Introduction
Upper Mississippian (Serpukhovian, Chesterian) sedimentary rocks are widespread in northern Alabama across both the Appalachian Plateau and the Valley and Ridge province of the Appalachian orogenic belt (Thomas, Reference Thomas1972). In the northeastern portion of the state, these strata are dominated by carbonate rocks (Monteagle and Bangor limestones) that were deposited on a shallow marine platform adjacent to the Black Warrior foreland basin (Thomas and Mack, Reference Thomas and Mack2013). In our study area, east of Huntsville, Alabama (Fig. 1.1), a thin interval of brown-gray to dark gray shale containing interbeds of quartzose limestone and calcareous quartzarenite (Raymond et al., Reference Raymond, Osborne, Copeland and Neathery1988) separates the Monteagle and Bangor limestones (Figs. 1.2, 2). These rocks in northeastern Alabama are equivalent to the Hartselle Sandstone of northwestern Alabama and are difficult to distinguish lithologically from the Pride Mountain Formation. A diverse assortment of marine fossils is commonly observed in the thin limestones within the shaley unit. To the south and west of our study area, adjacent to the Black Warrior basin, the Hartselle Sandstone is a quartzarenite up to 50 m thick (Raymond et al., Reference Raymond, Osborne, Copeland and Neathery1988) that was deposited as a series of northwesterly oriented, shallow-marine-to-brackish barrier-island complexes (Thomas and Mack, Reference Thomas and Mack2013; Kopaska-Merkel and Rindsberg, Reference Kopaska-Merkel and Rindsberg2016; Fig. 1.1).

Figure 1. (1) Location of the study area in relation to surface exposure of Hartselle Sandstone in northern Alabama. Area of thickest outcrop is shown by the > 30 m isopach (modified from Kopaska-Merkel and Rindsberg, Reference Kopaska-Merkel and Rindsberg2016). Sites 1–4 refer to sections reported by Haywick et al. (Reference Haywick, Kopaska-Merkel and Keyes2016). Site 5 is a sponge-rich locality reported by Rigby and Keyes (Reference Rigby and Keyes1998). The Woodville mound is located at site 1. The location of the Moulton mound within the Bangor Limestone (Kopaska-Merkel and Haywick, Reference Kopaska-Merkel and Haywick2001) is also indicated on the main map. (2) Mississippian stratigraphic relationships in northern Alabama. Locations of known mounds indicated by dots. The Monteagle-Hartselle-equivalent interval targeted by this study is indicated by the small dotted box (modified from Keyes, Reference Keyes2018).

Figure 2. Schematic sedimentary section summarizing the stratigraphy and lithology of site 1 of Haywick et al. (Reference Haywick, Kopaska-Merkel and Keyes2016). The positions of small mounds and sampling locations are indicated.
Serpukhovian (Chesterian) organosedimentary structures (mounds and reefs) were comparatively rare in shallow marine regions surrounding the Black Warrior basin (Thomas, Reference Thomas1972; Andronaco, Reference Andronaco1986; Kopaska-Merkel and Haywick, Reference Kopaska-Merkel, Haywick, Puckett and Rindsberg2014), although they were common elsewhere (e.g., Pray, Reference Pray1961; Wilson, Reference Wilson and Wilson1975; Lees and Miller, Reference Lees, Miller, Monty, Bosence, Bridges and Pratt1995). Previous work by Kopaska-Merkel and Haywick (Reference Kopaska-Merkel and Haywick2001, Reference Kopaska-Merkel, Haywick, Puckett and Rindsberg2014) identified only 13 small buildups in the Bangor Limestone of northern Alabama and southeastern Tennessee. The largest, a coral-bryozoan-pelmatozoan-microbial biohermal complex near Moulton, Alabama (Fig. 1.1), is ~ 1.5 m thick and several tens of meters across. Smaller bioherms in the Bangor Limestone were built by various combinations of rugose corals, fenestrate and other bryozoans, pelmatozoans, and nonskeletal microbes (Kopaska-Merkel and Haywick, Reference Kopaska-Merkel, Haywick, Puckett and Rindsberg2014). Two other Mississippian mounds have been described from Alabama: (1) a bryozoan mud mound in the Pennington Formation (Gibson, Reference Gibson1986), and (2) a sponge-microbial mound in the Tuscumbia Limestone (Kopaska-Merkel et al., Reference Kopaska-Merkel, Mann, Pashin, Mancini, Morgan, Ahr, Parcell, Dias-Brito and Harris2013). More recently, investigations of the Monteagle-Hartselle interval in northeastern Alabama yielded three small carbonate mounds (Haywick et al., Reference Haywick, Kopaska-Merkel and Keyes2016; Fig. 2) near Woodville. Two are in the Monteagle Limestone; the other nucleated on an ooid grainstone in the lower part of the Hartselle-equivalent shale unit. This mound was buried by fissile, calcareous, gray shale. The shale contains sparse fossils; thin, very fossiliferous, rudstone/grainstone lenses, where they occur, consist primarily of fragmented fenestrate bryozoan fronds and axes of Archimedes Owen, Reference Owen1838, small crinoid ossicles, and brachiopods (Table 1). The coarser rock types (calcareous sandstone and sandy limestone noted by Raymond et al., Reference Raymond, Osborne, Copeland and Neathery1988) are generally well sorted, contain abundant coarse to very coarse (1–2 mm) skeletal detritus (in addition to the aforementioned, mollusks, sponges, and chondrichthyan teeth), subround-to-round dolomite clasts (1–6 mm), and fine to medium (0.25–0.5 mm), well-rounded quartz sand (Haywick et al., Reference Haywick, Kopaska-Merkel and Keyes2016).
Table 1. Macrofossils from the Hartselle-equivalent strata, site 1 and nearby sites. Macrofossil list from unpublished site records and data, site 40 (our site 1), R. Keyes, 2019.

All three mounds were recognized in the field by their characteristic domical shape. The Monteagle mounds are both ~ 0.7 m tall and 0.5 m wide at the base. The Hartselle-equivalent mound (Woodville mound) is slightly larger, ~ 1 m wide and 1.2 m tall (Figs. 2, 3). Three nearby outcrops of the same interval were carefully examined, and no additional mound-like structures were discovered (Haywick et al., Reference Haywick, Kopaska-Merkel and Keyes2016). The Monteagle mounds are composed of very finely crystalline dolomite, and no conclusive biogenic microstructures have been observed in outcrop. The Woodville mound is a construct of macroscopic skeletal elements dominated by the bryozoan Fistulipora M'Coy, Reference M'Coy1849 and fine carbonate sediment, bound together by a consortium of microscopic binding organisms. The mound is primarily medium gray in color but contains numerous limonite-stained mottles up to several centimeters wide. These are cavities that were filled with a poorly sorted mixture of siliciclastic and carbonate sediment and calcite cement. All three of the mounds at the study site are small, rather inconspicuous in outcrop, and might easily have been overlooked when their constituent organisms were alive. We nevertheless consider them mounds because they were constructed the same way as those organosedimentary structures, through a combination of biogenic and depositional processes. Although small, they can be quite revealing about paleoenvironmental conditions and contain fossil species that are either rare in associated rocks or overlooked in larger structures.
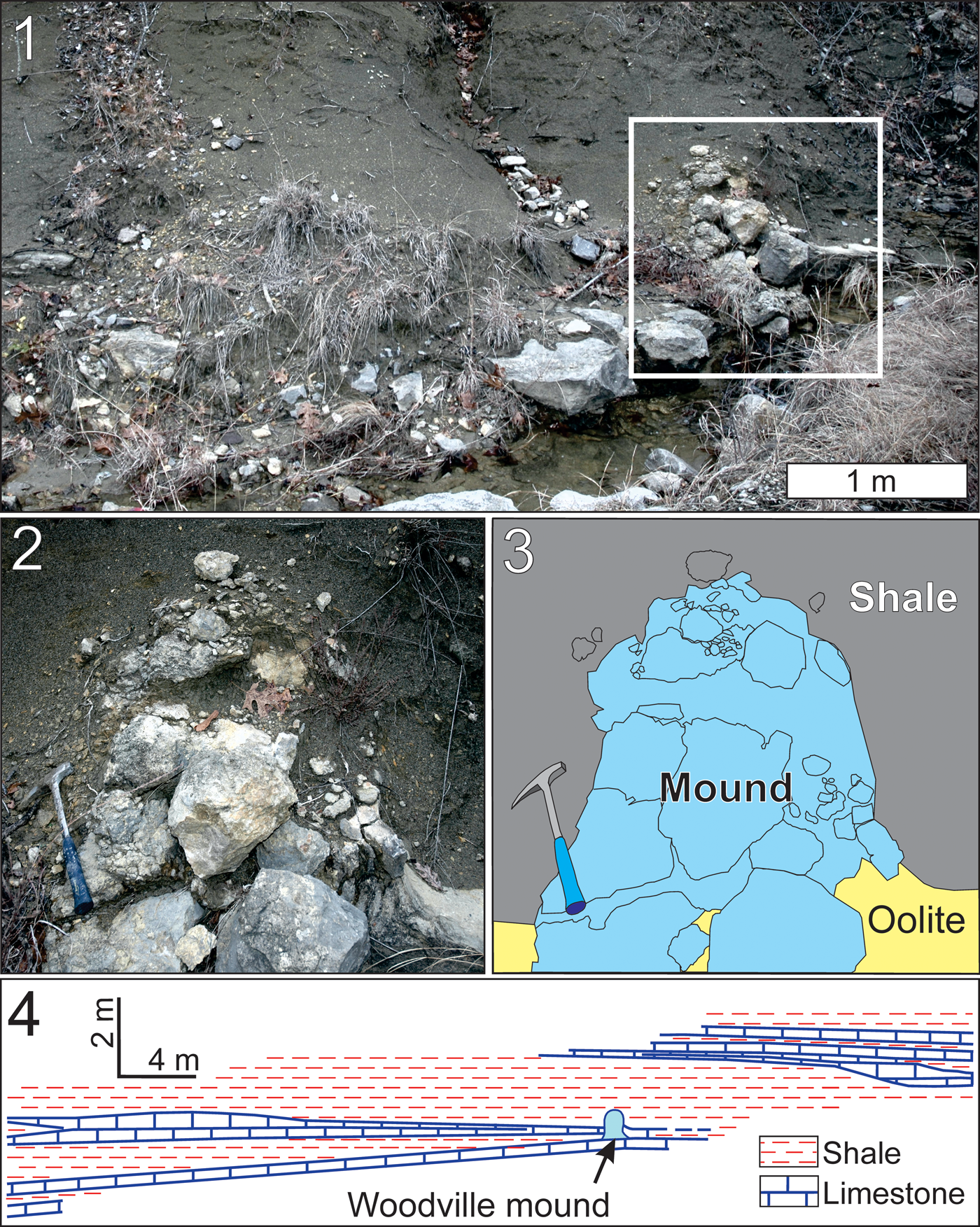
Figure 3. The Woodville mound: (1) the mound in relation to surrounding sandy shale within Hartselle-equivalent strata; (2) close-up of the mound, area indicated by square in Figure 3.1; (3) interpretative sketch of Figure 3.2 indicating lithofacies types; (4) the mound in stratigraphic context (from Keyes, unpublished data).
