Introduction
Significance of databases in research
When conducting experimental investigations, the feasibility of the procedure (whether it be due to financial, spatial, equipment or time constraints), the reaction conditions required, as well as the replicability of the experimental model are all major considerations. These choices need to be made as the experiment is being designed, and it could be inefficient if one has to rely solely on the text of current literature to assist them due to time. A database is useful for experimental planning because it concisely summarizes the information of a multitude of experimental designs, reagent/reaction condition combinations, and has utility to current researchers whether the motive is the replication of a past experiment, or filling in the gaps of current research. Large databases that are sortable by experimental parameters can also facilitate the field's ability to discern bigger pictures and larger chemical trends as evidenced by Barbier et al. (Reference Barbier, Huang, Andreani, Tao, Hao, Eleish, Prabhu, Minhas, Fontaine, Fox and Daniel2020) using the data set published by Huang et al. (Reference Huang, Barbier, Tao, Hao, de Real, Peuble, Merdith, Leichnig, Perrillat, Fontaine, Fox, Andreani and Daniel2020).
In the context of carbon reduction, there are many prebiotic conditions that have not been tested and/or modelled, and there is a plethora of parameters which need be accounted for, as they all have the potential to affect the outcomes of the investigations conducted. This generally makes carbon reduction experiments extremely difficult to conduct, as the equipment needed to recreate hydrothermal conditions, prepare the minerals to be used, and analyse the products (which are often in trace concentrations) can be expensive to obtain and use. In addition, there is field work that has been performed on analogue sites that could be relevant for experimental and modelled tests. Thus, knowing conditions that have and have not been tested and typical experimental setups and product yields to expect is imperative for facilitating efforts of new researchers looking to experimentally explore the field of carbon reduction, particularly in the origin of life field. While the work done by Huang et al. (Reference Huang, Barbier, Tao, Hao, de Real, Peuble, Merdith, Leichnig, Perrillat, Fontaine, Fox, Andreani and Daniel2020) includes 30 papers related to serpentinization, we aimed to design a data set related to experimental, field work, and modelled results within the field of carbon reduction.
The importance of carbon reduction
Carbon is a necessary element for life on Earth and can exist in various oxidized and reduced forms. Abiotically, the movement of carbon through oxidized and reduced phases constitutes the foundational carbon cycle on Earth. A dominant form of carbon on early Earth was carbon dioxide gas (CO2), as it made up the majority of the Hadean atmosphere (Kasting, Reference Kasting1993; Kasting and Catling, Reference Kasting and Catling2003; Trail et al., Reference Trail, Watson and Tailby2011; Armstrong et al., Reference Armstrong, Frost, McCammon, Rubie and Boffa Ballaran2019). CO2 is an oxidized form of carbon as is carbon monoxide (CO), which was also likely present in the early atmosphere, albeit in lower amounts/over shorter time scales (Kasting, Reference Kasting1990; DiSanti et al., Reference DiSanti, Mumma, Russo, Magee-Sauer, Novak and Rettig1999; Zahnle et al., Reference Zahnle, Lupu, Catling and Wogan2020). However, biomass is composed of organic molecules; therefore, if these oxidized gases were important prebiotic carbon sources they would have first had to have been reduced prior to the synthesis of prebiotic molecules like amino acids and nucleobases. Given this, reduction/fixation of CO2 and CO may have served as a significant source of organic molecules on the prebiotic Earth through a variety of mechanisms. The resulting reduced carbon materials (including methane, formaldehyde, methanol, formic acid, acetate and pyruvate) could then have served as starting materials for prebiotic reactions, and their synthesis may have thus been an important process for the origins of life (Butlerov, Reference Butlerov1861; Miller, Reference Miller1953; Nuevo et al., Reference Nuevo, Augar, Blanot and d'Hendecourt2008; Cleaves, Reference Cleaves and Gargaud2011; Kopetzki and Antonietti, Reference Kopetzki and Antonietti2011; McCollom, Reference McCollom2013; Becker et al., Reference Becker, Thoma, Deutsch, Gehrke, Mayer, Zipse and Carell2016; Stubbs et al., Reference Stubbs, Yadav, Krishnamurthy and Springsteen2020; Ruiz et al., Reference Ruiz, Fernández, Ifandi, Eloy, Meza-Trujilo, Devred, Gaigneaux and Tsikouras2021).
Because of this, the fixation or reduction of CO and CO2 has been the focus of a broad body of research in several fields, including planetary science and the origins of life, over the past 50 years. This work spans a significant amount of interdisciplinary research that includes theoretical modelling, laboratory experimentation, field work and analysis of mission data that relates to the origins of life on early Earth or a Martian environment. Modelling is often the focus of this research, and there are limited experimental results due to the challenges related to such work (e.g. high pressures require special reactors, isotopically labelled materials are expensive, and synthesizing pure/contaminant-free minerals is difficult). Experimental research also relies heavily on modelling to deduce which conditions are most promising to explore. In regards to these reactions, there are a number of parameters to investigate (temperature, pressure, pH, mineral source). In order to deduce plausible carbon reduction reactions that could have taken place on early Earth or Mars, it is important that both modelling and experimental work aim to constrain the conditions under which carbon reduction takes place.
We report on a summary of work explored on the reduction of CO and CO2 under geological contexts relevant to Mars and the early Earth. These results are aimed at experimental researchers who are looking for modelled reactions that have not yet been tested in a laboratory setting. However, this table includes modelled results, field work, theoretical studies, data from missions and experimental work and is therefore useful, across a variety of research techniques. In addition, we highlight gaps within the modelling literature that would be fruitful areas for future work. The experimental conditions under which observations took place can be applied to models and modified to different planetary conditions relevant to the search for life by altering the parameters, such as temperature, pressure, phase of the reaction, depth from surface, catalysts used and partial pressure of relevant atmospheric gases to better simulate worlds/environments of interest.
Mechanisms and locations of interest
CO2 has a variety of possible mechanisms for reduction and those mechanisms often depend on the environmental conditions. On the early Earth, atmospheric CO2 would have readily dissolved in the near neutral to mildly acidic oceans (Morse and Mackenzie, Reference Morse and Mackenzie1998; Sleep et al., Reference Sleep, Zahnle and Neuhoff2001; Holland and Turekian, Reference Holland, Turekian and Elderfield2006; Halevy and Bachan, Reference Halevy and Bachan2017; Krissansen-Totton et al., Reference Krissansen-Totton, Arney and Catling2018; MacLeod et al., Reference MacLeod, McKeown, Hall and Russell1994) and existed as a mixture of dissolved CO2 gas, bicarbonate (HCO3−) and carbonate (CO32−) ions. H sources for reducing CO2 to organic molecules could have been derived from atmospheric H2 (predicted to have been at least ~1% of the Hadean atmosphere; Kasting and Catling, Reference Kasting and Catling2003; Tian et al., Reference Tian, Toon, Pavlov and De Sterck2005; Liggins et al., Reference Liggins, Shorttle and Rimmer2020), water/water vapour, trace gases such as H2S, NH4+ and/or CH4. In general, CO2 reduction requires an electron source, an energy source to drive the reaction (typically thermal, electrochemical, radiation or electrical), and often a catalyst or mediator (e.g. Fe0; dissolved metals, iron/zinc minerals, certain organics, etc.); although, large amounts of energy (e.g. electric discharges) can sufficiently drive CO2 reduction in the absence of a catalyst (i.e. Miller–Urey chemistry; Cleaves et al., Reference Cleaves, Chalmers, Lazcano, Miller and Bada2008; Rodriguez et al., Reference Rodriguez, House, Smith, Roberts and Callahan2019). There are various mechanisms by which CO2 or CO can be reduced, but the ones of focus for origins of life research include reverse water–gas shift reactions (forms CO), hydrogenation (forms CH4, CH3OH) or a series of gas-phase reactions such as Miller–Urey chemistry, Fischer Tropsch (FT) reactions, and free radical chain reactions (forms CO, CH4 and hydrocarbons) (Pirronello et al., Reference Pirronello, Brown, Lazerotti, Marcantonio and Simmons1982; Riedel et al., Reference Riedel, Schaub, Jun and Lee2001; Jiang et al., Reference Jiang, Liu, Geng, Xu and Liu2018; Cleaves et al., Reference Cleaves, Chalmers, Lazcano, Miller and Bada2008; Porosoff et al., Reference Porosoff, Yan and Chen2016; Miyakawa et al., Reference Miyakawa, Yamanashi, Kobayashi, Cleaves and Miller2002).
