1. Introduction
The Takab complex of northwest Iran is located within the Alpine–Himalayan orogenic system. The Takab complex has been assigned to various tectonic subdivisions of Iran. It is included in the Central Iran Zone by Berberian & King (Reference Berberian and King1981), at the junction of the Central Iran, the Alborz–Azerbaijan and the Sanandaj–Sirjan zones (Babakhani & Ghalamgash, Reference Babakhani and Ghalamghash1990) and in the Sanandaj–Sirjan Zone (Gilg et al. Reference Gilg, Boni, Balassone, Allen, Banks and Moore2006) (Fig. 1a). Geological and lithological characteristics of the Takab area show affinities to the Central Iran micro-continent (Hajialioghli et al. Reference Hajialioghli, Moazzen, Droop, Oberhänsli, Bousquet, Jahangiri and Ziemann2007a, Reference Hajialioghli, Moazzen, Jahangiri, Droop, Bousquet and Oberhänslib). Zircon U/Pb dating of granitic gneisses from the Takab area yields an age of 560 Ma (Stockli et al. Reference Stockli, Hassanzadeh, Stockli, Axen, Walker and Dewane2004), which is comparable to U/Pb ages of granitic augen gneisses from the Central Iran micro-continent (c. 550 Ma: Ramezani & Tucker, Reference Ramezani and Tucker2003) and also similar rock types from the Menderes Massif of Turkey, to the west of the area (520–570 Ma, Loos & Reischmann, Reference Loos and Reischmann1999). Tertiary magmatism occurred in relation to the subduction of the Neo-Tethys (Şengör, Reference Şengör1984; Mohajjel & Fergusson, Reference Mohajjel and Fergusson2000; Mohajjel, Fergusson & Sahandi, Reference Mohajjel, Fergusson and Sahandi2003). In the context of the timing of closure of the Neo-Tethys and inception of collision between the Arabian and Iranian plates, different opinions are postulated. Berberian & King (Reference Berberian and King1981) and Alavi (Reference Alavi1994) advanced a late Cretaceous age for collision along the Zagros suture. Others describe a Cenozoic continental collision (e.g. Eocene: Berberian et al. Reference Berberian, Muir, Pankhurst and Berberian1982; J. Braud, unpub. Ph.D. thesis, Univ. de Paris-Sud, 1987; Şengör et al. Reference Şengör, Altiner, Cin, Ustaomer, Hsu, Audley-Charles and Hallam1988; Şengör, Natal'in & Burtman, Reference Şengör, Natal'in and Burtman1993; Şengör & Natal'in, Reference Şengör, Natal'in, Yin and Harrison1996; Mohajjel, Fergusson & Sahandi, Reference Mohajjel, Fergusson and Sahandi2003; Ghasemi & Talbot, Reference Ghasemi and Talbot2006; Oligocene: Agard et al. Reference Agard, Omrani, Jolivet and Mouthereau2005; and Miocene: Jackson et al. Reference Jackson, Aki, Cornell, Dieterich, Henyey, Mahdyiar, Schwartz and Ward1995; Şengör et al. Reference Şengör, Özeren, Keskin, Sakinç, Özbakir and Kayan2008). Based on palinspastic and plate tectonic studies, McQuarrie et al. (Reference McQuarrie, Stock, Verdel and Wernicke2003) suggested that the collision between the Iranian plate and the Central Iran micro-continent took place at 20 Ma at the latest, in relation to the subduction of the Neo-Tethys. Recent studies by Verdel et al. (Reference Verdel, Wernicke, Ramezani, Hassanzadeh, Renne and Spell2007) support a Neogene collision between the Iranian plate and the Central Iran micro-continent.

Figure 1. (a) Structural subdivision map of Iran from Gilg et al. (Reference Gilg, Boni, Balassone, Allen, Banks and Moore2006). The study area is shown by a rectangle. (b) Structural map of the area. (c) Geological map of the study area, adapted from geological maps of Takht-e-Solyman (Babakhani & Ghalamgash, Reference Babakhani and Ghalamghash1990) with some modifications. Dashed boxes are enlarged in Figure 2. (d, e) Cross-sections across profiles A–B and C–D to show the relations between the rock units in the area.
