Introduction
Hagstromite is the 16th new mineral to be described from the remarkable Pb–Cu–Te-rich secondary mineral assemblage at Otto Mountain, near Baker, California, USA (Kampf et al., Reference Kampf, Housley, Mills, Marty and Thorne2010a; Housley et al., Reference Housley, Kampf, Mills, Marty and Thorne2011). A complete list of secondary Te oxysalts from Otto Mountain up-to-date through 2016 is provided by Christy et al. (Reference Christy, Mills, Kampf, Housley, Thorne and Marty2016). Among the new Otto Mountain mineral species, only hagstromite and the recently described müllerite, Pb2Fe3+(Te6+O6)Cl (Mills et al., Reference Mills, Kampf, Momma, Housley and Marty2020), are not included in that list.
The mineral is named for John P. Hagstrom (b. 1953) of Las Vegas, Nevada, USA. A mineral collector since the age of 17, Mr. Hagstrom has in recent years focused on the mineral deposits in the Goodsprings district, Nevada, and the Blue Bell mine and Otto Mountain near Baker, California. He has provided many of his collected specimens for scientific study, especially those from Otto Mountain. Mr. Hagstrom collected one of only two known samples of hagstromite and provided the best portion of this specimen, which now constitutes the holotype for the species. Mr. Hagstrom has given his permission for the mineral to be named in his honour.
The new mineral and name have been approved by the Commission on New Minerals, Nomenclature and Classification of the International Mineralogical Association (IMA2019-093, Kampf et al., Reference Kampf, Housley, Mills, Rossman and Marty2020). One holotype and one cotype specimen are deposited in the Natural History Museum of Los Angeles County under catalogue numbers 73596 and 73597, respectively.
Occurrence
Hagstromite was found at sites on Otto Mountain, 2.5 km WNW of Baker, San Bernardino County, California, USA. It was found by one of the authors (JM) on a single specimen (cotype) collected from the E3 Vein (3rd quartz vein east of the Bird Nest drift) located at (35.276078°N, 116.099722°W, and it was found on a single specimen collected by John Hagstrom at the SW Cut (on the southwest side of Otto Mountain) located at 35.27222°N, 116.10116°W. Note that the latter specimen was broken into several pieces, only one of which is deposited as the holotype.
Hagstromite is very rare and has only been found on the two small samples, noted above. Crystals occur on recrystallised quartz fracture surfaces. On the holotype it is associated with cerussite, fuettererite (Kampf et al., Reference Kampf, Mills, Housley and Marty2013a) and thorneite (Kampf et al., Reference Kampf, Housley and Marty2010b). On the cotype, it is associated with caledonite, diaboleite and timroseite (Kampf et al., Reference Kampf, Mills, Housley, Marty and Thorne2010c). Hagstromite is a secondary oxidation zone mineral and is presumed to have formed by oxidation of earlier formed tellurides (most likely hessite), chalcopyrite and galena. Additional background on the occurrence is provided in Kampf et al. (Reference Kampf, Housley, Mills, Marty and Thorne2010a), Housley et al. (Reference Housley, Kampf, Mills, Marty and Thorne2011) and Christy et al. (Reference Christy, Mills, Kampf, Housley, Thorne and Marty2016).
Physical and optical properties
Hagstromite occurs as light yellow–green blades, elongated on [001] and flattened on {100}, up to ~100 μm long (Figs 1 and 2). The crystals were too small and imperfectly formed to measure crystal forms; however, {100} is prominent and {110} and {001} are probably based upon the general shape and the Donnay–Harker law. No twinning was observed optically under crossed polars or on the basis of single-crystal X-ray diffraction results. The streak is very pale yellow–green. Crystals are transparent with adamantine lustre; crystal intergrowths have silky lustre. Hagstromite does not fluoresce under long- or short-wave ultraviolet light. The Mohs hardness could not be measured, but is probably between 2 and 3. The mineral is brittle with splintery fracture. There appear to be two cleavages, probably on {100} and {010}. The density could not be measured because it is greater than those of available high-density liquids and there is insufficient material for physical measurement. The calculated density is 7.062 g cm–3 for the empirical formula and 7.038 g cm–3 for the ideal formula, calculated from the single-crystal unit-cell parameters. Hagstromite crystals dissolve in dilute HCl at room temperature.

