan and the ambient velocity through which the filtration fan was moving), as water movement past the filter is a necessary condition for filter feeding. This model considers the filtration fan and lobolith submerged within a moving fluid with some vertical velocity gradient (Fig. 6), such that the current at the lobolith (hence referred to as “current velocity”) is higher than at the filtration fan suspended below (hence referred to as “ambient velocity”). The lobolith, acted on by drag, pulls the filter, which due to its movement, experiences a retarding drag force in opposition to the drag acting on the lobolith. The filter thus acts in a fashion analogous to a sea anchor or a drogue, slowing down the lobolith. At equilibrium, the filter and lobolith must be moving at exactly the same absolute, steady velocity, and the drag forces on both lobolith and filter must be equal and opposite. In the model, the vertical forces include the downward force on the crown, equivalent to its weight in water, and an upward buoyancy force due to the buoyant lobolith. It is assumed that the buoyancy force is equal in magnitude to the downward force, such that the lobolith maintains a stable vertical position in the water column, whereas the vertical position of the filter below the lobolith is controlled by the angle of the stalk; the latter is determined by the balance of vertical and horizontal forces.

Figure 6. Two vertical velocity gradients used in modeling a scyphocrinoid as a tow-net filtrator: (A) gradual velocity gradient (k = −0.2) and (B) steep velocity gradient (k = −2). Three absolute current velocities at the lobolith are shown for each: 25 cm s−1, solid line; 15 cm s−1, dashed line; 5 cm s−1, dotted line. For each of the profiles, as one descends downward from the lobolith, the ambient velocity decreases. The velocity profile is shown for only the topmost 3 m depth below the lobolith, as that is length of the stalk, and thus the deepest reach of a suspended crown of scyphocrinoid.

Figure 7. Effect of absolute current velocity at the lobolith on absolute ambient velocity at the filter (solid black line) and relative velocity at the filter (dashed black line) for a model of scyphocrinoids. The diagonal, dotted gray line (slope = 1) represents the absolute current velocity at the lobolith. In the model, the scyphocrinoid lobolith has a drag coefficient of 0.47, a 3-m-long stalk, and 0.6 m filter diameter with drag coefficient of 0.73. The density of scyphocrinoid skeletal material is 1360 kg m−3, and the lobolith is assumed to provide sufficient buoyancy to counter the crinoid's weight in water. The schematics along the horizontal axis at the top illustrate the angle of the stalk of scyphocrinoid at equilibrium for a range of absolute current velocities (1 to 25 cm s−1), with current direction left to right. A, Steep velocity gradient (k = −2), lobolith diameter = 10 cm. B, Shallow velocity gradient (k = −0.2), lobolith diameter = 10 cm. C, Steep velocity gradient (k = −2), lobolith diameter = 20 cm. Note the magnitude of the relative velocity at the filter is approximately <10% of the absolute current velocity at the lobolith for the most realistic scenario (B) but increases with a steeper velocity gradient (A) or a larger lobolith (C).
For given values of morphologic parameters, coefficients of drag of the lobolith and filter, current velocity, and vertical velocity gradient, the model allows one to solve for the “relative velocity” at the filter, that is, the difference between the absolute ambient velocity at the filter and the absolute velocity of the filter. This relative velocity is equivalent to the velocity of the fluid at the filter of a benthic, sessile crinoid and is the primary variable that determines the rate of supply of particulate nutrients on which the crinoid feeds. It is important to emphasize that in the case where there is no velocity gradient, the absolute velocity of the lobolith and filter would be that of the current, and the resulting “relative velocity” would be 0.
The morphology of the lobolith was based on specimens described in the literature. For most dimensions of the calyx, arms, stalk, and lobolith, we relied on specimens described in Haude (Reference Haude1972) and Hess (Reference Hess, Hess, Ausich, Brett and Simms1999).
We explored the sensitivity of the model to various values of realistic morphologic parameters, including the dimensions of arms, stalk, and lobolith; crinoid filter (proportion of area covered by filter elements, such as arms and pinnules); coefficients of drag of filter and lobolith; and velocity gradients (e.g., Kondo et al. Reference Kondo, Sasano and Ishii1979; Price et al. Reference Price, Weller and Schudlich1987; Schudlich and Price Reference Schudlich and Price1998). Numerical models were run over a broad range of current velocities (1 to 25 cm s−1) that typically occur in Recent environments (e.g., Lumpkin and Johnson Reference Lumpkin and Johnson2013). In each run, the equilibrium stalk orientation, velocity of the filter, and ambient velocity at the filter were obtained.
The following set of governing equations was used in modeling scyphocrinoids as tow-net filtrators:

where U filter is the absolute velocity of the filter, and U lobolith is the absolute velocity of the lobolith.

where F drag-filter is the drag on the filter, and F drag-lobolith is the drag on the lobolith.