The purpose of this paper is two-fold: (1) to document the Woodville mound biota, which is unique in Alabama, and (2) to describe a previously unknown mound-binding organism that, along with synsedimentary induration, was instrumental in stabilizing skeletal debris and building this mound.
Geologic setting
A diverse middle Chesterian (lower Serpukhovian; Fig. 1.2) marine fauna is found in Hartselle-equivalent strata at site 1 (Fig. 1.1; Supplementary Data 1) and in much of northeastern Alabama. Crinoid plates and stem segments, Archimedes axes, fragmented fenestrate bryozoan fronds, and brachiopods are the most common fossils. Cephalopods, solitary rugose corals, nonfenestrate bryozoans, chondrichthian teeth, and sponges also are observed.
Stratigraphic setting
The stratigraphic section of interest here is upper Visean and lower Serpukhovian. In northeastern Alabama, the lower middle Chesterian consists of the upper 3–5 m of the Monteagle Limestone and overlying Hartselle-equivalent strata (Keyes, Reference Keyes, Puckett and Rindsberg2014). A distinct change in the echinoderm fauna took place at the base of the middle Chesterian. Archimedes axes and wing plates of Pterotocrinus Lyon and Casseday, Reference Lyon and Casseday1859 became very common and typically are found with cup plates of Agassizocrinus Owen and Shumard, Reference Owen and Shumard1852. Their remains commonly occur together in fossiliferous lenses within the shaley section. It is not unusual to see hundreds of specimens of these taxa, especially Archimedes axes, scattered about a middle Chesterian outcrop (Keyes, Reference Keyes, Puckett and Rindsberg2014). Archimedes axes are found throughout the Mississippian of the eastern midcontinental region, but they are most abundant in the middle Chesterian. Pterotocrinus is a unique camerate crinoid in the eastern midcontinent. Its wing plates evolved rapidly during the Chesterian, and many wing-plate species are useful in biostratigraphy (Butts, Reference Butts1917, Reference Butts1922; Ulrich, Reference Ulrich1917; Weller, Reference Weller1920; Sutton, Reference Sutton1934; Ettensohn et al., Reference Ettensohn, Ausich, Kammer, Johnson, Chesnut and Wong2007).
Fossiliferous rudstone/grainstone lenses that interfinger with shale in the Hartselle-equivalent section at site 1 contain abundant Agassizocrinus cup plates, wing plates of two species of Pterotocrinus (Pterotocrinus depressus Lyon and Casseday, Reference Lyon and Casseday1860 and Pterotocrinus cf. P. armatus Sutton, Reference Sutton1934), and Archimedes axes. Fossiliferous lenses containing an identical fauna are found near the top of the Hartselle-equivalent interval at site 5 (Rigby and Keyes, Reference Rigby and Keyes1998). The presence in lenses at site 1 of Pterotocrinus depressus wing plates, Pentremites tulipaeformis Hambach, Reference Hambach1903, and Pentremites cf. P. sulcatus Roemer, Reference Roemer1851 indicates an affinity with the upper middle Chesterian. These echinoderms are common throughout the lower Bangor Limestone in northern Alabama, Tennessee, and Kentucky, as well as the Glen Dean Limestone of Indiana, Kentucky, and Illinois (Butts, Reference Butts1917, Reference Butts1922; Weller, Reference Weller1920; Horowitz, Reference Horowitz1965). In contrast, specimens of Pterotocrinus cf. P. armatus and Pentremites cervinus Hall, Reference Hall1858 in the fossiliferous lenses at the localities described by Haywick et al. (Reference Haywick, Kopaska-Merkel and Keyes2016) indicate an affinity to the lower middle Chesterian (Sutton, Reference Sutton1934; Galloway and Kaska, Reference Galloway and Kaska1957; Ettensohn et al., Reference Ettensohn, Ausich, Kammer, Johnson, Chesnut and Wong2007).
In the Woodville area, the oldest middle Chesterian fossils are observed at site 3, four meters below the Monteagle-Hartselle contact. Here, Pterotocrinus lingulaformis Sutton, Reference Sutton1934, Pterotocrinus armatus wing plates, and Pentremites cervinus commonly occur with Archimedes axes. Great numbers of Pterotocrinus capitalis (Lyon, Reference Lyon1857) have been observed at the top of the Monteagle Limestone at sites 3 and 5. This species is considered an index fossil of the lower middle Chesterian in southern Illinois and northwestern Kentucky (Butts, Reference Butts1917; Ulrich, Reference Ulrich1917; Weller, Reference Weller1920, Reference Weller1926). Collectively, these echinoderm biostratigraphic data (Fig. 4) confirm that the Hartselle-equivalent unit in the study area is lower middle Chesterian, and that the microbial mound grew during the upper part of this interval.

Figure 4. Lower Carboniferous echinoderm biostratigraphy of northern Alabama. The age of the Woodville mound located at site 1 in Figure 1.1 is indicated. Species authorship (for taxa not in text): Pentremites godoni (Defrance, Reference Defrance1819); Pentremites pyriformis Say, Reference Say1825; Pentremites spicatus Ulrich, Reference Ulrich1917; Platycrinites huntsvillae Troost, Reference Troost1850; Pterotocrinus menardensis Weller, Reference Weller1920; Pterotocrinus serratus Weller, Reference Weller1920; Pterotocrinus tridecibranchiatus Gutschick, Reference Gutschick1965.
Locality information
Our field site (site 1 of Haywick et al., Reference Haywick, Kopaska-Merkel and Keyes2016; Fig. 1.1) is located along U.S. Route 72, just south of Woodville, Alabama, in Thomas Cove, southeastern Jackson County, ~ 35 km east of Huntsville, Alabama (Supplementary Data 1). The Woodville mound is exposed at road level near the top of the section.
In our study area, four roadcuts and one natural outcrop (Supplementary Data 1) expose part of the Monteagle Limestone (upper Visean) and the overlying lower Serpukhovian uppermost Monteagle Limestone and Hartselle-equivalent strata. Many outcrops are capped by the Serpukhovian Bangor Limestone, which overlies the Hartselle-equivalent beds. Hartselle-equivalent strata in northern Alabama are primarily composed of shale, with thin fossiliferous limestone to shale lenses and a few thin, quartzose, sand-rich lenses. To the west, the resistant Hartselle Sandstone forms a natural bench, which in some cases can be walked for kilometers (Thomas, Reference Thomas1972; Rindsberg, Reference Rindsberg1994).
Woodville mound construction
Petrographically, we recognize four structural elements that make up the Woodville mound: (1) relatively large (to 1 cm) skeletal fragments, (2) finely crystalline precipitates made by binding and encrusting organisms, (3) sediment-filled voids, and (4) calcite spar cements precipitated into intraskeletal pores, fenestrae, shelter voids, and fractures. The only remaining porosity in any of the thin sections that we examined was confined to minor fractures and pressure-solution seams.
Petrographic evidence indicates that the Woodville mound grew atop an oolitic grainstone that was cemented onto the seafloor by a marine phase of isopachous radiaxial calcite spar (Haywick et al., Reference Haywick, Kopaska-Merkel and Keyes2016; Fig. 5.1). Some of this marine cement also filled fractures near the periphery of the mound in contact with the oolite, suggesting that induration of the mound also began on the seafloor. The voids that constitute some of the mound's structure and their history of sediment fill provide additional insight into its constructional history. Voids are variable in both size and shape but can generally be grouped into three distinct generations based upon timing. The earliest generation includes intraskeletal voids, fenestrae, primary intergranular pore space, and shelter porosity. These voids are partially to completely filled with dark, nonferroan microcrystalline carbonate (< 10 μm) that, in places, displays geopetal textures suggesting gravitational settling (Fig. 5.2). Sediment fill is likely the same material that makes up the mud matrix of the mound. Borings, which cut through the first generation of voids as well as some skeletal and microbial elements, are filled by a second generation of less turbid, slightly coarser microcrystalline calcite (Fig. 5.2, 5.3). The remaining porosity in both of these kinds of voids is filled by meteoric calcite spar cement.

Figure 5. Thin-section photomicrographs and photographs of polished slabs of cements and void-filling sediment in the Woodville mound: (1) isopachous marine cement overlain by limonite stain (arrow) within ooid grainstone (thin section GSA-I33004); (2) first-generation microcrystalline geopetal fill (a) within a brachiopod; the brachiopod is coated in microencrusters (b) and both are cut by borings containing second-generation carbonate sediment (c) (thin section GSA-I33008); (3) first (a), second (b), and third (c) generation sediment fill in voids within the Woodville mound (thin section GSA-I33008); (4) third-generation sediment fill (arrows) in irregular void spaces within a polished slab of the Woodville mound; the limonite content of the sediment makes the voids very apparent in reflected light (sample GSA-M256); (5) third-generation sediment-filled voids (arrows) sandwiched between Fistulipora bryozoans (polished slab, reflected light, sample GSA-M256); (6) third-generation sediment fill (arrow) draping over microencrusters (a) (thin section GSA-M257).
The latest (third generation) voids are the largest and most dominant in the mound. They are irregularly shaped and can measure up to a few cm across. Some are partially filled by the microcrystalline calcite contained within the second generation voids (e.g., Fig. 5.3), but most are packed with a sediment composed of finely crystalline, limonite-stained ‘pseudospar’ calcite (10–20 μm crystals; 90%), quartz silt (20–50 μm; 2–5%), and what had originally been euhedral dolomite rhombs (20–30 μm; to 5%). Most dolomite rhombs were calcitized (dedolomitized) during postdepositional meteoric alteration. Because of the limonite content of the sediment fill, the distribution of third-generation voids in the mound is best observed in polished slabs under reflected light. Most of the voids are randomly oriented vugs and fractures that cut through mound constituents (Fig. 5.4), however, some are sandwiched between skeletal fragments, which in places imparts a parallel disposition to mound components (Fig. 5.5). Under transmitted light, some of the sediment in these voids can be seen draping over microbial colonies (Fig. 5.6). That they were buried rather than truncated suggests that the colonies might have been actively growing into some third-generation void spaces before sediment infiltration. The same limonite-stained sediment also fills the intergranular void space within portions of the oolite below the mound and clearly postdates the marine isopachous cement (Fig. 5.1).