Early Earth atmospheric CO2 could have been reduced via lightning (i.e. Miller–Urey chemistry; Cleaves et al., Reference Cleaves, Chalmers, Lazcano, Miller and Bada2008; Rodriguez et al., Reference Rodriguez, House, Smith, Roberts and Callahan2019) or impact events involving catalytic metals within the impactor (Kasting, Reference Kasting1990; DiSanti et al., Reference DiSanti, Mumma, Russo, Magee-Sauer, Novak and Rettig1999; Sekine et al., Reference Sekine, Sugita, Kadono and Matsui2003; Kress and McKay, Reference Kress and McKay2004; Zahnle et al., Reference Zahnle, Lupu, Catling and Wogan2020); CO2 adsorbed onto catalytic minerals at Earth's surface could have also been reduced if it were subjected to radiation, thermal or electrochemical energy sources (Hudson et al., Reference Hudson, de Graaf, Rodin, Ohno, Lane, McGlynn, Yamada, Nakamura, Barge, Braun and Sojo2020; Li et al., Reference Li, Li, Liu, Wu, Wu, Wang, Ye, Jia, Wang, Li, Zhu, Ding, Lai, Wang, Dick and Lu2020; Tsiotsias et al., Reference Tsiotsias, Charisiou, Yentekakis and Goula2020). CO2 reduction in aqueous solutions is more limited as water often poisons metal catalysts (Porosoff et al., Reference Porosoff, Yan and Chen2016). Carbon reduction in the deep sea (e.g. at deep-sea vents) and deep subsurface sediments is even more restricted considering the lack of sunlight. Thus, at these locales CO2 reduction is driven by either thermal (e.g. via Fischer Tropsch Type reactions; FTT) or electrochemical energy. It is debated to what extent the early Earth would have had land above sea level (Mojzsis et al., Reference Mojzsis, Harrison and Pidgeon2001; Wilde et al., Reference Wilde, Valley, Peck and Graham2001; Kemp et al., Reference Kemp, Wilde, Hawkesworth, Coath, Nemchin, Pidgeon, Vervoort and DuFrane2010; Reimink et al., Reference Reimink, Davies, Chacko, Stern, Heaman, Sarkar, Schaltegger, Creaser and Pearson2016; Hawkesworth et al., Reference Hawkesworth, Cawood and Dhuime2020; Rosas and Korenaga, Reference Rosas and Korenaga2021), so CO2 reduction in the deep sea may have been critical for facilitating abiogenesis events. Of the deep-sea environments, hydrothermal vents are the most promising given that FTT reactions require high temperatures and pressures such as those found at vents; vents also generate strong electrochemical gradients (redox / pH) which, depending on the conditions, can drive CO2 reduction (Martin and Russell, Reference Martin and Russell2007; Martin et al., Reference Martin, Baross, Kelley and Russell2008; Sojo et al., Reference Sojo, Herschy, Whicher, Camprubi and Lane2016). Accordingly, there is a plethora of work which has demonstrated how hydrothermal vents, particularly black smokers and alkaline vents, could generate conditions conducive for CO2 reduction and the formation of biologically relevant compounds including amino acids (Russell et al., Reference Russell, Daniel, Hall and Sherringham1994; Russell and Hall, Reference Russell and Hall1997; Braun and Libchaber, Reference Braun and Libchaber2004; Kelley et al., Reference Kelley, Karson, Früh-Green, Yoerger, Shank, Butterfeild, Hayes, Schrenk, Olson, Proskurowski, Jakauba, Bradley, Larson, Ludwig, Glickson, Buckman, Bradley, Brazelton, Roe, Elend, Delacour, Bernasconi, Lilley, Baross, Summons and Sylva2005; Russell et al., Reference Russell, Barge, Bhartia, Bocanegra, Bracher, Branscomb, Kidd, McGlynn, Meier, Nitschke, Shibuya, Vance, White and Kanik2014; Li et al., Reference Li, Kitadai and Nakamura2018; Barge et al., Reference Barge, Flores, Baum, VanderVelde and Russell2019, Reference Barge, Flores, VanderVelde, Weber, Baum and Castonguay2020; Hudson et al., Reference Hudson, de Graaf, Rodin, Ohno, Lane, McGlynn, Yamada, Nakamura, Barge, Braun and Sojo2020). Consequently, hydrothermal sites have been argued as potentially conducive for abiogenesis on early Earth (Baross and Hoffman, Reference Baross and Hoffman1985; Russell and Hall, Reference Russell and Hall1997; Weiss et al., Reference Weiss, Sousa, Mrnjavac, Neukirchen, Roettger, Nelson-Sathi and Martin2016). Indeed, the Iron Sulphur World hypothesis posits that the iron sulphide minerals at black smoker vents were critical for the origins of life as they not only reduce CO2, but have coordination structures reminiscent of Fe-S clusters in biological metalloenzymes (Wächtershauser, Reference Wächtershauser1990; McGlynn et al., Reference McGlynn, Mulder, Shepard, Broderick and Peters2009; Nitschke et al., Reference Nitschke, McGlynn, Milner-White and Russell2013; White et al., Reference White, Bhartia, Stucky, Kanik and Russell2015; Goldford et al., Reference Goldford, Hartman, Smith and Segrè2017). In addition, alkaline vents have been invoked as potentially relevant for the origins of life given that these systems can generate high temperature and alkaline, H2-rich fluids that are in disequilibrium with the surrounding near-neutral waters; the resulting pH gradients have been invoked as a mechanism for driving CO2 reduction akin to how organisms today produce adenosine triphosphate (ATP) via proton gradients (Russell and Hall, Reference Russell and Hall1997; Kelley et al., Reference Kelley, Baross and Delaney2002; Martin et al., Reference Martin, Baross, Kelley and Russell2008; Tivey, Reference Tivey2007; Sojo et al., Reference Sojo, Herschy, Whicher, Camprubi and Lane2016; Hudson et al., Reference Hudson, de Graaf, Rodin, Ohno, Lane, McGlynn, Yamada, Nakamura, Barge, Braun and Sojo2020). Notably, black smoker vents are significantly more acidic (pH 3–5) and hot (up to 350 °C) compared to alkaline vents (pH ~11; temperature 40–75 °C) (Kelley et al., Reference Kelley, Karson, Blackman, Früh-Green, Butterfield, Lilley, Olson, Schrenk, Roe, Lebon and Rivizzigno2001, Reference Kelley, Karson, Früh-Green, Yoerger, Shank, Butterfeild, Hayes, Schrenk, Olson, Proskurowski, Jakauba, Bradley, Larson, Ludwig, Glickson, Buckman, Bradley, Brazelton, Roe, Elend, Delacour, Bernasconi, Lilley, Baross, Summons and Sylva2005) and as such it has been suggested that organics would be more stable at alkaline than black smoker vents; though, metal-sulphide mineral precipitates that are abundant at black smokers may have higher electrochemical reactivity with CO2 (Roldan et al., Reference Roldan, Hollingsworth, Roffey, Islam, Goodall, Catlow, Darr, Bras, Sankar, Holt, Hogarth and de Leeuw2015; Li et al., Reference Li, Kitadai and Nakamura2018).