Fig. 1. Light yellow–green hagstromite blades with bright–green fuettererite, small yellow thorneite crystals and colourless cerussite crystals on quartz. Field of view: 1.14 mm across; holotype specimen #73596.

Fig. 2. Back-scatter SEM image of hagstromite (left) and thorneite (right). Fragment from holotype (#73596).
The Gladstone–Dale relationship (Mandarino, Reference Mandarino2007) predicts an average index of refraction of 2.071. The unavailability of index liquids with n > 2 precluded the measurement of the mineral's indexes of refraction. The γ–α birefringence (0.04) was measured using a Berek compensator and 2V was measured using extinction data analysed with EXCALIBRW (Gunter et al., Reference Gunter, Bandli, Bloss, Evans, Su and Weaver2004). This allowed the calculation of the indices of refraction. Hagstromite is optically biaxial (+), with α = 2.045(calc), β = 2.066(calc), γ = 2.102(calc) and 2Vmeas = 76(1)°. Dispersion could not be observed. The optical orientation is X = b, Y = a and Z = c. No pleochroism was observed.
Raman spectroscopy
Besides hagstromite, there are only two tellurate minerals that also contain essential carbonate: agaite, Pb3Cu2+Te6+O5(OH)2(CO3) (Kampf et al., Reference Kampf, Mills, Housley and Marty2013b) and thorneite, Pb6(Te6+2O10)(CO3)Cl2(H2O) (Kampf et al., Reference Kampf, Housley and Marty2010b). Raman spectra for all three minerals were recorded on a Horiba XploRA PLUS spectrometer with a 532 nm diode laser. The laser was focused onto the samples with a 100× objective lens and the spectrum was recorded with a 1800 gr/mm diffraction grating. The full spectrum for hagstromite from 3900 to 50 cm–1 and the spectra for all three minerals from 1500 to 50 cm–1 are shown in Fig. 3.

Fig. 3. The Raman spectra for hagstromite, agaite and thorneite recorded with a 532 nm laser.
The sharp bands at 1041 cm–1 (hagstromite), 1038 cm–1 (agaite) and 1056 cm–1 (thorneite) are in the shift range where the strongest carbonate band typically occurs. Tellurates have been shown previously to have the components of their Te–O stretching modes in the 850 to 600 cm–1 region (Blasse and Hordijk, Reference Blasse and Hordijk1972; Frost, Reference Frost2009; Frost and Keeffe, Reference Frost and Keeffe2009; Kampf et al., Reference Kampf, Mills, Housley and Marty2013b; Frikha et al., Reference Frikha, Abdelhedi, Dammak and Garcia-Granda2017; Missen et al., Reference Missen, Kampf, Mills, Housley, Spratt, Welch, Coolbaugh, Marty, Chorazewicz and Ferraris2019). Of the several hagstromite spectra recorded on different crystals in random orientations, all but one were featureless between 3900 and 1700 cm–1. The one exception, which is shown in Fig. 3, shows a low, broad hump between ~3500 and 2800 cm–1 in the OH stretching region, which may indicate a small amount of disordered OH in the structure. This feature is consistent with moderately strongly hydrogen-bonded OH, at least stronger than is associated with H2O and OH in many minerals, which may suggest that the carbonate group in hagstromite can have some bicarbonate character.
Chemical composition
Electron probe microanalysis (EPMA) of hagstromite was carried out on five samples using a JEOL 8200 at the Division of Geological and Planetary Sciences, California Institute of Technology. The wavelength dispersive spectroscopy (WDS) mode was used with 15 kV, 5 nA and a focused beam. The small fragile crystals embedded in epoxy could not be polished as they fragmented even when subjected to gentle abrasion. Analyses were eventually done on unpolished crystal surfaces. The possibility of Cl migration was specifically examined, but was found not to be a factor. No beam damage was observed; the somewhat low total is attributed to the somewhat irregular surfaces provided by even the flattest crystal faces. Analyses for N, Sb, S, Ca, Zn and As showed these elements to be below the limits of detection. No other elements were detected by energy dispersive spectroscopy (EDS) on a Hitachi S–3000N scanning electron microscope (SEM) using an Oxford SEMEDX200 with INCA software and internal standards. Raman spectroscopy and crystal structure analysis indicated the presence of carbonate and one out of several spectra recorded indicated the possibility of minor OH. There was insufficient material for CHN analyses, so CO2 was calculated on the basis of stoichiometry (Cl + C = 5 atoms per formula unit (apfu)) as indicated by the crystal structure. The analytical results are given in Table 1. The empirical formula (based on 11 Pb + Cu + Te apfu) is Pb8.07Cu2+0.98Te6+1.96C1.17Cl3.83O15.34. The ideal formula is Pb8Cu2+(Te6+O6)2(CO3)Cl4, which requires PbO 75.34, CuO 3.36, TeO3 14.82, CO2 1.86, Cl 5.98, O = Cl –1.35, total 100 wt.%.
Table 1. Electron microprobe analytical data (in wt.%) for hagstromite.