Mound composition
Skeletal elements
Skeletal organisms constitute < 30% of the Woodville mound and primarily consist of Fistulipora colonies (Fig. 5.4) with minor amounts of gastropods, brachiopods, crinoid ossicles, encrusting tabulate corals, and small sponges, including Pileospongia (Fig. 6). The iconic Serpukhovian (Chesterian) fenestrate bryozoan Archimedes, which is abundant in the Hartselle-equivalent shale that encompasses the mound, has not been identified in the mound itself. Indeed, few fenestrate bryozoans were found in the mound proper; however, fenestrate fragments in the oolite below the mound commonly acted as nuclei for ooid growth (Fig. 5.1). Skeletal elements are sporadically distributed within the mound and appear in places to be subparallel to one another (Figs. 5.5, 7.1). Other than their local subparallel disposition, skeletal elements exhibit no consistent orientation. Although ooid grainstone is in direct contact with the lower part of the mound, only a few ooids were found embedded within the mound.

Figure 6. Thin-section photomicrographs of sponges from the Woodville mound: (1) cross section of whole sponge with monaxon spicules (a); the left margin of the sponge is indicated by the arrow labeled b (thin section GSA-M258); (2) cluster of monaxon sponge spicules (a) overlying shelter void (b) containing micropelletal cement (c); a cluster of coccoid microfossils (d) is visible in the center of the photograph (thin section GSA-M259); (3) Pileospongia Rigby, Keys, and Horowitz, Reference Rigby, Keyes and Horowitz1979 (arrows) (thin section GSA-M260); (4) vase-shaped sponge (a) that was enveloped by a consortium of encrusters dominated by Aphralysia anfracta n. sp. and nonskeletal microbes; monaxon spicules are piled up in the bottom of the cavity once occupied by the sponge; thin-walled tubes of Problematicum A (b) are common in this view as are numerous examples of Aphralysia anfracta n. sp. (c) (thin section GSA-M261).

Figure 7. Thin-section photomicrographs of constituents within the Woodville mound: (1) cement-filled Fistulipora interlayered with third-generation void-fill sediment displaying subparallel orientation (thin section GSA-M262); compare to Webb (Reference Webb1987, fig. 8A); (2) coccoid microfossils (arrow); close-up of Figure 6.2 (thin section GSA-M259); (3) thin-walled cryptic organism (a) in a cement-filled void within a specimen of Fistulipora; pore spaces within the bryozoan and fenestrae (b) are filled by geopetal sediment (c) or are lined by possible micropelletal precipitates (d) (thin section GSA-M263); (4) close-up of rectangle indicated in Figure 7.3; Aphralysia anfracta n. sp. (a) suspended from the base of a specimen of Fistulipora; note the geopetal nature of micropelletal cement within the bryozoan chamber (b) and in a fenestral void (c) (thin section GSA-M263); (5) numerous colonies of Aphralysia anfracta n. sp. (arrows) binding sediment, bryozoans (a), and other skeletal elements within the Woodville mound complex (thin section GSA-M264); (6) domal composite structure consisting of alternating microcrystalline microbial layers and thinner layers made by Aphralysia anfracta n. sp. (a); similar to Webb (Reference Webb2005, figs. 8B, 12B); the digitate stromatolite envelops a large area of clotted microcrystalline carbonate containing possible monaxon sponge spicules (b) and overlying a large void filled with calcite cement; this could be a badly decayed sponge (thin section GSA-M258).
Cryptic organisms
Potential evidence of cryptic organisms in the mound includes the remains of coccoid microbes, pendant microcrystalline calcite in voids, and small sponges. These cover a wide range of sizes. In one example, coccoid microbes and pendant microcrystalline calcite occur together in a complex former void (Figs. 6.2, 7.2). Micropelletal microcrystalline cement appears to have been the first material precipitated into this void. Most of the remaining space was filled with drusy calcite spar (discussed further under void-filling cement). Conical projections from the top of the void are pendant microcrystalline carbonate that formed before precipitation of the drusy spar, probably under the influence of nonskeletal microbes. A cluster of loosely packed spheroids to the left of and below the void probably records the growth of coccoid microbes. This might have been part of the original void. If so, the colony grew before precipitation of micropelletal calcite. The coccoid bodies are composed of microcrystalline calcium carbonate and are clustered but not adpressed. Some are structureless; others contain slit-like voids that are filled with calcite cement (Fig. 7.2). The coccoid bodies form a mass ~ 1 mm across. Individual spheroids range ~ 30–60 μm in diameter. Further, beneath the colony and the void next to it is a packstone consisting of angular particles 150–300 μm across (fine to medium sand size), embedded in dark gray microcrystalline carbonate, which might be microbial. This area also might have originally been part of the void. Other possible examples of cryptic organisms include small sponges entombed within the mound (e.g., Fig. 6). Pore-lining and geopetal deposits, some composed of micropelletal calcite, are found in small fenestrae, bryozoan chambers, and other void spaces (Fig. 7.3, 7.4). That some pore-lining occurs along the tops of voids defying gravity suggests microbial influence. Nearby cavities contain both upright and pendant microcrystalline encrustations (Fig. 7.4).
Binding material
The majority of the skeletal elements, predominantly bryozoans, are discontinuously coated and bound together by two components. These are (1) microcrystalline calcium carbonate, and (2) tubules of a variety of sizes, tightness and regularity of curvature, and wall structure, all filled with calcite cement. These encrusting materials bound together not only the large bryozoans, but also coarse detritus, including gastropod shells and other fossil fragments (Fig. 7.5). Sponges (Fig. 6) helped to bind materials in the mound.
The microcrystalline calcium carbonate ranges from structureless to clotted to crudely laminated. It directly coats bryozoans and other large particles. It also coats tubular encrusting microfossils (Aphralysia anfracta new species and Aphralysia capriorae Mamet and Roux, Reference Mamet and Roux1975), and forms projections that appear to have extended into the water and away from the main mass of boundstone (Fig. 7.4). In a few cases, microcrystalline calcium carbonate and tubular microfossils are interlaminated, forming domal structures (Fig. 7.6).
Where encrusters are present, microcrystalline calcium carbonate coats them unevenly, fills the spaces among them, and in some cases completely engulfs them. Tubules either form compact masses or encrust other objects. There are at least three kinds of tubules composing the mound (and a fourth found reworked into ooid grainstone directly beneath the mound). These are described in later sections.
Small oncoids a few millimeters across (discussed further in a later section) are embedded in the mound, cemented in place by microcrystalline calcium carbonate and by Aphralysia anfracta n. sp. Because of their subspherical shape, we infer that the oncoids initially formed as free-rolling objects. The oncoids primarily consist, in order of decreasing abundance, of: (1) clotted microcrystalline calcium carbonate, (2) tubular microfossils referred to Aphralysia anfracta n. sp., (3) clusters of vesicles filled with white calcite cement, referred to Aphralysia capriorae, and (4) larger thin-walled calcite tubes that encrusted (and perhaps bored into) the oncoids, referred to Problematicum A. A small amount of boundstone debris (tuberoids; sponge or boundstone intraclasts) containing Aphralysia anfracta n. sp. and other mound components has been found in sandy silty calcareous mudstone above the mound.
An ooid in ooid grainstone directly beneath the mound contains adpressed calcite-walled vesicles, referred to Problematicum B. It is not known if Problematicum B served as a binder elsewhere.
Void-filling cement
Cement fills structural voids within fossils such as bryozoans and gastropods, fenestrae, shelter voids, and fractures that crosscut other structural components (e.g., Fig. 7.3, 7.5). The same synsedimentary isopachous radiaxial calcite spar that cemented the oolite on which the mound nucleated also lines some of the void spaces within the mound. In places, this phase of cement postdates the first generation of void-filling sediment, but in others, it predates the sediment, indicating that the two processes were simultaneous (i.e., before boring). Volumetrically, however, most spar cement within voids is drusy calcite (Fig. 7.4). This phase of cementation occurred during dissolution of aragonitic shells (e.g., gastropods) and was precompactional and nonferroan, suggesting precipitation during early meteoric (phreatic) diagenesis (James and Choquette, Reference James and Choquette1984). Many of the larger voids within the Woodville mound that contain mixed siliciclastic and carbonate sediment are stained with limonite (Fig. 5.3–5.6). Limonite also coats the radiaxial calcite spar in the oolite (Fig. 5.1), indicating that it too is a product of early meteoric diagenesis.
Materials and methods
Approximately 15% by volume of the mound was collected from the site, from which 57 thin sections were manufactured by us. Forty-eight of the thin sections were standard sized (2.7 cm by 4.6 cm). Nine were large format (7.6 cm by 5.0 cm). Eight of the standard-sized thin sections were polished to thicknesses < 30 μm. Two polished slabs (~ 15 cm by 8 cm) were also produced to examine macroscopic structures in the mound. Thin sections were used to examine microbial structures and their relationships to other mound components. Specimens of Aphralysia anfracta n. sp. were point counted using a grid digitally laid over digital thin-section photomicrographs, using Adobe Illustrator™ (Supplementary Data 2). Microfossils and other rock components were measured on digital photographs using calibrated scales in Adobe Illustrator™.
Repository and institutional abbreviation
Bulk samples containing fossil specimens collected from the Woodville mound site during this study, as well as all slabs and thin sections produced during this analysis, are housed in the Geological Survey of Alabama Paleontology Collection (GSA) in Tuscaloosa, Alabama (Supplementary Data 3). All type material consists of petrographic thin sections.
Systematic paleontology
Problematica
Family Aphralysiaceae Vachard et al., Reference Vachard and Krainer2001
Genus Aphralysia Garwood, Reference Garwood1914
Type species
Aphralysia carbonaria Garwood, Reference Garwood1914, p. 269, pl. 21, figs. 3, 4. Lower Carboniferous, Ravenstonedale, Westmoreland, England, by original designation.