Alkaline serpentinite-hosted vents such as Lost City form via serpentinization whereupon oceanic fluids oxidize the iron of minerals, namely olivine and pyroxene, within ultramafic-mafic rocks (i.e. enriched with Mg/Fe and depleted in SiO2 (<45 wt%)) producing a range of secondary mineral phases including magnetite and serpentine (Schulte et al., Reference Schulte, Blake, Hoehler and McCollom2006; Shibuya et al., Reference Shibuya, Yoshizaki, Sato, Shimizu, Nakamura, Omori, Suzuki, Takai, Tsunakawa and Maruyama2015), and magnesite which can be produced by mineral carbonation in CO2-containing systems (Klein and McCollom, Reference Klein and McCollom2013). In addition to being reactive, these materials can mediate organic transformations and are capable of preserving organics through a variety of mechanisms. For example, minerals can preserve organics within sheet structures that traps the organics effectively or organics can adsorb onto the surface of minerals (Farmer and Des Marais, Reference Farmer and Des Marais1999; Bonaccorsi, Reference Bonaccorsi2011).
Perhaps even more important for the habitability on early terrestrial bodies, serpentinization also generates molecular hydrogen (H2) which could have served as an energy source to organisms that may have been present (Schulte et al., Reference Schulte, Blake, Hoehler and McCollom2006). The serpentinization reaction generates heat and hydroxide anions, which can trigger subsequent hydrothermal alteration and precipitation reactions, including the precipitation of brucite, carbonates and iron oxyhydroxides.
Importantly, serpentinization occurs wherever there is ultramafic rock subjected to aqueous alteration – on early Earth such rocks were likely prevalent in the oceanic crust, especially the deep subsurface, given that hotter, younger mantles are more conducive towards generating ultramafic melts as evidenced by Archaean rocks and Martian meteorites, respectively (Griffin et al., Reference Griffin, Belousova, O'Neill, O'Reilly, Malkovets, Pearson, Spetsuis and Wilde2014; Shibuya et al., Reference Shibuya, Yoshizaki, Sato, Shimizu, Nakamura, Omori, Suzuki, Takai, Tsunakawa and Maruyama2015; Santosh et al., Reference Santosh, Arai and Maruyama2017; Drabon et al., Reference Drabon, Byerly, Byerly, Wooden, Keller and Lowe2021). While mafic rocks, namely basalt, have substantially less ferrous mineral content (e.g. pyroxene and olivine) compared to ultramafic rocks, serpentinization and the generation of H2 of mafic rocks can still occur (Stevens and McKinley, Reference Stevens and McKinley2000; Xiong et al., Reference Xiong, Wells, Menefee, Skemer, Ellis and Giammar2017). Studying these reactions via computer modelling and laboratory experiments provides critical analogues for early habitable systems on both Earth and Mars. While serpentinization does not reduce CO2, the resulting high temperatures, H2, and pH gradients from the reaction can drive CO2 reduction (Sleep et al., Reference Sleep, Meibom, Fridriksson, Coleman and Bird2004); thus, serpentinizing systems may have been conducive for origins of life events (Russell et al., Reference Russell, Hall and Martin2010). Serpentinization is suggested to occur in the Martian subsurface (Hand, Reference Hand2009; Brown et al., Reference Brown, Viviano-Beck, Bishop, Cabrol, Andersen, Sobron, Moersch, Templeton and Russell2016; Tarnas et al., Reference Tarnas, Lin, Mustard and Zhang2018a, Reference Tarnas, Mustard, Sherwood Lollar, Bramble, Cannon, Palumbo and Plesa2018b) and other worlds hosting water:rock interactions including Ceres, Europa, and Enceladus (Glein et al., Reference Glein, Baross and Waite2015; Vance et al., Reference Vance, Hand and Pappalardo2016; Castillo-Rogez et al., Reference Castillo-Rogez, Neveu, Scully, House, Quick, Bouquet, Miller, Bland, De Sanctis, Ermakov, Hendrix, Prettyman, Raymond, Russell, Sherwood and Young2020).
The primordial Martian environment can be considered an analogue to early Earth in some ways: it once had a magnetic field (Langlais et al., Reference Langlais, Purucker and Mandea2004), an atmosphere dominated by CO2 with transient periods of no O2 (Sholes et al., Reference Sholes, Smith, Claire, Zahnle and Catling2017), and water flowing over a basaltic crust (Bibring et al., Reference Bibring, Langevin, Mustard, Poulet, Arvidson, Gendrin, Gondet, Mangold, Pinet and Forget2006; Carr, Reference Carr2012). Notably, large portions of the Martian surface that are older than 3.7 Ga (i.e. rocks from the Noachian eon) have been preserved (Bibring et al., Reference Bibring, Langevin, Mustard, Poulet, Arvidson, Gendrin, Gondet, Mangold, Pinet and Forget2006). Given the preservation potential by minerals present on both the early Martian crust and the early Earth, the Martian surface may provide a window to observe abiotic chemistry unimpacted by a biosphere. This could be especially interesting to explore reactions involving CO and CO2 reduction given that organics and minerals that catalyse such reactions have been identified (Michalski et al., Reference Michalski, Onstott, Mojzsis, Mustard, Chan, Niles and Stewart Johnson2018; Liu et al., Reference Liu, Michalski, Tan, He, Ye and Xiao2021).
The importance of carbon cycling on other terrestrial planets beyond Earth, and the implications of that putative carbon cycle for the habitability of those worlds, is still an open question. For at least portions of its history, Mars possessed liquid water, photo and chemical energy sources to power potential microbial metabolisms, water of amenable pH and salinity, and the biogenic elements (carbon, hydrogen, oxygen, nitrogen, phosphorus and sulphur; i.e. CHONPS; Knoll and Grotzinger, Reference Knoll and Grotzinger2006). Indeed, Mars' atmosphere is currently dominated by CO2 (Franz et al., Reference Franz, Trainer, Malespin, Mahaffy, Atreya, Becker, Benna, Conrad, Eigenbrode, Freissinet, Manning, Prats, Raaen and Wong2017), making reduction in the atmosphere a possible pathway relevant to abiogenesis (Heinrich et al., Reference Heinrich, Khare and McKay2007; Franz et al., Reference Franz, Mahaffy, Flesch, Raaen, Freissinet, Atreya, House, McAdams, Knudson, Archer, Stern, Steele, Stutter, Eigenbrode, Glavin, Lewis, Malespin, Millan, Ming, Navarro-González and Summons2020). Furthermore, organic carbon has been detected on the Martian surface as evidenced by the organic content in Martian meteorites and the Sample Analysis at Mars (SAM) instrument (Callahan et al., Reference Callahan, Burton, Elsila, Baker, Smith, Glavin and Dworkin2013; Eigenbrode et al., Reference Eigenbrode, Summons, Steele, Freissinet, Millan, Navarro-González, Sutter, McAdam, Franz, Glavin, Archer, Mahaffy, Conrad, Hurowitz, Grotzinger, Gupta, Ming, Sumner, Szopa, Malespin, Buch and Coll2018; Steele et al., Reference Steele, Benning, Siljeström, Fries, Hauri, Conrad, Rogers, Eigenbrode, Schreiber, Needham, Wang, Mccubbin, Kilcoyne and Rodriguez Blanco2018). By providing a source of organics, this could have sustained extant life on the planet.