*Based on stoichiometry (Cl + C = 5 apfu)
S.D. – standard deviation
Using ideal cation contents, an excess in CO3 and a deficiency in Cl relative to the ideal formula Pb8Cu2+(Te6+O6)2(CO3)Cl4, such as for the formula Pb8Cu2+(Te6+O6)2(CO3)1.2Cl3.8, results in a net negative charge (–0.2 for the example given). It is possible that this could be balanced by adding a small amount of H, perhaps associated with the O sites belonging to the carbonate group, giving this group a partial bicarbonate (HCO3–) character. Two observations support this possibility: (1) one Raman spectrum we recorded reveals a weak broad band in the OH stretching region; and (2) in the refinement of the structure (see below), when the C–O bond lengths are not restrained, they become somewhat long for a normal CO3 group; however, we do not consider either of these as conclusive evidence for the presence of H. A similar situation was observed in the structure of favreauite (Mills et al., Reference Mills, Kampf, Christy, Housley, Thorne, Chen and Steele2014). Another possible mechanism for charge balance is the replacement of a small amount of C4+ by N5+, i.e. replace some CO32– by NO3–. To test this, we ran several EPMA WDS scans for N using GaN as the standard. No N was detected. It is also worth noting that no N–bearing phases have been found in the Otto Mountain mineral assemblages. We are, therefore, confident that there is no significant NO3 in hagstromite.
X–ray crystallography and structure determination
Powder X–ray diffraction data were obtained on a Rigaku R–Axis Rapid II curved imaging plate microdiffractometer utilising monochromatised MoKα radiation. Observed powder d values and intensities were derived by profile fitting using JADE 2010 software. The observed powder data fit well with the calculated from the structure, also using JADE 2010 (Table 2). The unit-cell parameters refined from the powder data using JADE 2010 with whole–pattern fitting are: a = 23.699(4), b = 9.0487(16), c = 10.4693(15) Å and V = 2245.1(6) Å3.
Table 2. X-ray powder diffraction data (d in Å) for hagstromite. Only calculated lines with I > 1 are listed.