The focus of this study is the calc-alkaline granitoids of the Takab area. This is the first study of the petrology, geochemistry and petrogenesis of the Tertiary granitoids in the area. The combination of the data will allow us to address the tectonic setting and geochemical significance of these rocks.
The composition of coexisting minerals is used to estimate the P–T conditions of equilibration during magmatic crystallization and subsolidus cooling stages of the magmatic rocks.
This study, along with ongoing studies on the associated granulites, amphibolites, metapelites and meta-ultramafic rocks (R. Hajialioghli, unpub. Ph.D. thesis, Univ. Tabriz, 2007; Hajialioghli et al. Reference Hajialioghli, Moazzen, Droop, Oberhänsli, Bousquet, Jahangiri and Ziemann2007a; Moazzen et al. Reference Moazzen, Oberhänsli, Hajialioghli, Möller, Bousquet, Droop and Jahangiri2009), will furnish a framework to decipher the geodynamic history of the Takab and the adjacent areas. This may elucidate the geological history of NW Iran in relation to the Tethyan-related events.
2. Geological setting and field relations
The Takab complex in NW Iran is located between longitude 47°45′ and 47°05′E and latitude 37°30′ and 36°30′N. It occurs as a NW–SE-trending horst which is thrust along the reverse faults (Figs 1, 2). The eastern border is marked by the Pari basin. The Qeynarj-e-Chartagh thrust fault forms the western border with the Shirmard basin (Fig. 1b). It consists of a variety of rocks including granulites, metabasites, amphibolites, migmatites, calc-silicate rocks, meta-ultramafics, gneisses, mica-schists and sporadically, granitoids (Hajialioghli et al. Reference Hajialioghli, Moazzen, Droop, Oberhänsli, Bousquet, Jahangiri and Ziemann2007a, Reference Hajialioghli, Moazzen, Jahangiri, Droop, Bousquet and Oberhänslib; Moazzen et al. Reference Moazzen, Oberhänsli, Hajialioghli, Möller, Bousquet, Droop and Jahangiri2009; Saki, Reference Saki2010). The oldest crustal protolith of the Takab basement has been dated about 2961±72 Ma (206Pb/238U–207Pb/235U) and 2775–2875 Ma (204Pb corrected 207Pb/206Pb) (R. Hajialioghli, unpub. Ph.D. thesis, Univ, Tabriz, 2007; Moazzen & Hajialioghli, Reference Moazzen and Hajialioghli2008), comparatively similar to the ages from the Central Iran micro-continent (c. 2140 Ma, 207Pb/206Pb: Ramezani & Tucker, Reference Ramezani and Tucker2003 and c. 2382 Ma, Rb/Sr whole rock ages: Haghipour, Reference Haghipour1974) and Bozburun of southeastern Turkey, to the west of the study area (c. 2522 Ma, 207Pb/206Pb: Kröner & Şengör, Reference Kröner and Şengör1990).

Figure 2. Larger map of the area showing rock units and the sample localities: (a) north of the area and (b) south of the area.
The Takab complex experienced regional metamorphism in Precambrian times, overprinted by another high-grade metamorphism during Tertiary times, producing the migmatites and the granitoids (very similar to Central Iran: Ramezani & Tucker, Reference Ramezani and Tucker2003). The age for Precambrian metamorphism is documented by dating on gneisses of the area by Stockli et al. (Reference Stockli, Hassanzadeh, Stockli, Axen, Walker and Dewane2004), and the age for migmatization and resulted granitoids is indicated by zircon SHRIMP dating (R. Hajialioghli, unpub. Ph.D. thesis, Univ. Tabriz, 2007; Moazzen & Hajialioghli, Reference Moazzen and Hajialioghli2008).