where Cdfilter is the coefficient of drag of the filter (0.73: based on filter solidity, area occupied by fibers/total area, of 0.53, and empirical relationship between solidity and Cd in Hoerner [Reference Hoerner1965]), ρfluid is the density of the fluid (1024 kg m−3), A filter is the area of the filter perpendicular to its motion, and U ambient is the absolute velocity of fluid at the filter; (U filter − U ambient represents the relative velocity of filter).

where k is a constant, L is stalk length (3 m), and θ is the angle of the stalk relative to horizontal.

where Cdlobolith is the coefficient of drag of the lobolith assumed to approximate that of a sphere (0.47: Vogel Reference Vogel1981: Fig. 6.5), A lobolith is the cross-section of the spherical lobolith (= π(d/2)2, where d is the lobolith diameter, 0.1 m or 0.2 m), and U current is the absolute velocity of fluid at the lobolith.

where F down-filter is the downward force on the filter, V crown is the volume of the skeletal elements of the crown estimated from dimensions of arms and calyx, ρskeleton is the density of crinoid skeletal elements (1360 kg m−3: Janevski and Baumiller Reference Janevski and Baumiller2010), and g is the gravitational constant (9.8 m s−2).

The equilibrium position of a filter relative to the lobolith expressed by the angle of its stalk relative to horizontal, θ, at any given current velocity, U current, can be found numerically. This is done by calculating the velocities (U ambient, U filter, U lobolith) and forces (F drag-filter, F drag-lobolith) for a given current velocity, U current, for stalk angles θ between 0° and 90°. For a given current velocity, U current, there is a unique angle θ, at which all of the above equations are satisfied; this represents the equilibrium.
Results
Micromorphology.—Walls of cirrus and plate loboliths are composed of two to three layers of tiny ossicles (cylindrical spicules or polygonal plates, respectively), which are made of coarse, structural labyrinthic stereom (mean trabeculae thickness: 12 μm; mean pore diameter: 8 μm; Figs. 2–4). The lateral surface of distal plates from the outermost lobolith wall, however, is strongly ornamented by irregular micrometer-sized wavy ridges, spines, and keels (Figs. 2A–D, 3A–D, and 4). The outermost layer is composed of microperforate stereom, sometimes revealing a weak “en echelon” pattern of elliptical to lanceolate lumina (Figs. 2F, and 4). The lateral surface of proximal ossicles is unornamented and composed of poorly ordered labyrinthic to fascicular-like stereom (Figs. 2G, H, and 4; cf. Smith Reference Smith1980).
Biomechanical Modeling of Scyphocrinoid as Tow-Net Filtrator.—The results of analyses for different scenarios are shown in Figure 7. Of these, the “standard” scenario is represented by Figure 7B. In the model, the scyphocrinoid is assumed to have a 10 cm lobolith with a 0.47 drag coefficient, a 3-m-long stalk, and a 60 cm filter diameter with a drag coefficient of 0.73. The density of scyphocrinoid skeletal material is 1360 kg m−3, and the lobolith is assumed to provide sufficient buoyancy to counter the crinoid's weight in water. More extreme scenarios are shown in Figure 7A and C: Figure 7A illustrates the effect of an extreme vertical velocity gradient (100% decrease over the 3 m length of the stalk), where the filtration fan is being towed through still water, and in Figure 7C, the scyphocrinoid is modeled with a large, 20-cm-diameter lobolith.
Results of the analysis with the “standard” parameter values for scyphocrinoids (Fig. 7B) show that the “relative velocity” of the filter is generally quite low (<10%) compared with the velocity of the current that the lobolith is experiencing. The relative velocity at the filter sets the upper limit on how much of the fluid (and particles in the fluid) directly upstream of the filter can be sampled by the filter; in actuality, the flux of particles will be lower than this value, as the resistivity (proportional to solidity) of the filter leads to some of the approaching fluid (and particles) diverging around the filter's edges (Baumiller Reference Baumiller, Maples and Waters1997).
A slightly higher relative velocity results when the filter is towed through still water, requiring an extremely steep velocity gradient (Fig. 6). Also, a higher relative velocity results when the lobolith is larger and experiences a larger force of drag (Fig. 7C). In this case, equilibrium (where the forces on the lobolith and the filter are equal and opposite) occurs when both the lobolith and the filter are moving at a greater velocity. The net result for the filtrator is that it moves faster relative to the ambient current, and thus it can ”sample” more fluid. Nevertheless, even under such extreme scenarios, the relative velocity does not change dramatically.