The strongest lines are in bold
Single-crystal X-ray studies were carried out using the same diffractometer and radiation noted above. Crystals of hagstromite are small and grow in subparallel intergrowths. Even the best crystals exhibit very high mosaicity. Due to diminishing intensity and broadening reflections, the crystal used for the single-crystal data collection provided usable data only to 1.18 Å. Note that attempts to collect data at the MX2 beamline of the Australian Synchrotron on a Dectris EigerX 16M detector and at the University of Manitoba using a Bruker D8 three-circle diffractometer equipped with a rotating anode generator (MoKα X-radiation), multilayer optics and an APEX–II CCD area detector were unsuccessful due to the poor crystal quality.
The Rigaku CrystalClear software package was used for processing the structure data, including the application of an empirical absorption correction using the multi–scan method with ABSCOR (Higashi, Reference Higashi2001). The structure was solved by the charge–flipping method using SHELXT (Sheldrick, Reference Sheldrick2015a). Refinement proceeded by full-matrix least-squares on F 2 using SHELXL–2016 (Sheldrick, Reference Sheldrick2015b). Regardless of the relatively poor quality of the data and its limited extent, the refinement was generally well behaved; however, the sites associated with the CO3 group and the related Cl3 site, which co-occupies the C site, required special treatment, including soft restraints of 1.26(3) Å on the C–O distances (see below). Data collection and refinement details are given in Table 3, atom coordinates and displacement parameters in Table 4, selected bond distances in Table 5 and a bond-valence analysis in Table 6. The crystallographic information files have been deposited with the Principal Editor of Mineralogical Magazine and are available as Supplementary material (see below).
Table 3. Data collection and structure refinement details for hagstromite.
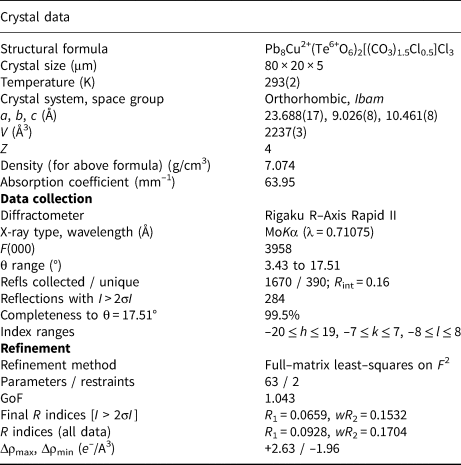
*R int = Σ|F o2–F o2(mean)|/Σ[F o2]. GoF = S = {Σ[w(F o2–F c2)2]/(n–p)}½. R 1 = Σ||F o|–|F c||/Σ|F o|. wR 2 = {Σ[w(F o2–F c2)2]/Σ[w(F o2)2]}½; w = 1/[σ2(F o2) + (aP)2 + bP] where a is 0.0863, b is 0 and P is [2F c2 + Max(F o2,0)]/3.
Table 4. Atom coordinates and displacement parameters (Å2) for hagstromite.

*The assigned occupancies are C/Cl3: C0.75Cl0.25, O1: 0.75, O2: 0.75.
Table 5. Selected bond lengths (Å) in hagstromite.

Note that occupancies assigned to the O1 (0.75), O2 (0.75) and Cl3 (0.25) sites have been factored in to the bond-length averages.
Table 6. Bond-valence sums for hagstromite. Values are expressed in valence units.