Methane was first detected in the Martian atmosphere by Mars Express (Formisano et al., Reference Formisano, Atreya, Encrenaz, Ignatiev and Giuranna2004; Webster et al., Reference Webster, Mahaffy, Atreya, Moores, Flesch, Malespin, McKay, Martinez, Smith, Martin-Torres, Gomez-Elvira, Zorzano, Wong, Trainer, Steele, Archer, Sutter, Coll, Freissinet, Meslin, Gough, House, Pavlov, Eigenbrode, Glavin, Pearson, Keymeulen, Christensen, Schwenzer, Smith, Harri, Genzer, Hassler, Lemmon, Crisp, Sander, Zurek and Vasavada2018; Yung et al., Reference Yung, Chen, Nealson, Atreya, Beckett, Blank, Ehlmann, Eiler, Etiope, Ferry, Forget, Gao, Hu, Kleinböhl, Klusman, Lefèvre, Miller, Mischna, Mumma, Newman, Oehler, Okumura, Oremland, Orphan, Popa, Russell, Shen, Sherwood Lollar, Staehle, Stamenković, Stolper, Templeton, Vandaele, Viscardy, Webster, Wennberg, Wong and Worden2018) at levels near the instrumental detection limit. The Curiosity rover recently observed methane in the vicinity of Gale Crater on Mars (Webster et al., Reference Webster, Mahaffy, Atreya, Flesch, Mischna, Meslin, Farley, Conrad, Christensen, Pavlov, Martín-Torres, Zorzano, McConnochie, Owen, Eigenbrode, Glavin, Steele, Malespin, Archer, Sutter, Coll, Freissinet, McKay, Moores, Schwenzer, Bridges, Navarro-Gonzalez, Gellert and Lemmon2015; Eigenbrode et al., Reference Eigenbrode, Summons, Steele, Freissinet, Millan, Navarro-González, Sutter, McAdam, Franz, Glavin, Archer, Mahaffy, Conrad, Hurowitz, Grotzinger, Gupta, Ming, Sumner, Szopa, Malespin, Buch and Coll2018; Giuranna et al., Reference Giuranna, Viscardy, Daerden, Neary, Etiope, Oehler, Formisano, Aronica, Wolkenberg, Aoki, Cardesín-Moinelo, Marín-Yaseli de la Parra, Merritt and Amoroso2019). However, the Trace Gas Orbiter has not detected any atmospheric methane despite having a much lower detection limit, leading to an inconsistency yet to be resolved (Korablev et al., Reference Korablev, Vandaele, Montmessin, Fedorova, Trokhimovskiy, Forget, Lefèvre, Daerden, Thomas, Trompet, Erwin, Aoki, Robert, Neary, Viscardy, Grigoriev, Ignatiev, Shakun, Patrakeev, Belyaev, Bertaux, Olsen, Baggio, Alday, Ivanov, Ristic, Mason, Willame, Depiesse, Hetey, Berkenbosch, Clairquin, Queirolo, Beeckman, Neefs, Patel, Bellucci, López-Moreno, Wilson, Etiope, Zelenyi, Svedham and Vago2019). While methane detections remain hotly debated, the SAM instrument aboard Curiosity has detected a suite of other organics, as well, including chlorinated organics (e.g. chloromethane, dichloromethane, chlorobenzene) and S-bearing organics (both aliphatic and aromatic) (Mahaffy et al., Reference Mahaffy, Webster, Cabane, Conrad, Coll, Atreya, Arvey, Barciniak, Benna, Bleacher, Brinckerhoff, Eigenbrode, Carignan, Cascia, Chalmers, Dworkin, Errigo, Everson, Franz, Farley, Feng, Frazier, Freissinet, Glavin, Harpold, Hawk, Holmes, Johnson, Jones, Jordan, Kellogg, Lewis, Lyness, Malespin, Martin, Maurer, McAdam, McLennan, Nolan, Noriega, Pavlov, Prats, Raaen, Sheinman, Sheppard, Smith, Stern, Tan, Trainer, Ming, Morris, Jones, Gundersen, Steele, Wray, Botta, Leshin, Owen, Battel, Jakosky, Manning, Squyres, Navarro-González, McKay, Raulin, Sternberg, Buch, Sorensen, Kline-Schoder, Coscia, Szopa, Teinturier, Baffes, Feldman, Flesch, Forouhar, Garcia, Keymeulen, Woodward, Block, Arnett, Miller, Edmonson, Gorevan and Mumm2012, Glavin et al., Reference Glavin, Freissinet, Miller, Eigenbrode, Brunner, Buch, Sutter, Archer, Atreya, Brinckerhoff, Cabane, Coll, Conrad, Coscia, Dworkin, Reanz, Grotzinger, Leshin, Martin, McKay, Ming, Navarro-González, Pavlov, Steele, Summons, Szopa, Teinturier and Mahaffy2013, Williams et al., Reference Williams, Eigenbrode, Floyd, Wilhelm, O'Reilly, Stewart Johnson, Craft, Knudson, Andrejkovičová, Lewis, Buch, Glavin, Freissinet, Willians, Szopa, Millan, Summons, McAdam, Benison, Navarro-González, Malespin and Mahaffy2019; Millan et al., Reference Millan, Williams, McAdam, Eigenbrode, Freissinet, Glavin, Szopa, Buch, Williams, Navarro-Gonzalez, Lewis, Fox, Bryk, Bennet, Steele, Teinturier, Malespin, Johnson and Mahaffy2021). The mechanism by which all these Martian organics were generated remains uncertain (Miller et al., Reference Miller, Eigenbrode, Freissinet, Glavin, Kotrc, Francois and Summons2016; Szopa et al., Reference Szopa, Freissinet, Glavin, Millan, Buch, Franz, Summons, Sumner, Sutter, Eigenbrode and Williams2020). One possibility is that they were derived from reduced gases, such as H2 and CH4, which have been hypothesized to have originated from various sources including serpentinization of ultramafic minerals such as olivine, subsurface hydrothermal environments, photocatalysis and volcanic activity (Sherwood Lollar et al., Reference Sherwood Lollar, Lacrampe-Couloume, Slater, Ward, Moser, Gihring, Lin and Onstott2006; Amador et al., Reference Amador, Bandfield and Thomas2018; Tarnas et al., Reference Tarnas, Lin, Mustard and Zhang2018a, Reference Tarnas, Mustard, Sherwood Lollar, Bramble, Cannon, Palumbo and Plesa2018b). Methane on Mars can be hypothetically produced by biological reactions (Boston et al., Reference Boston, Ivanov and McKay1992; Weiss et al., Reference Weiss, Yung and Nealson2000) or produced abiotically by water-rock reactions (Wallendahl and Treimann, Reference Wallendahl and Treimann1999; Max and Clifford, Reference Max and Clifford2000), volcanic outgassing (Wong et al., Reference Wong, Atreya and Encrenaz2003) or even exogenous delivery such as comets (Kress and McKay, Reference Kress and McKay2004). Further complicating this, methane is easily trapped in the subsurface, persisting in minerals or gas pockets through geologic time (Max and Clifford, Reference Max and Clifford2000). On Mars, methane could have been produced recently or trapped in the ice-bearing subsurface in the Noachian or Hesperian as the planet cooled (Kerr, Reference Kerr2004). The non-uniform atmospheric detections of methane in the Martian atmosphere are indicative of localized sources and/or localized sinks (Formisano et al., Reference Formisano, Atreya, Encrenaz, Ignatiev and Giuranna2004), emphasizing the need for more precise constraints on the reactions creating or uptaking Martian methane (Fig. 1).