Discussion
A Paradigm Approach of Lobolith as Float: Supposed Morphologic and Evolutionary Optimization.—Haude (Reference Haude1972), in his functional interpretation of loboliths, applied Rudwick's (Reference Rudwick1964) paradigm approach by noting morphologic similarities between buoys and scyphocrinitid bulbous-chambered loboliths, and he inferred a trend toward functional optimality (transition from cirrus to plate lobolith; but see below). The interpretation of the lobolith as a floating organ was also explored by Haude (Reference Haude1972, Reference Haude1998) using theoretical buoyancy calculations of the presumed gas infillings. However, it has been generally recognized that the use of the paradigm approach to infer function of fossil organisms has its problems (e.g., Grant Reference Grant1972, Reference Grant1975; Signor Reference Signor1982; Lauder Reference Lauder and Thomason1995); and as stressed by Plotnick and Baumiller (Reference Plotnick and Baumiller2000: p. 305), it is best employed in “generating, rather than testing, functional hypotheses.” Indeed, a bulbous and chambered morphology of loboliths, many of which reveal basal flattening (e.g., Hess Reference Hess, Hess, Ausich, Brett and Simms1999: Fig. 110; Peng Reference Peng2001: Fig. 13; Lee Reference Lee2005: Fig. 11; Haude et al. Reference Haude, Corriga, Corradini, Walliser, Wiese, Reich and Arp2014: Figs. 4, 7c), is not a test of buoyancy, as this shape characterizes many benthic echinoderms, such as echinoids, whose tests are filled by coelomic fluid and are negatively buoyant.
Importantly, our microstructural data suggest that the walls of cirrus and plate loboliths were not well protected against possible leaks of gas and/or ingress of water, which would be critical for a buoyancy device. In particular, there is no evidence of any adaptations that would reduce or prevent leakiness, such as internal and external imperforate stereom layers. Instead, the lobolith walls are almost entirely composed of coarse and open labyrinthic stereom, which locally (in the distal plates) extends into microperforate stereom layers, remarkably similar to those observed in fossil and living benthic crinoids (e.g., Simms Reference Simms2001). Lack of imperforate stereom layers, both within the outer and inner lobolith walls, indicates that any perforation of the epidermis/dermis due to wave action, solar radiation, epibionts, and/or pathogens (a process commonly recorded in Recent echinoderms; see, e.g., Jangoux Reference Jangoux1984; Scheibling Reference Scheibling1984; Bauer and Young Reference Bauer and Young2000) would result in a gas leak, ingress of water, and loss of any positive buoyance. Likewise, we found no microscale adaptation that might increase buoyancy (such as skeletal lightening and hollow microarchitecture). Additionally, it appears that ossicles forming plate loboliths, and even more so cirrus loboliths, are not tightly packed: occasionally, open spaces in the plate or cirri network are visible (e.g., Figs. 2J and 3G,H; see also Haude Reference Haude1972: Fig. 11E).
Haude (Reference Haude1992), as part of his argument, suggested a pathway of scyphocrinoid evolution. According to his scenario, scyphocrinoid ancestors had an open cirrus network and were rooted in a muddy sediment. Subsequently, their descendants evolved tight chambers within the cirrus network, enabling floating, and these were eventually transformed into large chambers of advanced plate loboliths. However, this evolutionary scenario is not supported by available data: as noted by Hess (Reference Hess2010: p. 5) “this sequence, starting from a crinoid rooted at the bottom and ending as a pelagic lobolith, is purely conjectural.” Furthermore, the scenario is inconsistent with stratigraphic occurrence of scyphocrinoid loboliths, as noted by Prokop and Petr (Reference Prokop and Petr2001: p. 260): “there is no evidence supporting the speculation that stratigraphically lower levels yielded cirrus loboliths…. while higher ones … yielded plate loboliths.” In fact, there is some evidence that both lobolith types evolved independently from different lineages within scyphocrinitids and marhoumacrinids (Prokop and Petr Reference Prokop and Petr1992).
Supposed Adaptations to Tow-Net Filtration.—A pseudo-planktonic mode of life is widely accepted for two Mesozoic crinoids preserved intimately associated with large driftwood, Seirocrinus and Traumatocrinus. These crinoids possess certain morphologic features thought to have been critical to the “tow-net filtrator” function (Seilacher and Hauff Reference Seilacher and Hauff2004). For example, the length of Seirocrinus and Traumatocrinus stalks is exceptional, reaching 20 and 11 m, respectively (e.g., Simms Reference Simms1986; Hagdorn et al. Reference Hagdorn, Wang and Wang2007). With increasing stalk length, it would have been possible for the proximal, crown-bearing end and the distal, driftwood end of these crinoids to occupy fluids with different flow regimes and nutrient concentrations. Additionally, these crinoids would have had very large “tow nets,” given their long arms with many endotomous branches resulting in as many as 600 arm tips (Seilacher and Hauff Reference Seilacher and Hauff2004). In contrast, scyphocrinoid stalk length (up to ~3 m) is much shorter, and its arms branch isotomously, resulting in a filtration fan having ~160 arm tips (Seilacher and Hauff Reference Seilacher and Hauff2004). Unlike the morphology of the stalk and arms of Seirocrinus and Traumatocrinus, the scyphocrinoid stalk and arms are typical of many benthic crinoids, both fossil, such as Acanthocrinus, Aryballocrinus, Glyptocrinus, and Ptychocrinus (Ubaghs Reference Ubaghs, Ubaghs, Moore and Teichert1978a), and living, such as Endoxocrinus and Cenocrinus (Macurda and Meyer Reference Macurda and Meyer1974; Messing et al. Reference Messing, Neumann and Lang1990, Reference Messing, David, Roux, Améziane and Baumiller2007).