Aphralysia anfracta new species
Figures 6.4, 7.3–7.6, 8–10, 13.3–13.6, 14.3, 14.4
- Reference Aretz and Herbig2003
Aphralysia sp.; Aretz and Herbig, pl. 37, figs. 5, 6, pl. 39, fig. 6.
- Reference Aretz and Herbig2008
Aphralysia sp.; Aretz and Herbig, fig. 10F.

Figure 8. Thin-section photomicrographs of Aphralysia anfracta n. sp.: (1) tubules (arrows) wandering through enclosing fine sediment, patchily coated with microcrystalline carbonate (thin section GSA-M265); (2) forms characterized by small equant vesicles in gently curving lines (box) and sinuous tight curves (arrows); some tubules in contact with a large calcitic mass (a) have been partially overprinted, suggesting postgrowth neomorphic alteration of a former skeletal allochem (thin section GSA-I33006). (3) holotype, Aphralysia anfracta n. sp. boundstone illustrating possible examples of branched tubules (a), a wide size range of tubules, and tightly curved areas (b); the image also contains a possible longitudinal cross section through a specimen of Problematicum A (c) (thin section GSA-M263). Figures 8.1–8.3 each show multiple examples of tightly curved segments of Aphralysia anfracta n. sp.
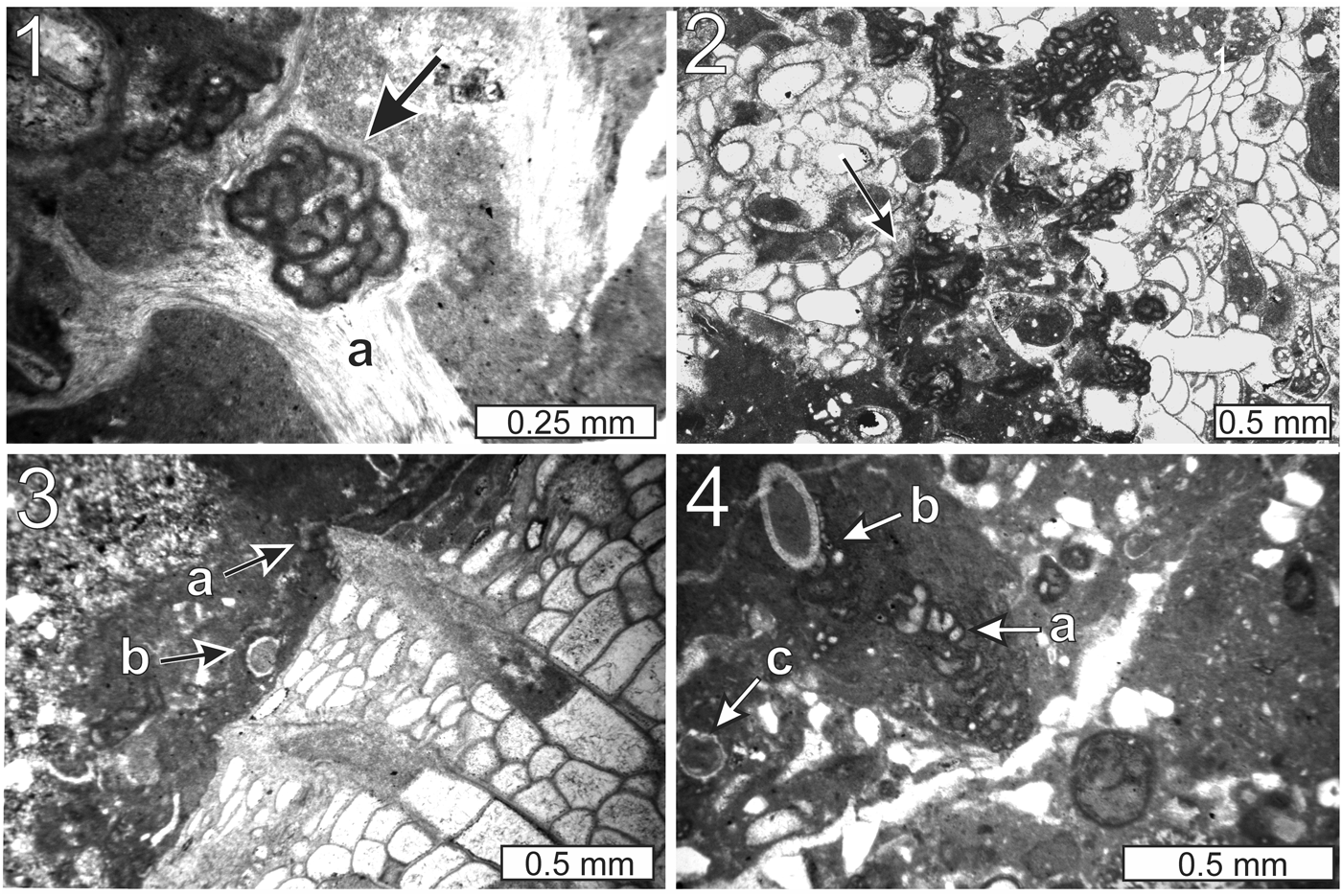
Figure 9. Thin-section photomicrographs of Aphralysia anfracta n. sp.: (1) arrow, encrusting fenestellid bryozoan (a) and vice versa (thin section GSA-I33006); (2) arrow, encrusting Fistulipora (thin section GSA-M261); (3) at (a) and microcrystalline carbonate encrusting Fistulipora and Problematicum A (b) (thin section GSA-I33007); (4) at (a), encrusting brachiopod spine (b) and thickly coated with microcrystalline carbonate; Problematicum A (c) is visible in the lower left quadrant of the image (thin section GSA-M263). Note tightly curved segments of Aphralysia anfracta n. sp. in Figures 9.1 and 9.4.

Figure 10. Thin-section photomicrographs of Aphralysia anfracta n. sp.: (1) spheroidal masses (a) embedded in microcrystalline carbonate; Problematicum A (b) is also present in the image (thin section GSA-M263); (2) gastropod encrusted by microcrystalline carbonate and Aphralysia anfracta n. sp. (a); geopetal sediment (b) fills a portion of the gastropod chamber (thin section GSA-M263); (3) arrow, enveloped by microbial carbonate, part of thick and complex encrustation on Fistulipora (outside field of view) (thin section GSA-I33006); (4) tightly curved tubule form with apparent swollen terminus (arrow) (thin section GSA-M258).
Type specimens
Holotype, GSA-M259 (Fig. 8.3). Topotypes, GSA-I33003–I33005, I33007, GSA-M256–M258, and M260–M263.
Diagnosis
Tubules; nonseptate; walls composed of dark-colored microcrystalline or microsparitic calcite (probably original mineralogy and texture) to 10 μm thick; length considerably greater than diameter; tubules intersecting planar surfaces in continuous sections as long as 0.4 mm; nearly straight to tightly curved and recurved; wall thickness and tubule diameter correlated; tubules rarely, if ever, branching.
Occurrence
Hartselle-equivalent (lower Serpukhovian, lower middle Chesterian, upper Mississippian) mound in silty sandy mudstone at site 1 (34.615591°N, 86.225243°W), near Woodville, east of Huntsville, Alabama. Specimens are found encrusting macrofossils or floating in matrix. Tuberoids containing Aphralysia anfracta n. sp. occur in sandy silty calcareous mudstone above the mound.
Description
Tubular calcitic microfossils, circular in cross section, walled with dark microcrystalline or microsparitic calcite, commonly, but not invariably, coated with or embedded in microcrystalline carbonate that is lighter in color than the tubule walls, and which extends beyond the tubules to envelop nearby particles (Figs. 9.4, 10.3) or as fingerlike projections. The most characteristic feature of this organism is the highly contorted nature of a minority of the tubules (Fig. 8.3; Supplementary Data 2). These contorted segments range from loosely to tightly curved; curves are regular and symmetrical to irregular and asymmetrical. Tubules cover a wide range of sizes, and contorted tubules represent the entire size range observed in a given sample (Fig. 8.3). Tubules are not segregated according to diameter, orientation, or degree of curvature. Portions of tubules intersected by the plane of a thin section maintain a nearly constant diameter from end to end. Wall thickness is typically 5–10 μm, inner diameter 10–30 μm, and external diameter 20–45 μm (approximate, mean ± 1 standard deviation; for raw data, see Supplementary Data 2). In a very few cases, tubules appear to bifurcate (Fig. 8.3). Apparent bifurcations are so rare that they might result simply from chance superposition of unbranched tubules.
Most specimens have an encrusting habit, attaching themselves to larger objects such as bryozoans, gastropods, or other encrusters, such as Problematicum A (see below). Some specimens appear to form, with other encrusters, free-floating oncoids, containing one or a few individuals of Aphralysia anfracta n. sp. These can be trapped in voids within the mound boundstone mass or can be attached to it in the third dimension. In a few cases, tubules are randomly oriented through fine sediment, and have thin and patchy coatings of microcrystalline calcite (Fig. 8.1).
Small, simple encrustations appear to consist of single specimens of Aphralysia anfracta n. sp., which gradually increase in diameter from one end to the other (but so gradually, that a uniform diameter is nearly always observed within a single transected part of a tubule). Larger encrustations or free-floating clusters almost certainly consist of multiple individuals.
Etymology
The species name is from the Latin anfractus, meaning bending or winding, in reference to the tightly curved tubule form that is commonly exhibited by specimens of this species.
Affinities
In this section, we discuss evidence for and against assigning Aphralysia anfracta n. sp. to various groups of microfossils.
Problematica
Nonseptate tubules, walled with microcrystalline or finely crystalline calcium carbonate, are common in the Paleozoic. A variety of microorganisms, ranging from foraminiferans to cyanobacteria to problematica, have been suggested as the makers of Aphralysia (personal communications, D. Vachard, B. Granier, and B. Pratt, 2016). Vachard et al. (Reference Vachard, Cózar, Aretz and Izart2016) illustrated examples of several species of the poorly understood encruster Aphralysia that were collected from late Visean–early Serpukhovian strata of the Montagne Noire of France. They referred these specimens to cyanobacteria. Aphralysia ferreoli Mamet and Roux, Reference Mamet and Roux1975 resembles the specimens described here; the tubules of the latter figured by Vachard et al. (Reference Vachard, Cózar, Aretz and Izart2016, fig. 2B, F) present an undulating aspect reminiscent of some of our material. In addition, tubule size and wall structure are similar (Table 2). However, their photographs do not show tight curvature in any of the tubules; the presence of tightly curved tubules is diagnostic of material from the Woodville mound.