Fig. 1. Plausible mechanisms for CO2 reduction on early Earth or Mars. (A) General CO2 reduction reaction requires a H source (likely H2 or H2O), energy (e.g. thermal, radiation, electric discharge or redox gradient), and some sort of catalyst or mediator (e.g. Fe0, Ni2+, magnetite). Common products of this reaction include CO (which can be further reduced) and CH4 (unstable to photolytic degradation) with organics usually produced in lower amounts (methanol, formaldehyde, formic acid and acetic acid are generally produced and are shown). (B) Locations of interest for CO2 reduction on early Earth or Mars: reduction via Miller–Urey chemistry with (1) H2 as a H donor or (2) H2O as a H donor generates a range of organics (Cleaves, Reference Cleaves2008); (3) impactors containing catalytic transition metals can facilitate CO2 reduction (e.g. Civiš et al., Reference Civiš, Szabla, Szyja, Smykowski, Ivanek, Knížek, Kubelík, Šponer, Ferus and Šponer2016; Steele et al., Reference Steele, Benning, Siljeström, Fries, Hauri, Conrad, Rogers, Eigenbrode, Schreiber, Needham, Wang, Mccubbin, Kilcoyne and Rodriguez Blanco2018); (4) CO2 dissolution via precipitation or with equilibrium with bodies of water produces carbonic acid, bicarbonate and carbonate ions; (5) reduction of CO2 adsorbed onto catalytic minerals such as anatase (which contains TiO2) via photolysis (e.g. Knížek et al., Reference Knížek, Kubelík, Bouša, Ferus and Civiš2020); (6) UV irradiation of CO2 generates reduced C species; (7) black smoker hydrothermal vents, (8) alkaline vents such as those at Lost City, (e.g. Hudson et al., Reference Hudson, de Graaf, Rodin, Ohno, Lane, McGlynn, Yamada, Nakamura, Barge, Braun and Sojo2020; Preiner et al., Reference Preiner, Igarashi, Muchowska, Yu, Varma, Kleinermanns, Nobu, Kamagata, Tüysüz, Moran and Martin2020). (9) serpentinization in the deep subsurface can generate conditions conducive for CO2 reduction (e.g. Etiope et al., Reference Etiope, Schoell and Hosgörmez2011; Preiner et al., Reference Preiner, Xavier, Sousa, Zimorski, Neubeck, Lang, Greenwell, Kleinermanns, Tüysüz, Micollom, Holm and Martin2018). *CO2 indicates a mixture of dissolved CO2 gas or bicarbonate/carbonate anions.
The source and production of reduced carbon on Earth and its availability on other worlds are not well constrained. Carbon reduction can give a variety of reduced products depending on the complex geological context (Schrenk et al., Reference Schrenk, Brazelton and Lang2013). In addition, there is limited access to extraterrestrial samples, which would help constrain the geologic context as well as provide insight to the chemical reactions occurring. CO and CO2 could both be sources for reduced carbon via several reaction pathways as both gases would have been available in the atmosphere and are more stable in comparison to methane (Kasting et al., Reference Kasting, Zahnle and Walker1983; Kasting, Reference Kasting1993; Kasting and Catling, Reference Kasting and Catling2003; Sherwood Lollar et al., Reference Sherwood Lollar, Lacrampe-Couloume, Slater, Ward, Moser, Gihring, Lin and Onstott2006).
Methods
We have generated a detailed dataset enumerating the research performed in the field of CO and CO2 reduction. We mainly focus on mineral catalysis of CO2 reduction in the context of early Earth and methane production on Mars, including serpentinization. Papers were identified via Google Scholar searches, using search phrases such as ‘carbon fixation,’ ‘methane on Mars,’ ‘organics on Mars,’ ‘serpentinization on Mars,’ ‘carbon monoxide reduction on early Earth,’ etc. The works reviewed span as early as 1979, however most of them are concentrated in the beginning of the 21st century until present. The table was primarily organized by the relevant mineral/catalyst for the reaction and to be sortable by individual minerals; therefore, papers discussing multiple minerals will occupy multiple rows in the table. We considered the carbon starting material, the reaction that occurred (primarily reduction), the end products that ensued, whether the research of relevance was experimental or model-based, as well as the relevance to Mars and early Earth. As more information was gathered, we added in fields pertaining to reaction conditions, such as temperature and pressure, the phase of the reactants, the source of the mineral, the type of reaction occurring, involvement of spectroscopic measurements and isotopic analysis, and if the reaction was low yielding or not – although in many papers, the aforementioned categories were not relevant and/or specified. If the entry did not apply to one of the columns or if the information was not specified, N/A was put in that slot.
The preliminary literature review performed here included 40 papers (Calvert and Steacie, Reference Calvert and Steacie1951; Kelley, Reference Kelley1996; Heinen and Lauwers, Reference Heinen and Lauwers1997; Horita and Berndt, Reference Horita and Berndt1999; Guan et al., Reference Guan, Kida, Ma, Kimura, Abe and Yoshida2003; McCollom and Seewald, Reference McCollom and Seewald2003; Foustoukos and Seyfried, Reference Foustoukos and Seyfried2004; Lyons et al., Reference Lyons, Manning and Nimmo2005; Oze and Sharma, Reference Oze and Sharma2005; Seyfried et al., Reference Seyfried, Foustoukos and Fu2007; Cleaves, Reference Cleaves2008; Ji et al., Reference Ji, Zhou and Yang2008; Mulkidjanian, Reference Mulkidjanian2009; He et al., Reference He, Tian, Liu and Feng2010; Lang et al., Reference Lang, Butterfield, Schulte, Kelley and Lilley2010; Etiope et al., Reference Etiope, Schoell and Hosgörmez2011; Lindgren et al., Reference Lindgren, Parnell, Holm and Broman2011; Steele et al., Reference Steele, McCubbin, Fries, Kater, Boctor, Conrad, Clamoclija, Spencer, Morrow, Hammond, Zare, Vicenzi, Siljeström, Bowden, Herd, Mysen, Shirly, Amundsen, Treiman, Bullock and Jull2012; Barge et al., Reference Barge, Cardoso, Cartwright, Doloboff, Flores, Macías-Sánchez, Sainz-Díaz and Sobrón2016; Etiope et al., Reference Etiope, Ehlmann and Schoell2013; Schouten et al., Reference Schouten, Gallent and Koper2013; Yamaguchi et al., Reference Yamaguchi, Yamamoto, Takai, Ishii, Hashimoto and Nakamura2014; Batalha et al., Reference Batalha, Domagal-Goldman, Ramirez and Kasting2015; Civiš et al., Reference Civiš, Szabla, Szyja, Smykowski, Ivanek, Knížek, Kubelík, Šponer, Ferus and Šponer2016; Civiš et al., Reference Civiš, Knížek, Ivanek, Kubelík, Zukalová, Kavan and Ferus2017; Miller et al., Reference Miller, Mayhew, Ellison, Kelemen, Kubo and Templeton2017; Neto-Lima et al., Reference Neto-Lima, Fernández-Sampedro and Prieto-Ballesteros2017; Santos-Carballal et al., Reference Santos-Carballal, Roldan, Dzade and de Leeuw2017; Ueda et al., Reference Ueda, Sawaki and Maruyama2017; Eigenbrode et al., Reference Eigenbrode, Summons, Steele, Freissinet, Millan, Navarro-González, Sutter, McAdam, Franz, Glavin, Archer, Mahaffy, Conrad, Hurowitz, Grotzinger, Gupta, Ming, Sumner, Szopa, Malespin, Buch and Coll2018; Preiner et al., Reference Preiner, Xavier, Sousa, Zimorski, Neubeck, Lang, Greenwell, Kleinermanns, Tüysüz, Micollom, Holm and Martin2018; Steele et al., Reference Steele, Benning, Siljeström, Fries, Hauri, Conrad, Rogers, Eigenbrode, Schreiber, Needham, Wang, Mccubbin, Kilcoyne and Rodriguez Blanco2018; Tosca et al., Reference Tosca, Ahmen, Tutolo, Ashpitel and Hurowitz2018; Varma et al., Reference