All of this suggests that compared with benthic crinoids, scyphocrinoid morphology was not that unusual, whereas it differed substantively from taxa generally thought to be planktonic/pseudoplanktonic. Furthermore, the biomechanical modeling calculations indicate that scyphocrinoids were not particularly well designed for tow-net filtration. Even when operating in high currents, the small size of the loboliths and their subspherical shape resulted in a drag force that could produce only a small relative velocity at the filter, roughly 10% of the current velocity, even assuming generously that the filtration fan was dragged through still water. The latter would require an unrealistically steep velocity gradient: 100% over the 3 m length of the stalk. From this perspective, the much longer stalks of Seirocrinus and Traumatocrinus and the fact they were towed by a “float” with a much larger surface area (driftwood), and thus drag, would greatly enhance their tow-net filtration properties. An analogy with a trawling method of fishing may help clarify the problem of scyphocrinoid as a tow-net filtrator: relative to Seirocrinus and Traumatocrinus, the scyphocrinoid crown would have been pulled through the water by an underpowered motor (small lobolith). Remarkably, loboliths have no specific adaptations in shape that would greatly enhance their drag (such as cubic or flat plate morphology).
Although the current biomechanical model does not include an analysis of particle capture rates or metabolic gains, as that would require modeling based on poorly constrained data on particle concentrations and size distributions, relative velocity is a parameter of first-order importance for such an analysis. So, while the results of the model do not preclude the possibility of scyphocrinoids as tow-net filtrators, they do suggest that simply “flipping” the model to the standard sessile stalked crinoid posture (lobolith attached to bottom with filtration fan up) allows the filter to take full advantage of the ambient flow while keeping the lobolith safely in the boundary layer.
Hess (Reference Hess2010) speculated that floating scyphocrinoids might have been able to filter zooplankton, relying on the latter's diurnal vertical migration. However, vertical migration speeds of zooplankton are typically very low (up to about few centimeters per second; see, e.g., Heywood Reference Heywood1996; Richards et al. Reference Richards, Possingham and Noye1996). Given the inconstant nature and low velocity of vertical migration of zooplankton (as compared with more stable and constant flow conditions and higher current velocities in the habitats of living benthic crinoids; see, e.g., Macurda and Meyer Reference Macurda and Meyer1974; Roux Reference Roux1980; Messing et al. Reference Messing, Neumann and Lang1990; Baumiller and Messing Reference Baumiller and Messing2007), this mode of feeding of scyphocrinoids would have been cost-ineffective and is thus also highly improbable.
Lobolith Development and Supposed Mechanisms of Gas Generation and Its Regulation.—Perhaps the most controversial aspects of the planktonic scyphocrinoid hypothesis relate to lobolith development, mechanisms of gas generation in loboliths, and mechanisms of buoyancy adjustment. Within collars of plate loboliths, small roots of crinoids are commonly present (e.g., Haude Reference Haude1992; Prokop and Petr Reference Prokop and Petr2001). It has been argued that these small roots represent either juvenile scyphocrinoids (Springer Reference Springer1917; Prokop and Petr Reference Prokop and Petr2001) or sexual dimorphs of this taxon (Seilacher personal communication in Haude Reference Haude1972). The latter would be unique among crinoids (Hess Reference Hess2010). Strimple (Reference Strimple1963) speculated that larvae of scyphocrinoids attached with roots to well-developed loboliths of mature specimens and then formed their loboliths after breaking off from the mature specimens. However, he failed to provide a mechanism. Others have suggested that juvenile scyphocrinoids were attached by their root cirri to algae drifting at the water's surface (Haude Reference Haude1992, Reference Haude1998) and that gas might have accumulated by diffusion from the surrounding water (Haude Reference Haude1972). Prokop and Petr (Reference Prokop and Petr1992) and Haude et al. (Reference Haude, Corriga, Corradini, Walliser, Wiese, Reich and Arp2014) speculated that juvenile scyphocrinoids were attached to mature scyphocrinoid loboliths and entrapped epipelagic photosynthetic algae during lobolith development. However, as noted by Hess (Reference Hess2010: p. 5): “It is hardly conceivable that a cirriferous radix attached to a larger lobolith could somehow turn into an independent lobolith, and no intermediate forms are known.”