Table 2. Sizes of some Aphralysia-like forms, scale in μm.

*Point-counted; see Supplemental Data 4.
**Including small vesicles, circular in cross section, that are noticeably smaller than many other specimens that they studied.
Calcimicrobes
An extensive literature search has not revealed any calcimicrobe with characteristics exactly matching Aphralysia anfracta n. sp. However, our material has been suggested to belong to the well-known calcimicrobial genus Renalcis Vologdin, Reference Vologdin1932 (written communication, B.R. Pratt, 2017). Any resemblance to Renalcis is superficial. For example, Renalcis exhibits closely spaced branching, whereas Aphralysia anfracta n. sp. tubules branch rarely, if at all. Secondly, Renalcis is commonly septate or lacks a lumen entirely, whereas Aphralysia anfracta n. sp. tubules are neither septate nor solid; most examples appear to consist of single elongate chambers. Tightly curved portions of Aphralysia anfracta n. sp. appear to be partially cut by incomplete septa, but this is an illusion, caused by the tight bending of the tubules, and the fact that the doubled tubule wall on the inside of the bend is commonly very thin (Figs. 8.3, 9, 10.4). Further, both wall thickness and chamber diameter of Renalcis are far more variable over short distances (on the order of tens of μm). Some of this variability is likely a result of the strong diagenetic overprint on Renalcis (Pratt, Reference Pratt1984). Where Renalcis is hollow, and not septate, it forms bulbous or cylindrical chambers that are commonly attached to one another, forming a branching network of blobs reminiscent of yeast colonies. This aspect is quite different from the sinuous and unbranched tubes prevalent in Aphralysia anfracta n. sp.
Cyanobacteria and algae
In terms of wall structure and tube diameter, Aphralysia anfracta n. sp. resembles some cyanobacterial filaments and microproblematica, such as those illustrated by Riding and Soja (Reference Riding and Soja1993). The primary difference between Aphralysia anfracta n. sp. and microalgae and cyanobacteria is that the latter two tend to have straight or gently curving tubules. No known members of either of these two groups grow filaments that are tightly bent. One would not expect a photosynthesizing organism, as most cyanobacteria are, to spend as much time growing away from the light as would be required to form tight curves. Also, many cyanobacterial and algal filaments are septate.
Foraminifera
Aphralysia anfracta n. sp. tubules resemble various small porcelaneous foraminiferans, in particular, nubeculariids, some of which approximate the characteristic tightly curved tubular morphology in their terminal chambers. These include Calcivertella Cushman and Waters, Reference Cushman and Waters1928 and Ammovertella Cushman, Reference Cushman1928 (examples illustrated by Croneis and Toomey, Reference Croneis and Toomey1965; Groves, Reference Groves1983; Vachard et al., Reference Vachard, Martini, Zaninetti and Zambetakis-Lekkas1993; Vachard and Krainer, Reference Vachard and Krainer2001; Nestell et al., Reference Nestell, Nestell, Wardlaw and Sweatt2006; Cózar et al., Reference Cózar, Somerville and Burgess2008, Reference Cózar, Somerville and Burgess2009; Błaźejowski, Reference Błaźejowski2009), of which the former is a junior synonym of the latter (Vachard and Krainer, Reference Vachard and Krainer2001). Minammodytes Henbest, Reference Henbest1963 exhibits a rudimentary tightly curved form in its terminal chamber (Croneis and Toomey, Reference Croneis and Toomey1965).
Juvenile portions of these foraminiferans resemble those of other small porcelaneous forms (see e.g., Nestell et al., Reference Nestell, Nestell, Wardlaw and Sweatt2006; Cózar et al., Reference Cózar, Somerville and Burgess2009), but many published examples include only the more mature wandering or tightly curved portion of the shells (Groves, Reference Groves1983; Vachard et al., Reference Vachard, Martini, Zaninetti and Zambetakis-Lekkas1993; Vachard and Krainer, Reference Vachard and Krainer2001; Cózar et al., Reference Cózar, Somerville and Burgess2008). No sections through unequivocal juvenile portions of typical small porcelaneous foraminiferans have been found in specimens of Aphralysia anfracta n. sp. in the Woodville mound, or within tubule-bearing tuberoids floating in the Hartselle-equivalent shale nearby. A few small circular chambers, some surrounded by larger chambers, have been found. These might correspond to juvenile parts of specimens of Aphralysia anfracta n. sp. (e.g., Fig. 10.4).
Worm tubes
We have found no known fossil worm-tube taxa to which these fossils can be confidently assigned. Most calcified worm tubes are much too large. Serpulid tubes, for instance, are both too large and have a laminated wall structure that is quite unlike that of Aphralysia anfracta n. sp. Sabellid worms make tubes that are contorted like the ones described here, and they are smaller than serpulid tubes, but their typical diameters in the neighborhood of 500 μm are still much larger than those that we found (e.g., Perkins, Reference Perkins1991). A survey of the literature revealed no calcified worm tubes as small as the tubules described here (< 0.1 mm in diameter). To some extent, this could be a result of collecting bias. Smaller worms exist in the Recent, but we know of no examples of calcium-carbonate tubes constructed by them.
Remarks
When examining our specimens at the highest magnification possible with the instruments at our disposal, the tubule walls appear to consist not of microcrystalline calcite, but of equant calcite crystals with diameters approximately the width of the wall. This is similar to diagenetically altered walls described from modern subfossils that resemble Wetheredella Wood, Reference Wood1948 (Kaźmierczak and Kempe, Reference Kaźmierczak and Kempe1992), but could be an agglutinated structure composed of microspar particles collected from the mound.
A tubular fossil that appears to undulate in two dimensions might be created diagenetically by compaction from an originally helical form. In the case of Aphralysia anfracta n. sp. in the Woodville mound, such a process is not likely to have taken place. Early cementation of the mound, in the form of: (1) marine cements, and (2) thick encrustations of calcium carbonate that were precipitated by a consortium of nonskeletal microbes and calcimicrobes, appears to have prevented significant compaction. This inference is supported by three observations. First, allochems (e.g., oncoids), larger macrofossils (e.g., sponges), and skeletal debris in general show no noticeable distortion. Second, there is no fracturing of fragile mound components (e.g., gastropods). Third, tightly curved tubules of Aphralysia anfracta n. sp. are seen in many different orientations. Vertically and horizontally oriented helices would look quite different from one another after compaction.
Figure 8.2 shows several examples of groups of equant vesicles arranged in straight or gently curving lines, which appear to be specimens of Aphralysia anfracta n. sp., based on their association with other tubules. They can be tightly curved tubules that undulate in a plane approximately normal to the plane of the thin section. Other photographs show similar gently curved chains of equant vesicles within specimens of Aphralysia anfracta n. sp.
Figure 8.2 also shows partial destruction of tubules by a large mass of what appears to be neomorphic calcite. The overall concentric and convex-outward orientation of tubules and of the tubule mass suggests that it encrusted an object that has been replaced by calcite cement, followed by neomorphic replacement of parts of the encrusting tubule mass.
Should all of these specimens, which share a common wall microstructure, be assigned to a single species? The percentage of tubules in a single photograph that are tightly curved ranges 2–54% (Supplementary Data 2). This suggests that two species might occur together, intimately intermingled—an unusual highly contorted species and another much less contorted, but otherwise similar. We measured wall thickness, internal diameter, and external diameter on 604 randomly selected tubules from nine photographs, of which 86 are tightly contorted (Supplementary Data 2). Internal and external diameters are correlated, hence, wall thickness is also correlated with tubule diameter. The size distributions approximate normal distributions, suggesting that only one species is present (Fig. 11). In addition, box plots of both internal and external diameters (Fig. 12) show that means and standard deviations of tubule size for all of these samples are similar, regardless of the proportion of tightly curved tubules that were sampled. We conclude that all of these specimens should be assigned to a single species.

Figure 11. Scatter plots of measurements of tubule exterior and interior diameters from five thin-section photomicrographs bearing Aphralysia anfracta n. sp. Means for each data set are indicated by crosses. Measurements listed in Supplementary Data 2.

Figure 12. Box plots of interior vs. exterior diameters of Aphralysia anfracta n. sp. specimens measured in nine photomicrographs imaged from Woodville-mound thin sections. Five of the images contained many contorted tubules (> 20% of the total counted). Two were characterized by few contorted tubules (< 20% of the total counted). We did not distinguish between contorted and noncontorted tubules in two of the images (undifferentiated tubules). Measurements listed in Supplementary Data 2.
The vesicles of Aphralysia capriorae and Aphralysia carbonaria differ from those of Aphralysia anfracta n. sp. in being rather short and domical. The vesicles of Aphralysia capriorae are comparable in their short dimensions to those of Aphralysia anfracta n. sp., whereas those of Aphralysia carbonaria are considerably larger (Table 2). All species of Aphralysia appear to have walls composed of microcrystalline calcite, but those of Aphralysia capriorae are thinner and lighter in color than those of Aphralysia anfracta n. sp. In addition, specimens of Aphralysia anfracta n. sp. exhibit many circular tubule cross sections in thin section, indicating that the tubules are cylindrical. Specimens of Aphralysia capriorae from the Woodville mound and some from the literature (e.g., Mamet and Roux, Reference Mamet and Roux1975) exhibit few circular tubule cross sections, suggesting that the vesicles seen in thin section are cross sections through objects that are for the most part not cylindrical, but perhaps tabular or loaf-shaped. Some other published photographs of Aphralysia capriorae (e.g., Vachard et al., Reference Vachard, Cózar, Aretz and Izart2016) do contain many vesicles or tubules with circular cross sections, but any attempt to revise this species is beyond the scope of this paper. Aphralysia matthewsi Mamet and Roux, Reference Mamet and Roux1975, is considerably smaller than all other named species, and Aphralysia garwoodi Hallett, Reference Hallett1970, is much larger (vesicles average 400 μm high and 1,800 μm across; vesicle walls averaging 50 μm in thickness). Aphralysia ferreoli closely resembles Aphralysia capriorae in form but contains vesicles that are elongate in cross section and probably tubular. Previously named species of Aphralysia— Aphralysia capriorae, Aphralysia carbonaria, Aphralysia ferreoli, Aphralysia garwoodi, and Aphralysia matthewsi—do not contain any tightly curved tubules.