Varma, Muchowska, Chatelain and Moran2018; Yung et al., Reference Yung, Chen, Nealson, Atreya, Beckett, Blank, Ehlmann, Eiler, Etiope, Ferry, Forget, Gao, Hu, Kleinböhl, Klusman, Lefèvre, Miller, Mischna, Mumma, Newman, Oehler, Okumura, Oremland, Orphan, Popa, Russell, Shen, Sherwood Lollar, Staehle, Stamenković, Stolper, Templeton, Vandaele, Viscardy, Webster, Wennberg, Wong and Worden2018; Civiš et al., Reference Civiš, Knížek, Rimmer, Ferus, Kublelík, Zukalová, Kavan and Chatzitheodoridis2019; Knížek et al., Reference Knížek, Kubelík, Bouša, Ferus and Civiš2020; Preiner et al., Reference Preiner, Igarashi, Muchowska, Yu, Varma, Kleinermanns, Nobu, Kamagata, Tüysüz, Moran and Martin2020; Liu et al., Reference Liu, Michalski, Tan, He, Ye and Xiao2021; Ruiz et al., Reference Ruiz, Fernández, Ifandi, Eloy, Meza-Trujilo, Devred, Gaigneaux and Tsikouras2021), particularly in the area of carbon phases and fixation processes. We realize this database is not perfectly comprehensive. Rather, our goal was to develop a downloadable resource of maximum utility to current researchers that could be appended and expanded as new findings emerge in the field. In addition, we specifically excluded papers without geological context (i.e. papers focused on catalysis for industrial, material or pharmaceutical purposes) and instead focused on papers that were relevant for Mars or the early Earth. CO2 reduction is certainly important in other fields (e.g. industrial processes). While some of these papers also utilize mineral material, (Wei et al., Reference Wei, Ge, Yao, Wen, Fang, Guo, Xu and Sun2017), we have chosen to focus on papers with a direct planetary context. We believe as such that this dataset presents the highlights of this area, as well as important directions for future research.
We categorized these papers by columns that are searchable (Table 1). Importantly, we distinguished between experimental and theoretical, mission based, modelled and field results. We also included relevant field work in the table, including studies done on the Chimaera Seep to understand methane flux (Etiope et al., Reference Etiope, Schoell and Hosgörmez2011). Recognizing if the research was based on models or experiments helps identify where further investigations could be conducted. Reaction conditions such as temperature, pH and pressure help elucidate which geologic setting would be most relevant to the experiment (e.g. hydrothermal vents, hydrothermal hot springs, the deep subsurface or past Martian environments). The database also summarizes product yields for each of the reactions to facilitate comparisons between the various reactions and to determine whether such chemistry could have generated significant or only trace amounts of the reduced carbon products. The mineral source was also noted to identify whether the mineral was synthetic or natural (thus having impurities, e.g. methane/hydrocarbons) which could contribute to reduced carbon compounds identified in the reaction. Indeed, previous work using isotopically labelled 13CO2 found that the majority of methane and carbon compounds formed in reactions using natural olivine samples were not derived from the starting 13CO2 but from methane/hydrocarbon contaminants within the mineral (McCollom and Seewald, Reference McCollom and Seewald2001; McCollom, Reference McCollom2016). Given that serpentinization may not generate as much reduced carbon compounds as previously thought, we also noted whether reactions used isotopically labelled reactants to rule out the role of contaminants. Furthermore, a field for spectroscopic measurements was included to clarify how products were identified. The phase (liquid, gas) of the reaction is also important as aqueous environments affect how substrates bind to catalysts, consequently the products yielded, as well as the relevance to hydrothermal vents as a possible site for the origins of life (e.g. Martin et al., Reference Martin, Baross, Kelley and Russell2008; Russell et al., Reference Russell, Hall and Martin2010). Fields classifying the papers as relevant to the discussions of Mars and Early Earth (or both) were also included so researchers using this table can consolidate papers of reference, as we want this database to be searchable and sortable. Miscellaneous notes were also added as each paper has exceptions and every single aspect of every single paper cannot be compartmentalized into individual cells on a spreadsheet without it losing its efficiency and a reasonable organization.
Table 1. List of column categories that were used in the database and their purpose

We have included an illustrative subset of the table as tables in this manuscript (Tables 1, 2 and 3). The complete table is available for download as supplemental information.
Table 2. Common products, reactants and minerals identified within the database

Table 3. Row of database corresponding to Roldan et al. (Reference Roldan, Hollingsworth, Roffey, Islam, Goodall, Catlow, Darr, Bras, Sankar, Holt, Hogarth and de Leeuw2015)
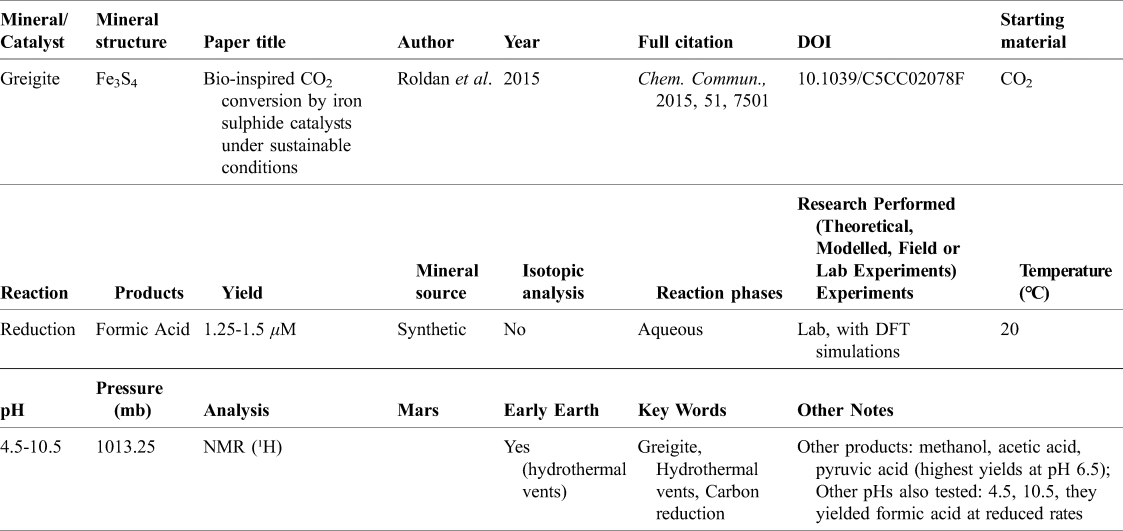
Results
A summary of the reagents and minerals used in the papers we surveyed is detailed in Table 2. We observed that iron minerals, iron-nickel-sulphides, iron-nickel alloys, titanium and magnesium compounds were commonly invoked in these reactions. The majority of the aforementioned minerals were synthetically sourced or not specified in the source when used in the experiments. There are larger amounts of literature relating to CO2 reduction as opposed to CO reduction. Isotopic analysis of 13C was often not mentioned or not conducted in the experimental designs of the included investigations. In general, it has been found that the reduction of CO2 and CO under early Earth or Mars relevant conditions has resulted in a variety of products, including formate, formaldehyde methanol, methane and acetate (Table 2). In general, methane and formate are the most dominant products formed in the database. Yields were not always reported or relevant but, in most cases, they range from the nanomolar to millimolar. In addition, the yields reported were not always constrained in the context of the quantity of the reactants, making it difficult to compare across different research papers. Often, the mechanisms are only identified as reduction, but the Sabatier reaction (i.e. Etiope et al., Reference Etiope, Schoell and Hosgörmez2011, Reference Etiope, Ehlmann and Schoell2013; Ruiz et al., Reference Ruiz, Fernández, Ifandi, Eloy, Meza-Trujilo, Devred, Gaigneaux and Tsikouras2021), serpentinization (i.e. Preiner et al., Reference Preiner, Xavier, Sousa, Zimorski, Neubeck, Lang, Greenwell, Kleinermanns, Tüysüz, Micollom, Holm and Martin2018) and FTT synthesis (i.e. Etiope et al., Reference Etiope, Schoell and Hosgörmez2011; Yung et al., Reference Yung, Chen, Nealson, Atreya, Beckett, Blank, Ehlmann, Eiler, Etiope, Ferry, Forget, Gao, Hu, Kleinböhl, Klusman, Lefèvre, Miller, Mischna, Mumma, Newman, Oehler, Okumura, Oremland, Orphan, Popa, Russell, Shen, Sherwood Lollar, Staehle, Stamenković, Stolper, Templeton, Vandaele, Viscardy, Webster, Wennberg, Wong and Worden2018) are also specifically identified. Papers discussing precipitation and adsorption are also included (Santos-Carballal et al., Reference Santos-Carballal, Roldan, Dzade and de Leeuw2017; Tosca et al., Reference Tosca, Ahmen, Tutolo, Ashpitel and Hurowitz2018).