The presence of encrusting organisms on mature loboliths, including juvenile scyphocrinoids, platyceratid gastropods, and bryozoans (e.g., Springer Reference Springer1917; Haude Reference Haude1972; Hess Reference Hess, Hess, Ausich, Brett and Simms1999), is noteworthy, because being “weighty,” they would have certainly affected the buoyancy of the adult scyphocrinoid “host.” These weighty encrusters imply that scyphocrinoids either had lots of excess buoyancy and thus floated on the surface or needed to evolve an active buoyancy compensation mechanism.
If scyphocrinoids were capable of being buoyed by loboliths, one of the scenarios assumes that the loboliths drifted passively at the water surface (Haude Reference Haude1992, Reference Haude1998). However, surface-floating loboliths would have been exposed to destructive solar radiation, wind, high-energy wave action, and storm events. Haude et al. (Reference Haude, Corriga, Corradini, Walliser, Wiese, Reich and Arp2014) suggested that loboliths might have been protected against sun, wind, and wave energy by forming a distal wall strengthened with pustules (e.g., Haude Reference Haude1972: Fig. 11d, Reference Haude1998: Fig. 4c). He also speculated that the distal sides of loboliths might have been encrusted by protective algae or bacteria absorbing solar radiation and that the outermost distal wall actually might have been free of stroma. However, evidence supporting any of this is entirely lacking. Furthermore, if the distal wall was free of stroma, a gas leak would have been even more likely (due to lack of imperforate stereom layers). Noteworthy, waves are expected to put stress also on the stalk as the lobolith is bounced up and down. Seilacher and Hauff (Reference Seilacher and Hauff2004) attempted to solve the problems associated with a surface-floating loboliths by assuming that scyphocrinoids actively adjusted their buoyancy and lived below the surface. They speculated: “As a stiff capsule, the lobolith was unsuited for Cartesian diving, but its aperture made it possible to control the gas content so that buoyancy could be actively adjusted to a certain depth, as in a swim bladder” (pp. 8–9). More recently, however, Seilacher and Gishlick (Reference Seilacher and Gishlick2014) advocated a Cartesian diver buoyancy compensation mechanism for these crinoids without providing any evidence. The possibility of active adjustment of buoyancy to a certain depth was refuted by Haude et al. (Reference Haude, Corriga, Corradini, Walliser, Wiese, Reich and Arp2014), because: (1) encrusting organisms are almost exclusively present on the proximal side of loboliths; and (2) this would require the evolution of a highly sophisticated and physiologically regulated mechanism for changing gas volume.
Taphonomy and Distribution.—Evidence from taphonomy has been commonly invoked in support of the planktonic lifestyle of scyphocrinoids. Specifically, it has been pointed out that loboliths may occur in different facies and are commonly not associated with scyphocrinoid crowns (e.g., Hess Reference Hess, Hess, Ausich, Brett and Simms1999). One explanation that has been offered for this pattern is that stalks of scyphocrinoids were easily broken off near the loboliths during storms, allowing nekroplanktonic dispersal of loboliths over long distances. Subsequently, waterlogged loboliths sank to the bottom, with their proximal part (with the collar) upward, like short-necked, water-filled bottles (Hess Reference Hess2010).
However, there are several problems related to this taphonomic scenario. First, scyphocrinoids commonly occur in discrete lenses (Hess Reference Hess, Hess, Ausich, Brett and Simms1999). As stated by Hess (Reference Hess, Hess, Ausich, Brett and Simms1999: p. 102): “A storm would not normally deposit floating animals neatly into lenses that thin out into clays or shales.” Second, loboliths cannot be directly compared with the short-necked bottles. Following the crinoid's death and stalk detachment, rapid decay of soft tissues is expected to occur within the lobolith. Thus, ingress of water and gas leakage would have occurred throughout labyrinthic and micorperforate stereom layers, including between plate boundaries, rather than only through the proximal canals within the collar. It is unlikely that this would have led to deposition of floating loboliths with their proximal parts preferentially oriented upward (see Springer Reference Springer1917; Ray Reference Ray1980). Third, close associations of loboliths and crowns are well known from many sites in the United States and Morocco (e.g., Springer Reference Springer1917; Haude et al. Reference Haude, Corriga, Corradini, Walliser, Wiese, Reich and Arp2014). Notably, a single, nearly complete juvenile specimen still attached to a lobolith has been reported (Hess Reference Hess, Hess, Ausich, Brett and Simms1999).