Migmatization of the crustal rocks in the Takab area occurred at c. 25 Ma (U/Pb dating on zircons from the leucosome parts of the migmatites: R. Hajialioghli, unpub. Ph.D. thesis, Univ. Tabriz, 2007; Moazzen & Hajialioghli, Reference Moazzen and Hajialioghli2008), corresponding to the Alpine orogeny. The granitoids studied here are small and scattered exposures within the metamorphic rocks. They appear as voluminous leucosome parts of migmatites in places and also as isolated granitic bodies within the metamorphic rocks (Fig. 3), indicating possible short-distance migration of the leucosome parts, coalescence of the melt and formation of the granitoid patches. The sizes of these granitic patches are too small to be shown on the map. Only the sampling locations are shown. The old metamorphic rocks and the granitoids are unconformably overlain by the Oligo-Miocene volcanic and sedimentary rocks (Fig. 1c–e). The Oligo-Miocene sedimentary rocks include basal conglomerate, marl and red sandstone. The conglomerate is polygenetic, having various fragments of metamorphic rocks (Babakhani & Ghalamgash, Reference Babakhani and Ghalamghash1990). Alluviums and enormous deposits of travertine along the thrust faults are Quaternary in age (Figs 1, 2).

Figure 3. Field photographs of the granitoids and the migmatitic rocks. (a) Limited outcrop of granitoids. The width of the photo is ~2 m. (b) Granitoid patches intruded the amphibolites with small amphibolite enclave. Visible portion of marker is 11 cm long. (c) Leucosome portion of migmatite cross-cutting the rocks. Length of hammer is 33 cm. (d) Mesosome and melanosome parts ‘floating’ in the granitoid leucosome. Length of pen is 16 cm.
Considering the lack of Palaeozoic and Mesozoic sediments, Babakhani & Ghalamgash (Reference Babakhani and Ghalamghash1990) believed that the Takab metamorphic basement most probably was a high region during Palaeozoic and Mesozoic times.
There is no published account on the radiometric age of the granitoids, but as discussed below, a Tertiary age can be considered for them. On the basis of field relations, Lotfi (Reference Lotfi2001) considered the relative age of the granitoids to be post-Cretaceous. Mazhari et al. (Reference Mazhari, Bea, Amini, Ghalamghash, Molina, Montero, Scarrow and Williams2009) believed these granitoids to be Tertiary, and Babakhani & Ghalamgash (Reference Babakhani and Ghalamghash1990) also considered the granitoids to be Tertiary in age. The late Palaeozoic and Mesozoic rocks are lacking in this area. In addition, Oligo-Miocene sediments and volcanic rocks unconformably cover the metamorphic basement rocks and the granitoids. Fragments of amphibolites and gneisses appear as enclaves in granodiorite in some places. There are sharp contacts between amphibolite and granodiorite in the SW Barut-Aghaji and north of the village of Baglu-kandi (Figs 1, 3b). Mineralogically the Takab granitoids are very similar to plutonic rocks from the Sanandaj–Sirjan Zone of the Zagros belt and the Central Iran Zone (e.g. Mazhari et al. Reference Mazhari, Bea, Amini, Ghalamghash, Molina, Montero, Scarrow and Williams2009), which are mainly Tertiary in age and are related to the Neo-Tethys oceanic crust subduction and the subsequent collision. U/Pb zircon dating on migmatites, which are spatially associated with granitoid intrusions, gives an age of c. 25 Ma (R. Hajialioghli, unpub. Ph.D. thesis, Univ. Tabriz, 2007). Taking all these facts together, a Tertiary age for the studied granitoids is very likely. However, appropriate age dating studies will furnish more information in this regard.
Faulting and folding of the Oligo-Miocene sediments and volcanic rocks (Aghdareh anticline in the SW of the study area, which consists of Oligo-Miocene limestone, marl and andesite and Ghar-e-Tapa anticline in the SW having folded Oligo-Miocene conglomerate and sandstone; Fig. 1), refolding of the Precambrian metamorphic complex (Lal-e-Kan anticline in south and Chughoti anticline in the NW of the area; Fig. 1) and occurrence of Miocene–Pliocene continental sediments related to the collisional regime, are results of Alpine orogeny in the area. All rock units in the area show a NW–SE trend, parallel to the main deformational features (e.g. gneissosity, schistosity and foliation). The NW–SE trend is consistent with the compression of the rocks between the Arabia and Eurasia continents, corresponding to the closure of Neo-Tethys and the subsequent continental collision.