The table also includes work that shows reduced organics that could have been products of carbon fixation have been detected on Mars along with reactive minerals. Experimental procedures included methods such as gas chromatography mass spectrometry (GC-MS), cross-track infrared sounder (CRIS) and nuclear magnetic resonance (NMR), as well as viewing terrestrial analogues, often combined with modelling and computational chemistry simulations. Most of the spectroscopic methods utilized were in the context of analysis of the mineral used for catalysis or involved in serpentinization as opposed to analysis of the product. Spectroscopic methods were more likely to be applied to minerals of natural source such as Martian meteorites or montmorillonites as opposed to synthetic ones. Many papers rarely noted other environmental factors and their effects on the yields of different products in detail, such as salinity and fugacity. These papers identify possible mechanisms for the emergence of methane (including volcanic outgassing and photocatalysis) and hydrogen on Mars (including serpentinization).
Shown in Table 3 is an example row included in the database. This paper used greigite as the mineral of study, an iron sulphide often cited in the context of hydrothermal vents (Roldan et al., Reference Roldan, Hollingsworth, Roffey, Islam, Goodall, Catlow, Darr, Bras, Sankar, Holt, Hogarth and de Leeuw2015). Therefore, this paper is very relevant to carbon fixation on the early Earth and is marked as such in the database. In this paper, Roldan et al. (Reference Roldan, Hollingsworth, Roffey, Islam, Goodall, Catlow, Darr, Bras, Sankar, Holt, Hogarth and de Leeuw2015) performed both a lab experiment as well as some modelling through Density Functional Theory (DFT) simulations. The starting material used was CO2 and the primary product of the reduction reaction was formic acid. The reaction was tested at pH 4.5, 6.5 and 10.5 at room temperature and pressure. It took place in an aqueous environment and the mineral was synthetic. Other reduced products identified included methanol, acetic acid and pyruvic acid, all of which were formed in the highest quantities at a pH of 6.5, similar to formic acid. The reactions were analysed with NMR to determine the organic products. By having the reactions of different minerals laid out in a database, these reaction conditions, analysis techniques, and yields can be directly compared to other works and can be fit into a broader context within the other studies that have been done in this area.
A variety of the minerals, including iron sulphides, that have been shown in the research examined to catalyse carbon reduction are relevant to hydrothermal vent systems which are of particular interest to the origins of life community. Hydrothermal vents are high-pressure, sometimes high-temperature environments that are composed of metal sulphides (for black smokers, e.g. greigite) and hydroxides (in both black smokers and alkaline vents), which can be reactive sites. Some minerals such as greigite resemble structures in modern carbon dehydrogenase (CODH) enzymes found in archaeabacteria and other iron-sulphur cluster enzymes (Russell and Hall, Reference Russell and Hall1997; Russell and Martin, Reference Russell and Martin2004; Nitschke et al., Reference Nitschke, McGlynn, Milner-White and Russell2013; Roldan et al., Reference Roldan, Hollingsworth, Roffey, Islam, Goodall, Catlow, Darr, Bras, Sankar, Holt, Hogarth and de Leeuw2015), which could make them especially relevant to the development of protometabolic or metabolic cycles (Kitadai et al., Reference Kitadai, Nakamura, Yamamoto, Yoshita and Oono2019; Zhao et al., Reference Zhao, Bartlett and Yung2020). Similar in structure to the Fe-S minerals, iron-nickel-sulphide minerals (such as violarite) were also seen as effective mediators in laboratory experiments (i.e. Yamaguchi et al., Reference Yamaguchi, Yamamoto, Takai, Ishii, Hashimoto and Nakamura2014; Roldan et al., Reference Roldan, Hollingsworth, Roffey, Islam, Goodall, Catlow, Darr, Bras, Sankar, Holt, Hogarth and de Leeuw2015; Santos-Carballal et al., Reference Santos-Carballal, Roldan, Dzade and de Leeuw2017; Hudson et al., Reference Hudson, de Graaf, Rodin, Ohno, Lane, McGlynn, Yamada, Nakamura, Barge, Braun and Sojo2020). Pressures and temperatures varied as experiments were carried out in simulated hydrothermal conditions, sometimes influencing the yield of various products (Neto-Lima et al., Reference Neto-Lima, Fernández-Sampedro and Prieto-Ballesteros2017).
Discussion and implications for future research
This dataset was designed to be useful for both experimentalists and modellers. It gives a general overview of carbon reduction research in relation to Mars and the origins of life on Earth and indicates what conditions have already been tested or modelled. The table also allows for easy identification of conditions that have been modelled but not experimentally explored, which could be a place for expanded research. The table identifies reactive minerals of interest within the field, and these minerals can be explored in reactions including a form of carbon reduction.
More laboratory / experimental research should be designed, especially those to utilizing isotopic labelling. This could be constrained by modelling studies which was significantly more covered at least in the 39 studies we included in the preliminary database. For the laboratory studies, in many cases either the mineral material is significantly analysed or only the organic material is analysed; we recommend performing more mineralogy studies when exploring the carbon reactivity. Carbon reduction at more extreme pH measurements is understudied. Also, connecting the field work to laboratory experiments would be helpful in expanding the understanding of carbon reduction for the early Earth and Mars. CO2 reduction has also been better explored in this database in comparison to CO. Similar to work by Barbier et al. (Reference Barbier, Huang, Andreani, Tao, Hao, Eleish, Prabhu, Minhas, Fontaine, Fox and Daniel2020) on the Huang et al. (Reference Huang, Barbier, Tao, Hao, de Real, Peuble, Merdith, Leichnig, Perrillat, Fontaine, Fox, Andreani and Daniel2020) dataset, a variety of machine learning techniques could be utilized to further analyse the dataset to identify general trends and important factors.