The dissociation of crowns and holdfasts of scyphocrinoids should not be viewed as unusual, as it is well known in benthic crinoids (e.g., Plotnick et al. Reference Plotnick, Ebey and Zinga2016). The reason for such a dissociation is that these two distinct body parts are affected differentially by biostratinomic processes (e.g., Brett Reference Brett1981; Brett et al. Reference Brett, Moffat, Taylor, Waters and Maples1997). One famous example is the Ordovician Cabo Busto (Spain) lag deposit containing thousands of holdfasts of the benthic Oryctoconus but hardly any other crinoid ossicles (Seilacher and MacClintock Reference Seilacher and MacClintock2005). In the same way, the large size and bulbous shape of loboliths would have given them hydrodynamic characteristics distinct from those of the stem and crown, such that differential transport following stem detachment would have been likely. Also, one cannot exclude nekroplanktonic dispersal of loboliths, as this process is also known in Recent benthic echinoderms, including echinoids (Reyment Reference Reyment1986). Admittedly, however, this commonly occurs due to the dessication of echinoids through subaerial exposure, which results when they get swept onshore by storm/wave action, a process unlikely to affect those scyphocrinoids that lived below storm wave base.
A critical argument for the planktonic mode of life of scyphocrinoids has been the wide geographic distribution of loboliths. However, when examined at the appropriate taxonomic scale, namely at the species level, the argument for their wide distribution fails. As pointed out by Petr and Prokop (Reference Prokop and Petr2001): “there is no evidence of ‘cosmopolitan distribution’ of particular species (Prokop and Petr Reference Prokop and Petr1987) … the fossil evidence points rather to more or less restricted colonies of different species or even genera.” Moreover, wide geographic distributions are common in benthic crinoids such as the Recent Atelecrinus, Comissia, Poliometra, Pentametrocrinus, and Thaumatocrinus (see Hess and Messing Reference Hess, Messing and Selden2011) and fossil uintacrinoids (see Milsom et al. Reference Milsom, Simms and Gale1994; Gorzelak et al. Reference Gorzelak, Głuchowski, Brachaniec, Łukowiak and Salamon2017), not because they are planktonic as adults, but rather because their larvae had high dispersal capabilities.
Finally, some of the encrusters of scyphocrinoids argue against their planktonic life mode. For instance, epibenthic platyceratid snails, known to infest benthic invertebrates (mostly crinoids; see, e.g., Gahn and Baumiller Reference Gahn and Baumiller2003) are also associated with scyphocrinoids (Haude Reference Haude1992: Plate 1; Hess Reference Hess, Hess, Ausich, Brett and Simms1999: Fig. 110). Although, the vertical migration of platyceratid planktotrophic larvae to the water's surface is a possibility, to our knowledge no examples of syn-vivo platyceratid infestation of other definitively nektonic invertebrates are known (Horný Reference Horný2000).
Proposed Mode of Life.—Taken together, morphologic, taphonomic, and theoretical arguments suggest that scyphocrinoids were benthic. Interestingly, Springer (Reference Springer1917: p. 9), in his generally overlooked monograph on this enigmatic group of crinoids, raised doubts about loboliths as buoys: “The theory that they served as a float loses much of its force. That supposition, always at best a somewhat forced one, is no longer necessary, since the upright position of the bulbs [loboliths] is perfectly consistent with the simpler and more natural idea that they served merely as enlarged roots, by which the crinoids were permanently or temporarily fixed to the sea bottom.”
Our micromorphologic data support the view that the lobolith served as a specialized holdfast. Its bulbous and hollow structure, along with irregular wavy ridges, keels, and spines (observed mostly on the distal, slightly flattened side of lobolith), might have prevented crinoids from dislodgement and from sinking deeply into unconsolidated substrate. Similar “iceberg” and “snowshoe” strategies are present in some Recent mollusk and brachiopod species living on muddy bottoms (Thayer Reference Thayer1975). The fact that chambers of loboliths are frequently filled (at least partly) with fine sediments matching external sediment lithology may be consistent with this interpretation (e.g., Ray Reference Ray1980; Lee Reference Lee2005; Huber and Gibson Reference Huber and Gibson2007; Haude et al. Reference Haude, Corriga, Corradini, Walliser, Wiese, Reich and Arp2014; Donovan and Miller Reference Donovan and Miller2014; our data, Fig. 4). The question as to whether all chambers were open or closed during crinoid life remains unanswered. Hess (Reference Hess, Hess, Ausich, Brett and Simms1999) argued that larger chambers around the base of the stem were probably open, because they are commonly infilled by a sediment (Haude Reference Haude1992). The other chambers might have been closed and filled by coelomic or perihaemal fluid, as inferred from the local presence of sparry calcite. Admittedly, data from internal infillings are inconclusive, because similar types of infillings commonly characterize invertebrates displaying hollow internal structures/cavities (e.g., brachiopods, echinoids, and mollusks) irrespective of their benthic or nektonic/planktonic mode of life (e.g., Wieczorek Reference Wieczorek1979; Zamora et al. Reference Zamora, Mayoral, Vintaned, Bajo and Espílez2008). However, as stressed by Ray (Reference Ray1980: p. 15) “The stems’ upward orientation in the outcrops…, the geopetal structures that indicate an upward-oriented majority…, and the unsorted sizes of bulbs [loboliths] found in the outcrops, unlike sorted floating organisms pocketed together after death, are several facts that are consistent with the ‘anchoring root’ conclusion.”