3. Petrography
The petrography of the granitoids and the associated migmatites is provided below. Modal amounts of minerals in the granitoids and migmatitic leucosomes are presented in Table 1. Mineral abbreviations are from Kretz (Reference Kretz1983), except for amphibole (Amp).
Table 1. Mineral composition (modal %) of the granitoids and migmatitic leucosomes from the Takab area

The studied granitoids are classified as granodiorites, Qtz-monzodiorites and granites on the Streckeisen (Reference Streckeisen1974) diagram.
Granodiorites. Coarse to medium granular texture is common in the granodiorites (Fig. 4a). The dominant mineral assemblage is plagioclase (30–50 modal %), K-feldspar (7–15 modal %), hornblende (10–45 modal %) and quartz (20–25 modal %). K-feldspar occurs as small subhedral laths and anhedral grains ranging from 0.2 to 0.4 mm in diameter. Plagioclase displays polysynthetic and Karlsbad twinnings.

Figure 4. (a) Hornblende and plagioclase with granular texture in the granodiorite. Plagioclase shows compositional zoning. Crossed nicols, field of view 4.8 mm. (b) Subsolidus actinolite around magmatic hornblende in the granodiorite. Plain polarized light, field of view 2.4 mm. (c) Clinopyroxene, amphibole and plagioclase in the quartz diorite. Subsolidus amphibole is seen parallel to the cleavage system of clinopyroxene. Plain polarized light, field of view 2.4 mm. (d) Magmatic titanite in the diorite. Amphibole as patches within clinopyroxene and as products of deuteric alteration. Plain polarized light, field of view 2.4 mm. (e) The migmatitic leucosome with granular texture. Interstitial quartz and K-feldspar are anhedral. Plain polarized light, field of view 2.4 mm. (f) Hornblende and plagioclase with foliated texture in the mesosome. Plain polarized light, field of view 2.4 mm.
Coarse-grained plagioclase exhibits optical zoning (Fig. 4a). Amphibole with greenish-brown to grey and olive-green pleochroism displays well-developed cleavages and is frequently twinned. In some hornblende crystals up to 2 cm in diameter, many zircons and opaque minerals occur as inclusions. Epidote, titanite and biotite are present in minor amounts. Magmatic epidote, in textural equilibrium with other magmatic phases, occurs as zoned and twinned crystals. Secondary epidote, replacing plagioclase, is seen sporadically in the rocks. Zircon, apatite and opaque minerals are accessory phases. Actinolite around hornblende is a late subsolidus crystallization product (Fig. 4b). Coarse-grained plagioclase and hornblende porphyroclasts in a fine-grained matrix are characteristics of the brecciated granodiorites (SW of Barut-Aghaji; Fig. 2). Plagioclase and quartz crystals show undulatory extinction.
Qtz-diorite. The mineral assemblage of the rock is plagioclase (35–45 modal %), hornblende (20–35 modal %), clinopyroxene (15 > modal %) and quartz (5–10 modal %). Subhedral plagioclase (up to 4 mm in diameter) is weakly zoned and shows polysynthetic twinning. It is full of clinopyroxene inclusions and has a poikilitic texture. Clinopyroxene with a granular texture reaches up to 0.7 mm in diameter and is simply twinned. Amphibole is crystallized at the margins and parallel to the cleavage system of clinopyroxene (Fig. 4c). The magmatic hornblende (0.6 mm in length) is optically zoned and shows strong pleochroism. Euhedral titanite (up to 1.2 mm in diameter) and epidote are minor phases (Fig. 4d).