As noted in our findings, the exact mechanism of carbon reduction in many cases is not well understood or specified in the results (and often just written as ‘reduction’ in the database). Understanding the different forms of reduction is critical and we recommend further models and experimental research to improve the understanding of how the C material interacts with mineral species. Related to the reduction mechanism, the nature of how the carbon interfaces within hydrothermal vents is not well defined (Martin et al., Reference Martin, Baross, Kelley and Russell2008). In addition, the effects of heterogeneous catalysts have not been fully examined and this could be addressed with additional research of the mechanism.
Methane on Mars has been detected, but the origins of it and hydrogen are still debated, as photocatalysis, volcanic outgassing, serpentinization, magnetite authigenesis, silicate cataclasis, and other processes are potential options (Webster et al., Reference Webster, Mahaffy, Atreya, Flesch, Mischna, Meslin, Farley, Conrad, Christensen, Pavlov, Martín-Torres, Zorzano, McConnochie, Owen, Eigenbrode, Glavin, Steele, Malespin, Archer, Sutter, Coll, Freissinet, McKay, Moores, Schwenzer, Bridges, Navarro-Gonzalez, Gellert and Lemmon2015; Eigenbrode et al., Reference Eigenbrode, Summons, Steele, Freissinet, Millan, Navarro-González, Sutter, McAdam, Franz, Glavin, Archer, Mahaffy, Conrad, Hurowitz, Grotzinger, Gupta, Ming, Sumner, Szopa, Malespin, Buch and Coll2018; Yung et al., Reference Yung, Chen, Nealson, Atreya, Beckett, Blank, Ehlmann, Eiler, Etiope, Ferry, Forget, Gao, Hu, Kleinböhl, Klusman, Lefèvre, Miller, Mischna, Mumma, Newman, Oehler, Okumura, Oremland, Orphan, Popa, Russell, Shen, Sherwood Lollar, Staehle, Stamenković, Stolper, Templeton, Vandaele, Viscardy, Webster, Wennberg, Wong and Worden2018). However, spectroscopic measurements for the mineralogy of the planet, and modelling has been the main source of information or prediction for the redox state of the Martian crust and mantle (Batalha et al., Reference Batalha, Domagal-Goldman, Ramirez and Kasting2015; Liu et al., Reference Liu, Michalski, Tan, He, Ye and Xiao2021; Tosca et al., Reference Tosca, Ahmen, Tutolo, Ashpitel and Hurowitz2018). Serpentinization in the context of methane production on Mars, the role of serpentinized minerals, and the Mars subsurface conditions need to be further investigated. Future missions to Mars could be devoted to examining abiotic chemistry on early Mars, possibly in the Northern lowlands which was once hypothesized to be an ancient ocean (Liu et al., Reference Liu, Michalski, Tan, He, Ye and Xiao2021). Such an investigation is not only beneficial to understanding the geologic history and water inventory on early Mars, but it might provide a reasonable analogue to early Earth. The nature of filling the geochemical gaps of the emergence of life, with limited access to relevant environments and geology, sparks debate and conflicting evidence depending on the papers that are read. We hope that tables of this format allow larger trends to be seen, and can be utilized in conjunction with other datasets (e.g. Barbier et al., Reference Barbier, Huang, Andreani, Tao, Hao, Eleish, Prabhu, Minhas, Fontaine, Fox and Daniel2020; Huang et al., Reference Huang, Barbier, Tao, Hao, de Real, Peuble, Merdith, Leichnig, Perrillat, Fontaine, Fox, Andreani and Daniel2020).
Laboratory studies can help contextualize mission work by providing baseline information. However, in situ subsurface and chemical measurements would help address open questions about methane production on Mars. The summary table presented here helps identify established or hypothetical conditions, including data that researchers report that they need. In addition to helping laboratory researchers, this table can help direct the future and ongoing missions to Mars, including sampling protocol and traverse routes that could answer critical open questions. For example, the Perseverance rover is the first step in a proposed Mars Sample Return programme (Farley et al., Reference Farley, Williford, Stack, Bhartia, Chen, de la Torre, Hand, Goreva, Herd, Hueso, Liu, Maki, Martinez, Moeller, Nelessen, Newman, Nunes, Ponce, Spanovich, Willis, Beegle, Bell, Brown, Hamran, Hurowitz, Maurice, Paige, Rodriguez-Manfredi, Schulte and Wiens2020), and its proximity to Nili Fossae, a hypothesized exposed serpentinizing system (Ehlmann et al., Reference Ehlmann, Mustard, Swayze, Clark, Bishop, Poulet, Des Marais, Roach, Milliken, Wray, Barnouin-Jha and Murchie2009, Reference Ehlmann, Mustard and Murchie2010), may provide future insight to how these processes occur on Mars. In addition, ESA's ExoMars rover will have a 2-meter drill that can retrieve subsurface sediment samples and analyse them with GC-MS, Raman, and NIR spectroscopy (Vago et al., Reference Vago, Westhall, Pasteur Instrument Teams and Landing Site Selection Working Group2017). Information gathered by ongoing and planned Martian missions will also direct lab studies in this area for the coming decade.
Collection of data in such an accessible format will make it more available for others. We propose that those with relevant work should add their contributions to databases and similar data sets in order to make their work accessible. We believe this format is not just helpful for the origins of life community but would also be useful for other scientific fields that are highly collaborative and multidisciplinary. This format is able to not only inform laboratory studies but can impact future mission studies. In the context of confirming methane on these planetary bodies, different techniques in the lab have been explored. The techniques used in these laboratory studies can also inform new technology for future missions and provide different options for detection and quantification. We hope that our work can organize different techniques used and identify gaps in flight technology for upcoming missions.
Conclusion
CO and CO2 reduction are important processes that are a focus of much active research in astrobiology. We have tabulated experimental work relevant to CO and CO2 reduction under geological settings relevant to Mars and early Earth. This table is useful both for those interested in the background of this experimental and theoretical research area as well as those looking to test different theoretical conditions experimentally. We posit that collecting data in such a manner will be beneficial for astrobiology and help connect researchers interested in these worlds. We also suggest that other researchers add their research to similar databases and tables.
Supplementary material
The supplementary material for this article can be found at https://doi.org/10.1017/S1473550422000052.
Acknowledgements
We thank Dr Yuk Yung and Danica Adams (Caltech) for helpful discussions. This research was carried out at the Jet Propulsion Laboratory, California Institute of Technology, under a contract with NASA (80NM0018D004). JMW, LMB and MP were funded by NASA/NSF Ideas Lab for the Origins of Life, ‘Becoming Biotic: Recapitulating Ancient Cofactor-Mediated Metabolic Pathways on the Early Earth’. LMB was also was supported by a JPL Researchers on Campus award, ‘Investigating mechanisms of carbon reduction and abiotic methane generation on Mars’. JMW and LER were supported by JPL Strategic Research and Technology Development (R&TD), ‘Fate of Organics on Ocean Worlds.’ RYS was supported by a JPL Strategic Research and Technology Development.” (R&TD), ‘Experimental Constraints on Groundwater Driven Redox Gradients on Mars.’ Copyright 2021 California Institute of Technology. Government sponsorship acknowledged.
Conflict of interest
No authors declare a conflict of interest.