During their evolutionary history, and especially during the Paleozoic, many crinoids developed a variety of structures at their distal stems identified as adaptations for living on soft substrates (Seilacher and MacClintock Reference Seilacher and MacClintock2005; Plotnick et al. Reference Plotnick, Ebey and Zinga2016). In our view, loboliths represent yet another example of such a structure. Development of such a hollow structure allowed a crinoid to form a large holdfast without having to secrete massive quantities of calcite. Springer (Reference Springer1917) noted the analogue between loboliths and holdfasts of living alcyonarian polyps (pennatulids). Another analogous bulbous holdfast characterizes the Recent algae Saccorhiza, in which a stalk may reach up to about 5 m in length (e.g., McKenzie and Moore Reference McKenzie and Moore1981).
An important question that needs to be addressed concerns the feeding posture of scyphocrinoids. Maintaining a nearly vertical 3-m-long stalk with a lobolith on the substrate (Fig. 8) would have been challenging, because a slight displacement of the center of mass from the stalk's long axis would have been difficult to counter and would likely have resulted in the crinoid tipping over. The stability problem would have been even more severe with drag exerted on the crown, as that would produce a large moment (drag × stalk length). One solution is that scyphocrinoids, like Recent isocrinids (Baumiller Reference Baumiller2008), extended the distal portion of the stalk along the bottom for stability (Figs. 9A,B and 10). In soft substrates, the mass of the stalk and lobolith along the bottom would also act as a counterweight to drag and the weight of the crown, reducing the risk of tipping over. Maintenance of such a feeding posture would have been even more effective if the lobolith was positioned downstream (Fig. 9B). In this scenario, depending on the drag, the horizontal to vertical stalk length ratio might have been actively adjusted with the aid of mutable collagenous tissues (with high drag leading to more of the distal stalk along bottom). In this context, the horizontal part of the string-like stalk could have also helped prevent sinking and, together with lobolith, might have additionally acted as a drag anchor (see Plotnick and Bauer [Reference Plotnick, Bauer, Hembree, Platt and Smith2014] for a discussion of biological anchors). The free-lying anchors of scyphocrinoids may have been advantageous during crinoid dislodgement (e.g., induced by storms or anomalously high currents), because they would have remained attached to the long stem and could have been used again. Such drag anchors lying on the bottom might have allowed safe reimplantation of a crinoid in soft substrates without a risk of breaking off the stem (which typically leads to a crinoid death).

Figure 8. Reconstruction of scyphocrinoids as benthic crinoids with nearly vertical stalks.