Qtz-monzodiorites. Plagioclase (45–50 modal %), hornblende (10–40 modal %) and K-feldspar (<10 modal %) with a granular texture are the main constituent minerals of these rocks. Hornblende, 0.8 mm in diameter, is simply twinned and plagioclase (up to 5 mm in diameter) shows weak optical zoning. Titanite (0.4 mm in diameter) occurs in minor amounts. Apatite, zircon and opaque phases are accessory minerals.
Granites. Dominant minerals are quartz (20–30 modal %), plagioclase (25–40 modal %), K-feldspar (10–20 modal %) and hornblende (5–15 modal %). Granular texture is dominant in the granites. Hornblende reaches 1 mm in diameter. Plagioclase contains hornblende as inclusions. Titanite and biotite are minor phases. Accessory phases are zircon and apatite.
Mafic migmatites. Mafic migmatites occur in association with granitoids in the NW Ghar-e-Naz (Fig. 2; R. Hajialioghli, unpub. Ph.D. thesis, Univ. Tabriz, 2007). Structurally the investigated migmatites are very heterogeneous. Diatexite is the most common structure of the Takab mafic migmatites. Foliation in the mafic migmatites is marked by separation of the alternating centimetre-scale light-coloured quartz-feldspathic (leucosome) and dark hornblende-rich (melanosome) layers. Melanosome is also formed as thin selvages around leucosome. Schlieren structure includes floating melonocratic fragments in leucosome and is seen in some of the rocks (R. Hajialioghli, unpub. Ph.D. thesis, Univ. Tabriz, 2007). Migmatitic leucosomes have compositions consistent with granodiorite, Qtz-monzodiorite and Qtz-diorite. Medium- to fine-grained granular texture is common in the leucosomes. Subhedral plagioclase, 0.7 mm in diameter, is twinned (Fig. 4e). Quartz and K-feldspar occur with interstitial texture filling the spaces between plagioclase laths (Fig. 4e). Hornblende, biotite and titanite are present in minor amounts. Hornblende (>50 modal %) and minor plagioclase are the essential minerals in the melanosome. The mesosome is characterized by foliated texture. It is composed of hornblende and plagioclase together with minor K-feldspar and quartz (Fig. 4f). Table 1 includes modal amounts of minerals of the mafic migmatites in the Takab area.
4. Analytical methods
Well-defined samples from the granitoids were analysed for major and trace elements at the GeoForschungsZentrum, Potsdam, Germany. In addition, the leucosome, mesosome and melanosome portions of the migmatites were carefully separated and analysed for major oxides and trace elements. International rock standards were measured in the same runs to monitor the precision and accuracy of the XRF technique. The precision of the analyses is 1–3% for the major element oxides and <5% for the trace elements. The results are presented in Table 2. FeO/Fe2O3 ratio is calculated using the conventional equation of Irvine & Baragar (Reference Irvine and Baragar1971) and SiO2 v. (Na2O+K2O) diagram (Le Maitre, Reference Le Maitre1976). The amount of Fe2O3 is very low. Total iron is shown as Fe2O3 in Table 2.
Table 2. Major and trace element compositions of the representative rocks in the Takab area

Gran. – Granitoid; Leuc. – Leucosome; Meso. – Mesosome. *Fe total as Fe2O3.
Minerals from the granitoids were also analysed for major element oxides by wavelength-dispersive spectrometry using a CAMECA SX-100 microprobe at the GeoForschungsZentrum, Potsdam, Germany. The measuring conditions were 15 kv accelerating voltage, 10–20 nA beam current. The spot size was between 3 and 5 μm. A PAP correction procedure was applied. Natural and synthetic standards ((Fe2O3 (Fe)), rhodonite (Mn), rutile (Ti), MgO (Mg), wollastonite (Si, Ca), fluorite (F), orthoclase (Al, K) and albite (Na)) were used for the calibration of the element oxides. Representative mineral analyses are given in Table 3.
Table 3. Representative mineral analyses from the Takab granitoids

Amp – amphibole; Pl – plagioclase; Cpx – clinopyroxene; Ttn – titanite; Ilm – ilmenite; Ep – epidote.