Shen and Webb (Reference Shen and Webb2008, fig. 9A) referred to Aphralysia sp. a specimen from the Visean of Queensland, Australia, which is remarkably similar to our material. Their specimen includes tubules with rather tight bends, but it is not possible to say without more information whether this is a specimen of Aphralysia anfracta n. sp.
The most likely example of Aphralysia anfracta n. sp. in the published literature comes from Aretz and Herbig (Reference Aretz and Herbig2003, pl. 37, figs. 5, 6, pl. 39, fig. 6). They identified as Aphralysia sp. a common encrusting component of Welsh Visean mounds that exhibited numerous tightly bent tubules. The same authors also illustrated a specimen of Aphralysia from the Visean of Morocco that appears to contain tightly curved tubule sections (Aretz and Herbig, Reference Aretz and Herbig2008, fig. 10F).
Aphralysia capriorae Mamet and Roux, Reference Mamet and Roux1975
Figure 13
- Reference Mamet and Roux1975
Aphralysia capriorae Mamet and Roux, p. 157, pl. 12, figs. 10–15, pl. 13, figs. 1–3.
- Reference Mamet, Mortelmans and Roux1978
Aphralysia aff. A. capriorae; Mamet et al., p. 364, pl. 6, figs. 2, 3.
- Reference Adams1984
Aphralysia sp.; Adams, p. 237, fig. 4?
- Reference Vachard and Aretz2004
Aphralysia capriorae; Vachard and Aretz, p. 649, fig. 7.1, 7.2.
- Reference Rodríguez2004
Aphralysia sp.; Rodríguez, fig. 4C.
- Reference Shen and Webb2008
Aphralysia sp.; Shen and Webb, p. 94, figs. 9A, 10C.
- Reference Vachard, Cózar, Aretz and Izart2016
Aphralysia capriorae; Vachard et al., fig. 2E
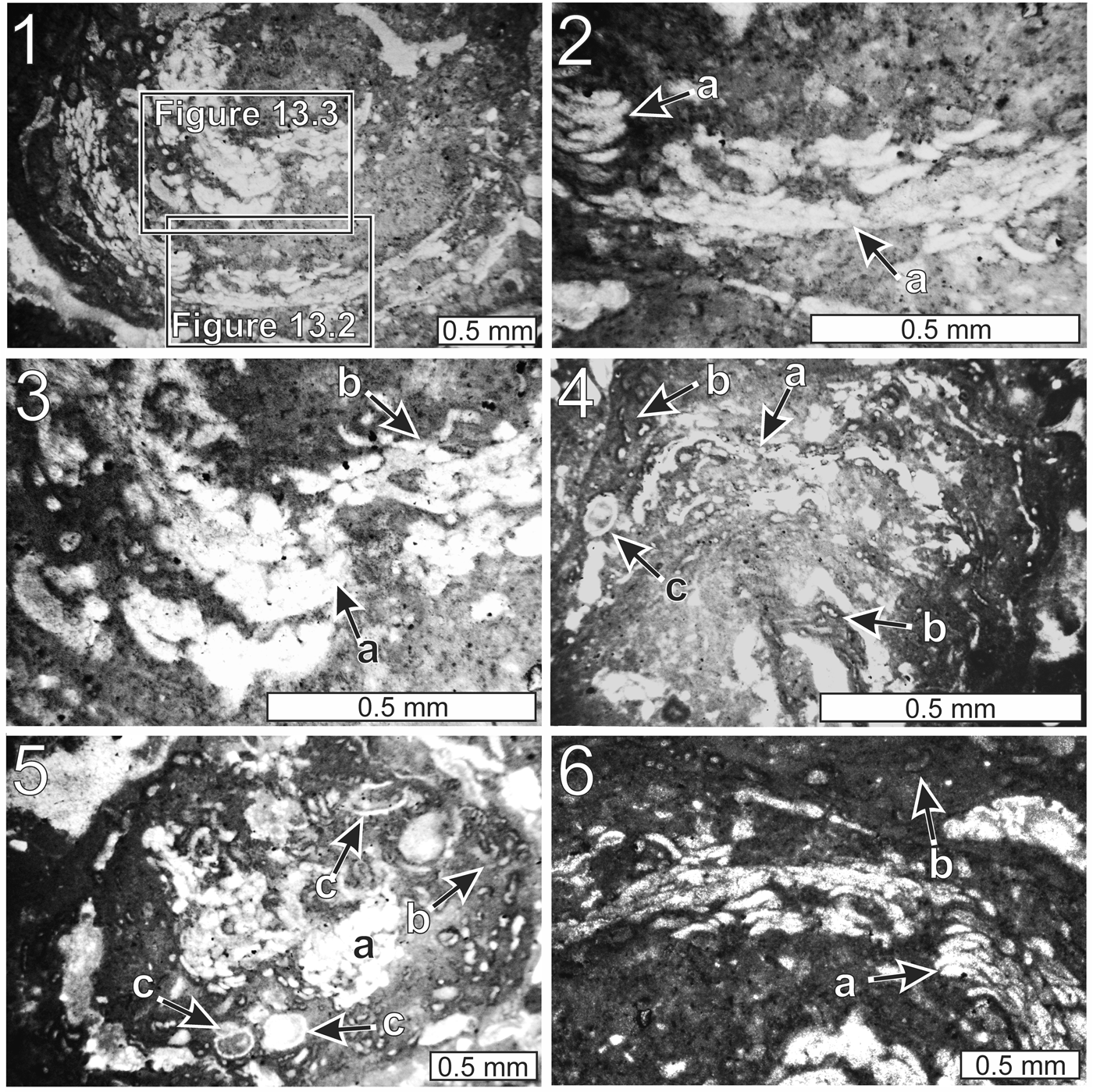
Figure 13. Thin-section photomicrographs of Aphralysia capriorae Mamet and Roux, Reference Mamet and Roux1975 (all from thin section GSA-M265): (1) portion of oncoid, embedded in mound, constructed by a consortium of Aphralysia capriorae, minor Aphralysia anfracta n. sp., and nonskeletal microbes; (2, 3) close-ups of Figure 13.1: Aphralysia capriorae (a), Aphralysia anfracta n. sp. (b); (4–6) portions of oncoid constructed by a consortium of Aphralysia capriorae (a), Aphralysia anfracta n. sp. (b), Problematicum A (c), and nonskeletal microbes.
Holotype
Repository unknown, Ro 42/12 (site 144), Cabrieres, France, zone 16iur., upper Visean (Mamet and Roux, Reference Mamet and Roux1975, pl. 12, fig. 13).
Description
Vesicles within oncoids (within the Woodville mound; a similar occurrence was illustrated by Vachard and Aretz, Reference Vachard and Aretz2004) or encrusting clasts; vesicles wider (parallel to the oncoid surface) than thick (normal to the oncoid surface), of equal thickness throughout, or tapering from maximum thickness near center or at one end, tightly clustered or in contact with one another, straight or curved. Curved vesicles convex outward from near center of oncoid (Fig. 13.1). Vesicles not branching, not septate (Fig. 13.2, 13.3, 13.6). Vesicles unlined, or lining very thin, nearly transparent (Fig. 13.3). Vesicle thickness (normal to oncoid surface) 10–40 μm, averaging 26 μm. Most vesicles wider than thick as seen in thin section; most considerably shorter than 100 μm in long dimension; a few as long as 200 μm. Vesicles, which can be as much as several hundred micrometers across, not cylindrical, like those of Aphralysia anfracta n. sp. Few vesicles with circular cross sections within specimens of Aphralysia capriorae; these structures loaf-shaped or tabular.
Vesicles embedded in clotted light to dark-gray microcrystalline carbonate; clots equant to vermiform; to 30–40 μm across (Fig. 13.1–13.3). Small dark gray clots probably rich in remnant organic material. Microcrystalline carbonate in these oncoids interpreted as microbial in origin. Aphralysia anfracta n. sp. recognized as minor component in some oncoids on basis of wall structure and tubule diameter. No tightly curved tubules observed in association with Aphralysia capriorae.
Materials
Oncoids embedded in the Woodville mound, thin section GSA-M262.
Remarks
For a comparison of Aphralysia capriorae and Aphralysia anfracta n. sp., see Remarks under the latter species.
Sparaphralysia Vachard in Vachard and Beckary, Reference Vachard and Beckary1991 has a similar growth habit (Vachard et al., Reference Vachard, Hauser, Martini, Zaninetti, Matter and Peters2001), but vesicles are considerably smaller. Aphralysia matthewsi and Aphralysia garwoodi both have similar growth habits, but the former is much smaller, and the latter is much larger than Aphralysia capriorae (see Hallett, Reference Hallett1970; Mamet and Roux, Reference Mamet and Roux1975). Aphralysia carbonaria, the type species, also resembles Aphralysia capriorae. However, Aphralysia carbonaria is significantly larger than Aphralysia capriorae, although substantially smaller than Aphralysia garwoodi (see Garwood, Reference Garwood1914; Hallett, Reference Hallett1970). Aphralysia ferrioli resembles Aphralysia capriorae, but the vesicles are wider, and some appear tubular in thin section (Mamet and Roux, Reference Mamet and Roux1975).
Specimens identified as Aphralysia sp. from the Visean of Poland (Belka, Reference Belka1981), resemble Aphralysia capriorae and are approximately the right size. However, Belka's material is septate and the walls are perforated; these characteristics are not shared either with the material that we assign to Aphralysia capriorae nor with specimens illustrated by other authors.
Jarochowska and Munnecke (Reference Jarochowska and Munnecke2014) reinterpreted the problematicum Allonema Ulrich and Bassler, Reference Ulrich and Bassler1904 as a foraminiferan (and synonymized another problematicum, Wetheredella, with it). These fossils resemble Aphralysia capriorae. However, specimens of Allonema sp. figured by Jarochowska and Munnecke (Reference Jarochowska and Munnecke2014) have a double-layered wall structure. Our material does not show double-layered walls, but the quality of preservation might be inadequate to make such a determination. Further, Paleozoic Allonema and Wetheredella spp. have perforate walls (Vachard and Aretz, Reference Vachard and Aretz2004; Jarochowska and Munnecke, Reference Jarochowska and Munnecke2014), but the walls of at least some specimens of Aphralysia are imperforate (Vachard and Aretz, Reference Vachard and Aretz2004; Shen and Webb, Reference Shen and Webb2008).
Modern Wetheredella-like bioconstructions are cyanobacterial (Kazmierczak and Kempe, Reference Kaźmierczak and Kempe1992). The modern Wetheredella-like vesicles described by Kazmierczak and Kempe are imperforate and are therefore probably not closely related to Paleozoic Wetheredella to which those authors made comparison. Modern Wetheredella-like vesicles resemble Aphralysia capriorae in form and wall structure, but they are nearly an order of magnitude larger.