Figure 9. Reconstructions of scyphocrinoids as benthic crinoids with the stalks partly extending along the bottom and loboliths facing upstream (A) or downstream (B).
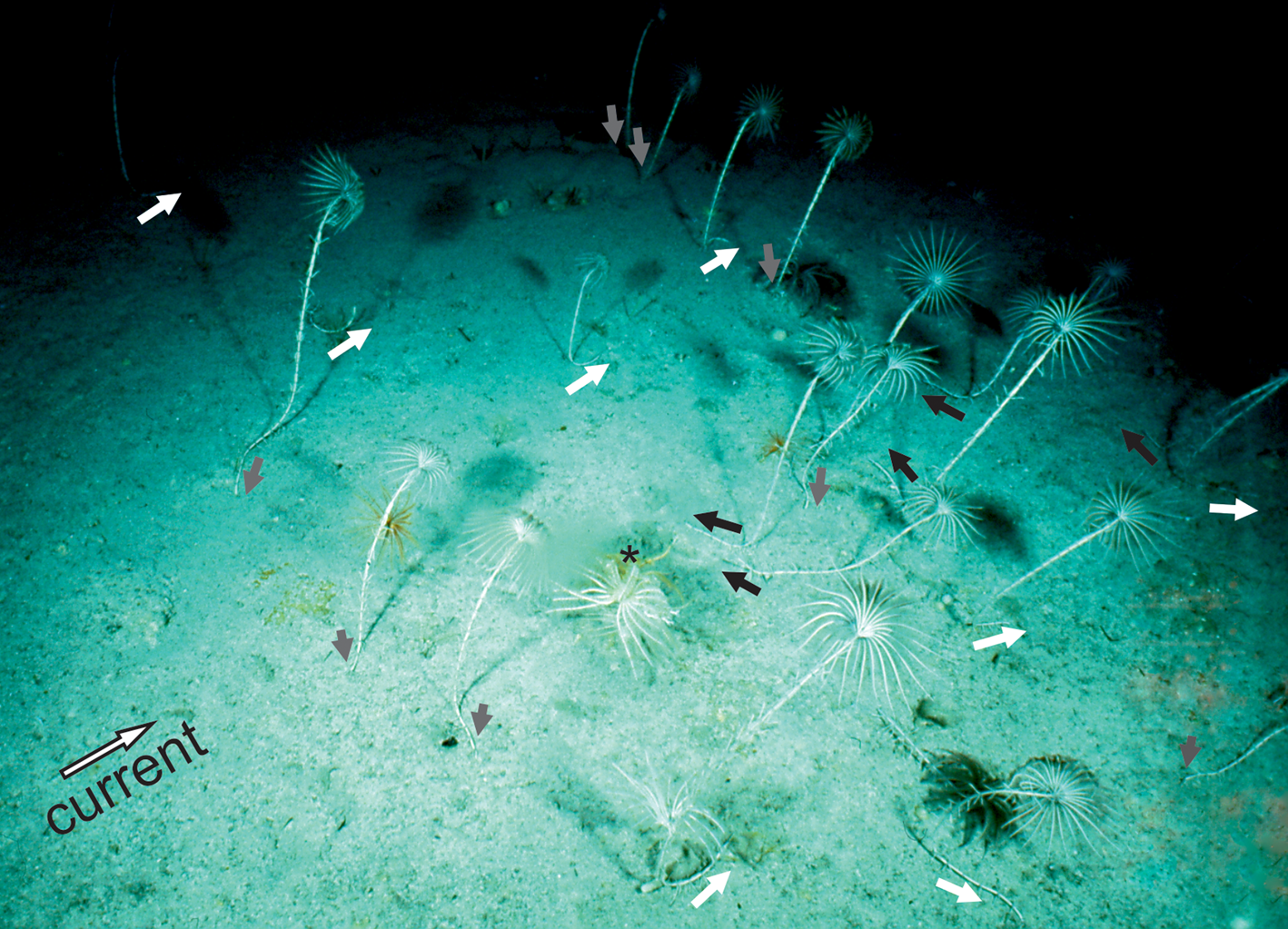
Figure 10. The living isocrinids Neocrinus decorus in a feeding posture at a depth of 424–430 m south of West End, Grand Bahama Island (26°36.8′N, 78°58.6′W). The water current direction is indicated by the large white arrow. Note that distal portions of isocrinid stalks are extended along the bottom, facing downstream (or oblique-downstream; white arrows) or upstream (or oblique-upstream; black arrows); in some specimens, distal portions of stalks have been buried with fine sediment (gray arrows); the asterisk marks a specimen that is crawling. Photo courtesy of C. G. Messing.
Even with a partly recumbent feeding posture, scyphocrinoids, with a stem up to ~3 m long, could have easily achieved the highest epifaunal tier of Paleozoic crinoids (Ausich and Bottjer Reference Ausich and Bottjer1982). Development of such a long stem would have been advantageous not only for feeding, as it elevated the food-gathering crown well above the seafloor into faster currents (Ausich Reference Ausich1980; Ausich and Bottjer Reference Ausich and Bottjer1982), but also for “escaping” the bottom when unfavorable (e.g., low-oxygen) conditions became prevalent.
Conclusions
As rightly noted by Seilacher and Hauff (Reference Seilacher and Gishlick2014: p. 14): “For sessile benthic organisms, becoming pelagic is an evolutionary step comparable to the acquisition of flight in land animals and likewise requires ecological steppingstones.” The hypothesis that scyphocrinoids may have been planktonic, which held sway for more than 100 years, ignores many taphonomic and micro-and macro-anatomical features of these crinoids. Furthermore, we demonstrate that scyphocrinoids would have been poorly suited for a pelagic, tow-net lifestyle. Several lines of evidence suggest that they were benthic and that their loboliths served as modified holdfasts for anchoring on unconsolidated bottoms. So whereas we find no evidence for the “evolutionary step” envisioned by Seilacher and Hauff (Reference Seilacher and Gishlick2014) in scyphocrinoids, this crinoid group still remains unique: with a 3 m stem elevating the crown well above the bottom, they would have occupied the highest epifaunal tier in the Paleozoic.
Acknowledgments
Parts of this study were conducted in the NanoFun laboratory cofinanced by the European Regional Development Fund within the Innovation Economy Operational Programme POIG.02.02.00–00-025/09. We thank Carol Abraczinskas, who helped with Figures 5, 6, and 7, two anonymous reviewers for helpful comments and suggestions, and Paul Selden for permission to reproduce Fig. 1B,C. This paper was completed while M.A.S. was a recipient of a grant from the National Science Centre (grant number UMO-2018/31/B/ST10/00387).