5. Whole-rock geochemistry
5.a. Major elements
Major element concentrations of the analysed rocks are listed in Table 2. The granitoids span a wide range of SiO2 content (54–74 wt%). MgO, CaO, TiO2 and FeOt decrease with increasing SiO2 content. Na2O concentration of the granitoids is high at intermediate SiO2 contents but it decreases toward more felsic compositions. CaO, Na2O, TiO2, MgO and Al2O3 variations versus SiO2 in the leucosome part of migmatites show similar trends to those defined by these oxides in the granitoids.
Classification based on the normative Ab–Or–An scheme (Barker, Reference Barker and Barker1979) identifies the analysed rocks as quartz monzonite, granodiorite and tonalite. On the total alkalis versus silica (TAS) diagram (Middlemost, Reference Middlemost1994; Fig. 5a), the investigated granitoids are classified as granite, granodiorite, quartz monzonite, monzonite and quartz diorite. The rocks dominantly plot in the sub-alkaline field of the diagram of Irvine & Baragar (Reference Irvine and Baragar1971) (Fig. 5a). The agpaitic index (AI=mol (Na+K/Al)) shows that the samples are predominantly calc-alkaline (AI<0.87; Liégeois & Black, Reference Liégeois, Black, Fitton and Upton1987). Based on the discriminant diagram of Frost et al. (Reference Frost, Barnes, Collins, Arculus, Ellis and Frost2001), the granitoids indicate alkali-calcic, calc-alkalic and calcic rock series (Fig. 5b). The analysed rocks have normative diopside, hypersthene and titanite, but normative corundum never exceeds 1%. These features most likely characterize an I-type nature (Nagudi, Kobert & Kurat, Reference Nagudi, Kobert and Kurat2003) for the source materials of the investigated granitoids.

Figure 5. Geochemical classification of the granitoids. (a) Total alkalis v. silica diagram (Middlemost, Reference Middlemost1994). (b) Major element discrimination diagrams of Frost et al. (Reference Frost, Barnes, Collins, Arculus, Ellis and Frost2001). The analysed rocks range from alkali-calcic, calc-alkali to calcic rock series. (c) A/CNK v. SiO2 (wt%) diagram (Chappell & White, Reference Chappell and White1974). (d) Plot of A/NK v. A/CNK for the granitoids and migmatitic leucosome in the Takab area. A/NK = molar ratio of Al2O3/(Na2O+K2O); A/CNK = molar ratio of Al2O3/(CaO+Na2O+K2O). The analysed rocks indicate dominantly metaluminous characteristics. The leucosome compositions are presented for comparison.
The aluminium saturation index (ASI: molecular ratio of Al2O3/(Na2O+K2O+CaO)), ranging from 0.46 to 1.04, shows that the granitoids are metaluminous, comparable with migmatitic leucosome compositions (Fig. 5c). The diagram of Al2O3/(Na2O+K2O) (A/NK) against Al2O3/(CaO+Na2O+K2O) (A/CNK) also indicates a metaluminous characteristic for these rocks (Fig. 5d).
5.b. Trace elements
Trace element concentrations of the Takab granitoids and the associated migmatites are listed in Table 2, and their variations versus SiO2 are plotted on Harker diagrams (Fig. 6). Sr concentration decreases, whereas Ba, Nb and Rb contents increase with increasing SiO2 content. Zirconium shows a considerably scattered pattern (Fig. 6). Ni, Cr and V (transitional elements) exhibit a negative correlation with silica content since they behave as compatible elements. Samples from migmatitic leucosomes have compositional trends comparable with the trends for the granitoids.