Certain Paleozoic fossils referred to as Aphralysia consist of centimeter-scale mounds or clusters of blister-shaped vesicles with microcrystalline or microsparitic carbonate walls. In this, they closely resemble our material. However, the vesicles appear to occupy two distinct size ranges (Table 2). Specimens figured by Vachard et al. (Reference Vachard, Cózar, Aretz and Izart2016, fig. 2E) and Rodríguez (Reference Rodríguez2004, fig. 4C) range to ~ 50 μm in the short dimension (from the relatively flat base to the convex top of the blister). These fossils range in long dimension from ~ 1–3 times the short dimension. Specimens figured by Shen and Webb (Reference Shen and Webb2008, fig. 9A), Garwood (Reference Garwood1914, pl. 21, figs. 3, 4), and Kazmierczak and Kempe (Reference Kaźmierczak and Kempe1992, several figures) range in short dimension from ~ 30–200 μm. Specimens of Aphralysia capriorae in our material range in blister height 10–40 μm, averaging ~ 26 ± 8 μm (Supplementary Data 4). These are comparable in size to the smaller examples of Aphralysia capriorae and similar specimens of Aphralysia found in the published literature (e.g., Adams, Reference Adams1984; Rodríguez, Reference Rodríguez2004; Vachard et al., Reference Vachard, Cózar, Aretz and Izart2016). Other published photographs of Aphralysia cited above show vesicles that are substantially larger than those that we found (Table 2). This consistent difference in size suggests that small and large specimens of Aphralysia of the capriorae type were made by two different kinds of organisms.
Other binding and encrusting problematica
Problematicum A
Figures 6.4, 8.3, 9.3, 9.4, 10.1, 13.4, 13.5, 14.1, 14.3

Figure 14. Thin-section photomicrographs of constituents within the Woodville mound: (1) small oncoid (a) near the periphery of the Woodville mound (dashed line) in direct contact with adjacent oolite, and Problematicum A (b) (thin section GSA-M265); (2) cluster of Problematicum B (a) serving as an ooid nucleus; (3) specimen of Problematicum A (a) incorporated into an oncoid constructed by a consortium of Aphralysia anfracta n. sp. (b) and nonskeletal microbes (thin section GSA-M265); (4) cortex of a small oncoid within the Woodville mound composed mostly of Aphralysia anfracta n. sp. (a); the oncoid nucleus is a fenestellid bryozoan (b) (thin section GSA-M260).
Materials
Thin sections GSA-M257, M258, M261, and M262.
Remarks
The boundstone bodies that make up the Woodville mound incorporated skeletal debris and were themselves syndepositionally encrusted by thin-walled tubes of calcite, which appear white in plane-polarized light. These tubes are 125–250 μm in diameter and are filled with dark microcrystalline carbonate, in some cases, calcite cement. Tube walls are 15–30 μm thick. Tubes vary in shape, ranging in cross section from subrounded to oval to semicircular. Semicircular tubes in some cases show blunt projections in cross section from the curved side extending past the straight side (Fig. 14.3), which are similar to those of Problematicum B (although wall structure is very different). Where the thin-walled tubes are visible in approximately longitudinal section, they are seen to pinch and swell, in some cases resembling a set of connected chambers (Figs. 6.4, 14.1). The walls themselves vary from smooth to roughly covered with sharp to blunt small protrusions on outer surfaces and are smooth on their inner surfaces. A variety of problematic Paleozoic worm tubes have been referred to the tentaculitoids by Taylor et al. (Reference Taylor, Vinn and Wilson2010), which those authors considered to be allied to the lophophorates. Problematicum A superficially resembles one group of these, called the microconchids. However, all but the smallest microconchids are at least several times greater in diameter than any specimens of Problematicum A, and they have much thicker walls. In addition to the size difference, microconchids tend to be either more uniform in diameter or conical; many bear both annulae and longitudinal striations; and spines, where present, tend to be relatively large, stout, and evenly spaced (Vinn and Mutvei, Reference Vinn and Mutvei2005; Taylor and Vinn, Reference Taylor and Vinn2006; Zhan and Vinn, Reference Zhan and Vinn2007; Taylor et al., Reference Taylor, Vinn and Wilson2010; Vinn, Reference Vinn2010; Vinn and Wilson, Reference Vinn and Wilson2010; Wilson et al., Reference Wilson, Vinn and Yancey2010). The affinities of Problematicum A are unknown.
Problematicum B
Figure 14.2
Materials
Thin section GSA-M262.
Remarks
This is a microfossil preserved within an ooid in the ooid grainstone directly beneath the Woodville mound (Fig. 14.2). The ooid nucleus consists primarily of a cluster of calcite vesicles that are individually to 0.15 mm high and to 0.28 mm wide; vesicles are ovoid to hemispherical; many hemispherical vesicles have blunt-pointed projections extending from a convex side past a roughly linear side (Fig. 14.2).
Discussion
The Woodville mound was built by a consortium of organisms (including Aphralysia anfracta n. sp, Aphralysia capriorae, and Problematicum A) strikingly different from those that built any previously described Mississippian mounds or reefs in or near Alabama (Gibson, Reference Gibson1986; Kopaska-Merkel and Haywick, Reference Kopaska-Merkel and Haywick2001, Reference Kopaska-Merkel, Haywick, Puckett and Rindsberg2014; Kopaska-Merkel et al., Reference Kopaska-Merkel, Mann, Pashin, Mancini, Morgan, Ahr, Parcell, Dias-Brito and Harris2013; Fig. 15). Most previously described mounds and reefs contain abundant fenestellid bryozoans, crinoids, and rugose corals, none of which are common in the Woodville mound. The Moulton mound, which is the largest known in Alabama, is a biodetrital structure built by rugose corals, crinoids, and fenestellid bryozoans (Kopaska-Merkel and Haywick, Reference Kopaska-Merkel and Haywick2001). The three small mounds in the Bangor Limestone near Monteagle, Tennessee are the only other buildups that we have examined that plot at the microbial pole on the ternary diagram (Fig. 15). These mounds are crinkly-laminated planar stromatolites. Thin sections reveal that they have been thoroughly dolomitized, and that they contained sponges (Kopaska-Merkel and Haywick, Reference Kopaska-Merkel, Haywick, Puckett and Rindsberg2014). Carboniferous mounds in and near Alabama are united by their diversity, scarcity, and ultimately, their small size. Miniaturization is a well-known indicator of environmental stress in individual organisms (e.g., Urbanek, Reference Urbanek1993; He et al., Reference He, Shi, Feng, Campi, Gu, Bu, Peng and Meng2007). By analogy, miniaturization and reduction in number of organosedimentary structures suggest a poor fit between members of the mound community and prevailing environmental conditions. The skeletal materials that constitute part of the mound differ from macroscopic fauna on the surrounding shelf. For example, fenestellid bryozoans are abundant as ooid nuclei and as noncoated fossil fragments in ooid packstone-grainstone directly beneath the Woodville mound, but few were observed within the mound (Fig. 14.4).
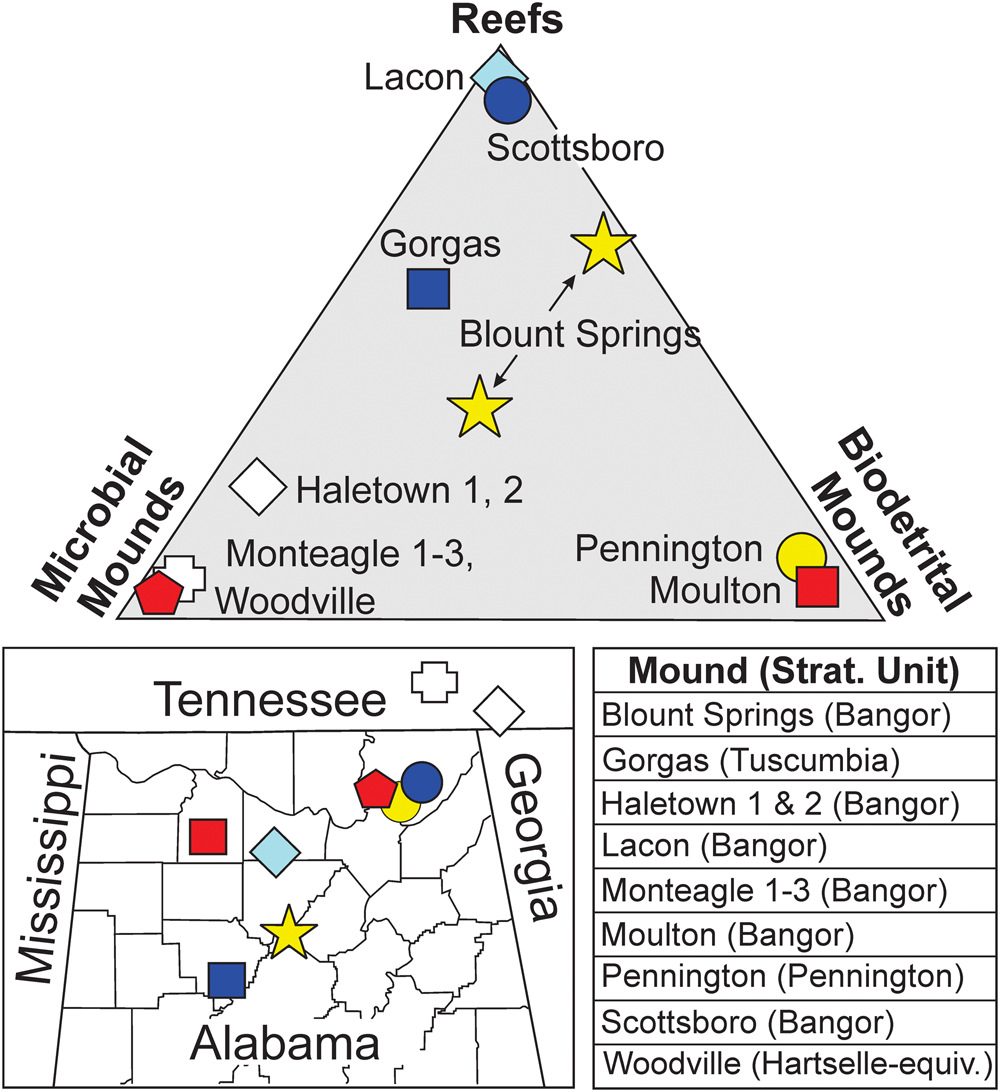
Figure 15. Ternary classification of mound construction, locations and stratigraphic associations of Serpukhovian (Chesterian) reefs, and mounds in and near Alabama. Apices correspond to pure framework reefs, mounds constructed entirely by microbial organisms, and mounds consisting of biodetrital debris. Modified from Kopaska-Merkel and Haywick (Reference Kopaska-Merkel, Haywick, Puckett and Rindsberg2014). Data also from Gibson (Reference Gibson1986), Kopaska-Merkel and Haywick (Reference Kopaska-Merkel and Haywick2001), Kopaska-Merkel et al. (Reference Kopaska-Merkel, Mann, Pashin, Mancini, Morgan, Ahr, Parcell, Dias-Brito and Harris2013), and Haywick et al. (Reference Haywick, Kopaska-Merkel and Keyes2016).