Pb2+–O bond-valence parameters are from Krivovichev (Reference Krivovichev2012). Cu2+–O and C4+–O are from Gagné and Hawthorne (Reference Gagné and Hawthorne2015). Te6+–O are from Mills and Christy (Reference Mills and Christy2013).
Description of the structure
The structure of hagstromite contains one Te6+ in octahedral six-fold coordination with O atoms, one Cu2+ in square-planar four-fold coordination with O atoms and four independent Pb2+ cations (Pb1, Pb2, Pb3 and Pb4) in off-centre 7½- and 8-fold coordinations with O and Cl atoms, typical of Pb2+ with stereoactive lone-pair electrons. There are two independent fully occupied Cl sites (Cl1 and Cl2).
An unusual feature of the structure is a partially occupied CO3 group that is co-occupied by Cl. This was initially indicated by a very low isotropic displacement parameter for the C site and less-than-full refined occupancies for the associated O sites of the CO3 group (O1 and O2). In addition, it was noted that the C site is surrounded by Pb sites in a coordination environment compatible with Cl at that site (Fig. 4; bond-valence sum = 0.87 valence units). The EPMA analyses consistently showed Cl contents close to 3.8 apfu, whereas the fully occupied Cl1 and Cl2 sites in the structure provide only 3 Cl apfu. The only region in the structure that can accommodate the additional Cl content is the vicinity of the CO3 group. A close match to the EPMA derived formula can be attained by assigning 0.6 occupancy to the CO3 group and 0.4 occupancy to Cl (Cl3) on the C site, which provides the formula Pb8Cu2+(Te6+O6)2(CO3)1.2Cl3.8; however, the refinement supports occupancies for these sites close to (CO3)0.75Cl0.25 corresponding to the formula Pb8Cu2+(Te6+O6)2(CO3)1.5Cl3.5. The relatively poor quality of the structure data and the possibility of additional unresolved Cl in the vicinity of the CO3 group sites leads us to regard the EPMA-derived Cl content to be more reliable.

Fig. 4. The structure of hagstromite viewed down [010]. Note that the square-planar CuO4 coordination (green) appears to be rectangular because it is oriented at ~45° to the direction of view (in alternating directions). Also, note that Cl3 is located at the centre of the CO3 triangle when the CO3 group sites are not occupied.
The structure of hagstromite is unique. Each of the four vertices of the CuO4 square is shared with a different TeO6 octahedron creating a novel Cu2+Te6+2O12 chain along c (Figs 4 and 5). The Pb atoms bond to all of the O atoms participating in the chain (O3, O4 and O5), to the two O atoms of the CO3 group (O1 and O2) and to the two Cl atoms (Cl1 and Cl2). When the CO3 group is replaced by Cl3, the Pb atoms bond to this Cl atom. The framework structure thereby created is shown in Figs 4 and 5.

Fig. 5. The structure of hagstromite viewed slightly canted down [001], the chain direction.
The only two other tellurate minerals containing essential carbonate are agaite, Pb3Cu2+Te6+O5(OH)2(CO3) and thorneite, Pb6(Te2O10)(CO3)Cl2(H2O), both of which also occur at Otto Mountain (and thorneite occurs in direct association with hagstromite). The structure of agaite (Kampf et al., Reference Kampf, Mills, Housley and Marty2013b) contains edge-sharing chains of Cu2+O5 square pyramids and Te6+O6 octahedra that are joined by corner-sharing to form polyhedral sheets; the thick interlayer region contains eight- and nine-coordinated Pb2+, as well as CO3 and OH groups. The structure of thorneite (Kampf et al., Reference Kampf, Housley and Marty2010b) contains edge-sharing octahedral tellurate dimers, [Te2O10]8– that bond to nine- and ten-coordinated Pb2+, which in turn are linked via bonds to Cl atoms, CO3 groups and H2O groups. In the structural classification of Te oxysalts of Christy et al. (Reference Christy, Mills, Kampf, Housley, Thorne and Marty2016), hagstromite falls into the category of structures with ‘monomeric Te6+X 6 as part of a larger structural unit that is an infinite chain’; however, the only chains composed of TeO6 octahedra and Cu2+ polyhedra (CuO4, CuO5 or CuO6) documented in that survey involve edge-sharing linkages.
Acknowledgements
Comments by anonymous reviewers are appreciated. Mark A. Cooper is thanked for his efforts to collect structure data at the University of Manitoba. Studies at Caltech were supported by the Northern California Mineralogical Association and by NSF grant EAR-1322082. Part of this study has been funded by The Ian Potter Foundation grant “tracking tellurium” to SJM. The remainder of this study was funded by the John Jago Trelawney Endowment to the Mineral Sciences Department of the Natural History Museum of Los Angeles County.
Supplementary material
To view supplementary material for this article, please visit https://doi.org/10.1180/mgm.2020.30