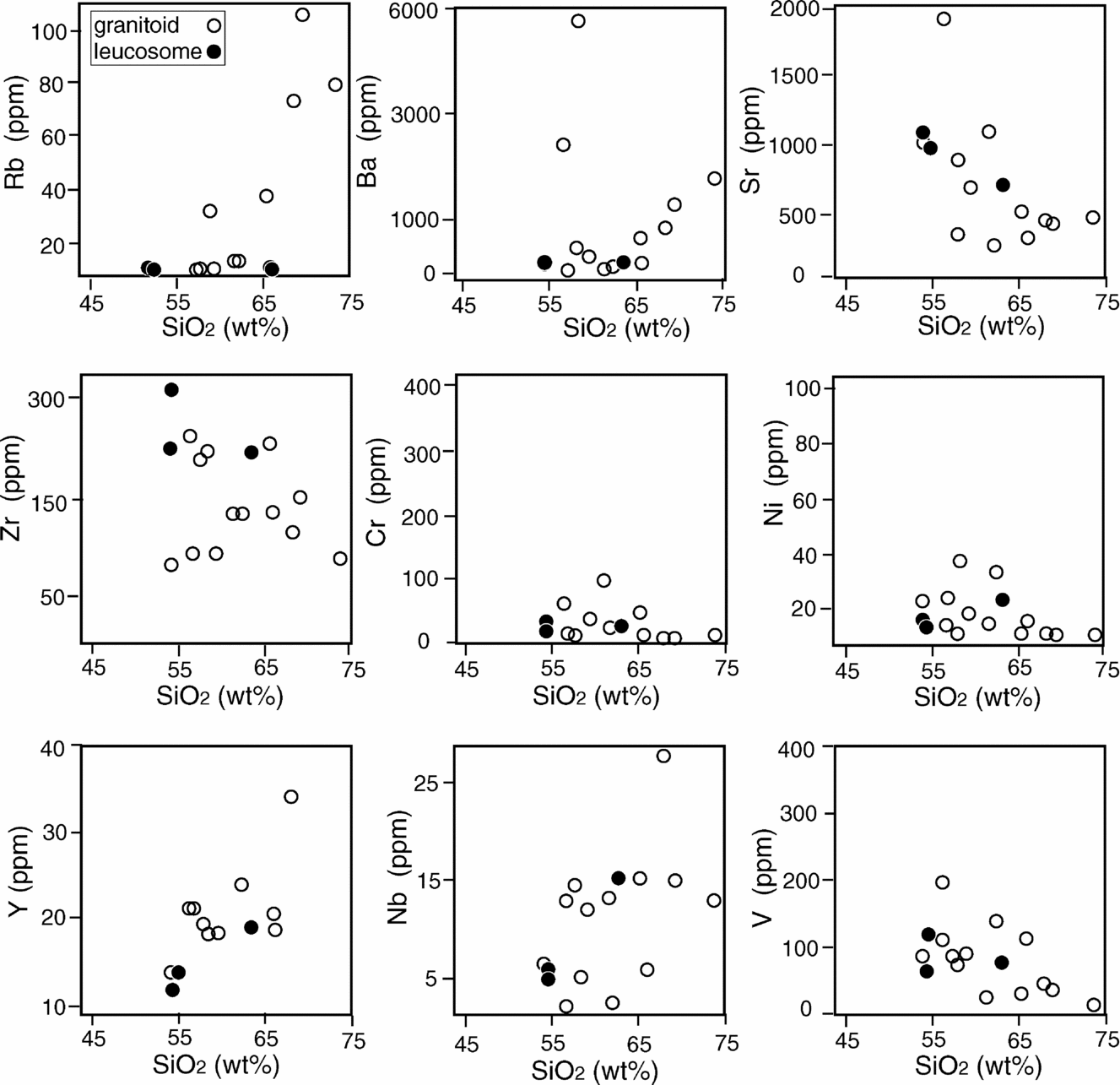
Figure 6. Trace element variations for the granitoids from the Takab area. For details see text.
The Rb/Zr–SiO2 diagram (Harris, Pearce & Tindle, Reference Harris, Pearce, Tindle, Coward and Ries1986) is used to discriminate I- and A-type granitoids from S-type rocks. The Takab granitoids and the migmatitic leucosomes plot mainly in the I- and A-type fields (Fig. 7a). The diagram of Zr versus SiO2 (Collins et al. Reference Collins, Beams, White and Chappell1982) verifies the distinct I-type nature of these rocks (Fig. 7b).