The Woodville mound was a firm structure containing significant void space. It likely began to grow atop a hardground formed during marine cementation of an oolitic grainstone (Fig. 16.1). Carbonate sedimentation occurred intermittently during siliciclastic sedimentation as evidenced by the interbedded limestone-shale strata that compose the Hartselle-equivalent interval in the study area. The Woodville mound arose directly from the seafloor to its full height of just over one meter during a hiatus in sedimentation (Fig. 16.2). Upon resumption of siliciclastic sedimentation, the oolite and mound were both covered by interbedded thin layers of fossil hash and thicker shale beds, terminating skeletal colonization and microbial growth (Fig. 16.3). There is no evidence of siliciclastic material within the constructed portion of the mound. Quartz and possibly detrital dolomite are restricted to third-generation voids. Microencrusters grew on skeletal debris or other encrusters. Early void spaces were filled by carbonate mud or precipitated calcite (Fig. 16.4). Limonitic sediment infiltration of void spaces within the mound and in the underlying oolitic grainstone occurred when deposition on the shallow marine platform was transitioning back to siliciclastic sedimentation (Figs. 5.3, 16.5). The sheer quantity of void-filling carbonate (e.g., Fig. 5.3–5.5) and no indication of collapse of the voids implies that the mound was significantly indurated before the onset of calcareous sandy shale deposition. This is supported by the presence of mound-derived tuberoids in the enclosing sediment. The only evidence of compaction in the field was in the shale layers that were deformed where they made contact with the mound. All evidence leads us to conclude that the Woodville mound arose as a single column from the seafloor and that it was a remarkably porous structure during growth. In this respect, the Woodville mound resembled a classic columnar stromatolite, but with a more diverse microbiota.

Figure 16. Schematic and artist's representations of the Woodville mound: (1–3) summary of the life cycle of the mound from colonization atop an oolite hardground, through maximum growth during an absence of sedimentation, and finally burial during the resumption of siliciclastic mud sedimentation; (4) artistic interpretation of the interior of the Woodville mound during the growth phase; for simplification purposes at this scale, microbial elements are not separately distinguished and nonbiogenic components are reduced to carbonate mud, phase 1/2 sediment fill, and open voids; bryozoans, mollusks, and brachiopods are the only skeletal elements shown in this interpretation; (5) close-up of the boxed area in Figure 16.4, focusing on the microscopic components of the Woodville mound; Aphralysia anfracta n. sp. is the dominant microencruster, coating skeletal elements such as bryozoans (a), forming pendants in what were likely open voids (b), and is also a common component of isolated oncoids (c). Aphralysia capriorae Mamet and Roux, Reference Mamet and Roux1975 (d) also encrusts skeletal elements and forms oncoids. Problematicum A is usually found within areas of carbonate mud (e). The voids in this interpretation are largely empty except for geopetal fill by Phase 1/2 carbonate sediment (f) and small carbonate peloids that are likely the product of microbial processes (g). Other void-filling elements depicted in this interpretation are mud-filled intraskeletal pores within bryozoans (h) and marine (or possibly very early postdepositional) calcite cement within shelter voids (j).
Aphralysia and other microorganisms played important roles in binding lower Carboniferous buildups elsewhere in the world. Reefs in the Visean Cannindah Limestone of Queensland, Australia, contain Aphralysia cf. A. capriorae and other calcimicrobes as binders (Shen and Webb, Reference Shen and Webb2005, fig. 5D; Reference Shen and Webb2008, figs. 9A, 10C). These reefs, which grew on carbonate banks, are much larger and more taxonomically diverse than the Woodville mound (Shen and Webb, Reference Shen and Webb2008). Similarly, Tournaisian reefs in the Gudman Formation of Queensland contain minor amounts of Aphralysia as encrusters and binders. These reefs are considerably larger than the Woodville mound, but, like the latter, nucleated on high-energy, shallow-water carbonate rocks (Webb, Reference Webb2005). Aphralysia was a minor to major component of Visean bioherms in Britain (Garwood, Reference Garwood1914; Adams, Reference Adams1984; review by Webb, Reference Webb1994; Aretz and Herbig, Reference Aretz and Herbig2003), Belgium (Mamet et al., Reference Mamet, Mortelmans and Roux1978), and Spain (Rodriguez, Reference Rodríguez2004; Rododriguez et al., Reference Rododríguez, Arribas, Bermúdez-Rochas, Calvo, Côzar, Falces, Hernando, Mas, Moreno-Eiris, De la Peña, Perejôn, Sanchez-Chico, Somerville and Wong2007; Rodríguez-Martínez et al., Reference Rodríguez-Martínez, Mas and Reitner2010, Reference Rodríguez-Martínez, Moreno-González, Mas and Reitner2012), which grew in settings ranging from platform margin to midramp to protected marine shelf. Species of Aphralysia described by Mamet and Roux (Reference Mamet and Roux1975), collected from Britain and France, range in age from Tournaisian to Serpukhovian, but their paleoenvironmental settings were not described. Visean mounds in Morocco contain encrusting specimens of Aphralysia that were not identified to the species level, but probably belong to Aphralysia anfracta n. sp. (Aretz and Herbig, Reference Aretz and Herbig2008, fig. 10). Aphralysia is a minor component of these mounds, which grew on a high-energy, shallow-marine platform (Aretz and Herbig, Reference Aretz and Herbig2008). Aphralysia occurs in Australia, North America, western Europe, and northern Africa, but is common only locally in Great Britain, Spain, and in the Woodville mound. Aphralysia had a considerable paleogeographic distribution, and ranged throughout the Mississippian (contra Vachard et al., Reference Vachard, Cózar, Aretz and Izart2016), but because it is small and unobtrusive, it can be overlooked in mounds where it is uncommon. Late Chesterian mounds in the Pitkin Formation of Arkansas were deposited across a marine carbonate shelf (Jehn and Young, Reference Jehn and Young1976; Webb, Reference Webb1987). Faunally diverse, these mounds are dominated by thrombolite, but include microbial and metazoan constituents. The Pitkin thrombolite mounds are much larger than the Woodville mound and were probably deposited in deeper water (Jehn and Young, Reference Jehn and Young1976; Webb, Reference Webb1987). Aphralysia has not been reported from the Pitkin mounds, but their age and paleoenvironmental setting are similar to those of reefs and mounds where Aphralysia has been found. Environmental controls that might have excluded Aphralysia from Mississippian mounds that grew on marine carbonate shelves (e.g., Webb, Reference Webb1999; Aretz, Reference Aretz2002) are not yet understood. Younger and older mounds (Devonian and upper Carboniferous) were dominated by different suites of mound-building organisms and generally do not contain Aphralysia (e.g., Krainer et al., Reference Krainer, Flűgel, Vachard and Joachimski2003; Shen and Webb, Reference Shen and Webb2010). One possible Devonian occurrence of Aphralysia is in the Givetian Hanonet-Trois Fontaines formations of Belgium (Mamet and Préat, Reference Mamet and Préat2009, pl. 2, figs. 3, 4). Other reports of Aphralysia from before or after the Mississippian cannot be substantiated on the basis of the evidence given (e.g., Mamet and Boulvain, Reference Mamet and Boulvain1992).
Conclusions
A small carbonate mound is described from siliciclastic-dominated, Hartselle-equivalent strata near Woodville, Alabama. The Woodville mound is the only known carbonate mound in the Serpukhovian (Chesterian) Hartselle-equivalent strata in Alabama, and was built by a previously unknown consortium, including Aphralysia anfracta n. sp., a new species of microproblematicum.
Mound builders included Fistulipora, which provided a distributed structural framework and might have encrusted other mound components, and several encrusting taxa: nonskeletal microbes produced thrombolite (microcrystalline carbonate), which is volumetrically the most abundant encrusting component, and the organisms that made Aphralysia anfracta n. sp. and Aphralysia capriorae were a close second and third. The maker of Problematicum A was a fairly common and widespread encruster.
Minor components of the Woodville mound include cryptic sponges, tiny cryptic microbes, and other organisms that either were trapped in the mound or dwelled there voluntarily (e.g., small gastropods and rare fenestellid bryozoans).
Lower Carboniferous mounds are plentiful in the southeastern USA. Aphralysia, previously unrecognized in this area, could be an important contributor to many of them. Aphralysia has now been recognized as a component of lower Carboniferous, shallow-water, carbonate mounds in Australia, North America, western Europe, and northern Africa. We reconsider the taxonomic affinities of Aphralysia, in light of the new species’ morphology, and conclude that the maker was likely not photosynthetic. This, in turn, has implications for paleoenvironmental interpretation. Although the Woodville mound is small, it can contribute significantly to our understanding of late Paleozoic microbial evolution and bioherm development.
Acknowledgments
We thank the following for advice and other assistance: B. Axsmith, the late R. Folk, B. Granier, R. Hoover, B. Pratt, B. Ray, A. Rindsberg, and D. Vachard, as well as several members of the PaleoNet community. T. Harrell and D. Hills reviewed the manuscript. B. Tew, State Geologist of Alabama, gave permission for publication of this report.
Accessibility of supplementary data
Data available from the Dryad Digital Repository: http://zoobank.org/d3988875-a7fb-4382-bd14-b17c083d87ad.
Supplementary Data 1. Locations of sites referred to in this paper, datum = WGS 84.
Supplementary Data 2. Size measurements of tubules of Aphralysia anfracta n. sp.
Supplementary Data 3. Sample numbers.
Supplementary Data 4. Size measurements of Aphralysia capriorae vesicles in specimen GSA-M265.