Figure 7. (a) Rb/Zr v. SiO2 diagram (Harris, Pearce & Tindle, Reference Harris, Pearce, Tindle, Coward and Ries1986) discriminating I-and A-types granitoids from S-type rocks. (b) Zr v. SiO2 diagram of Collins et al. (Reference Collins, Beams, White and Chappell1982). Both verify I-type nature for the analysed rocks.
The trace elements of the granitoids are normalized to primitive-mantle values (Sun & McDonough, Reference Sun, McDonough, Saunders and Norry1989; Fig. 8). The composition of the leucosomes from the Takab area is also represented for comparison (Fig. 8). The trace element patterns of the different rock types show almost similar trends (Fig. 8). Nb and Ti show negative anomalies, but the Zr anomaly is positive.

Figure 8. Primitive mantle-normalized element patterns for the granitoids from the Takab area. Normalization factors are after Sun & McDonough (Reference Sun, McDonough, Saunders and Norry1989). The migmatitic leucosome compositions are presented for comparison.
6. Mineral chemistry
6.a. Feldspar
Representative electron microprobe analyses and the structural formula of plagioclase are given in Table 3. The plagioclase is zoned with core compositions of XAn~0.48 and XAn~0.35 and rim compositions of XAn~0.38 and XAn~0.21 in the quartz diorite and the granodiorite, respectively. The decrease of Ca from the core to the rim is interpreted as normal zoning during crystallization. The K2O content of the plagioclase ranges between 0.03 and 0.05 (a.p.f.u.). The chemical compositions of plagioclase from granodiorite (Ab63–79An20–35Or1–4) and quartz diorite (Ab51–62An38–48Or1.0–1.5) are oligoclase and andesine, respectively.
6.b. Amphibole
Amphibole is the predominant mafic phase in the granitoids. Ferrous and ferric iron in the amphibole formula were calculated using the charge balance method. The amphiboles are calcic using the classification of Leake et al. (Reference Leake, Woolley, Arps, Birch, Gilbert, Grice, Hawthorne, Kato, Kisch, Krivovichev, Linthout, Laird, Mandarino, Maresh, Nickel, Rock, Schumacher, Smith, Stephenson, Ungaretti, Whittaker and Youzhi1997) ((Ca+Na)(M4)≥1.34; Na(M1)<0.67). Core compositions are different, having higher TiO2 content. Hornblende inclusions in plagioclase have chemical compositions indistinguishable from the core compositions of the hornblende within the rock. The Ti content of amphiboles from the granodiorite is low compared to that of the quartz diorite. On the classification diagram of Leake et al. (Reference Leake, Woolley, Arps, Birch, Gilbert, Grice, Hawthorne, Kato, Kisch, Krivovichev, Linthout, Laird, Mandarino, Maresh, Nickel, Rock, Schumacher, Smith, Stephenson, Ungaretti, Whittaker and Youzhi1997), the amphibole compositions are consistent with tschermakite and hornblende with minor amounts of edenite and tremolite end-members. Secondary tremolite has high Mg and Si and low Al contents.
6.c. Clinopyroxene
Representative clinopyroxene analyses from the quartz diorite are shown in Table 3. The clinopyroxene has mainly high MgO (14.50–15.60 wt%), FeO (6.5–13.5 wt%) and CaO (12.60–24.60 wt%) concentrations but it is low in TiO2 (<0.26wt%) and Na2O (<0.75 wt%) contents. In the classification diagram of Morimoto et al. (Reference Morimoto, Fabries, Ferguson, Ginzburg, Ross, Seifert, Zussman, Aoki and Gottardi1988), the analysed clinopyroxenes plot in the ‘Quad’ field. Their compositions vary in the range of En42–44Fs7–9Wo48–50. Clinopyroxene compositions on En–Fs–Wo diagram (after Deer, Howie & Zussman, Reference Deer, Howie and Zussman1978) are diopside. On the basis of the Ti content, clinopyroxenes from the Takab area plot in the low- and very low-Ti field of the diagram by Beccaluva et al. (Reference Beccaluva, Macciotta, Piccardo and Zeda1989). Plotting on diagrams of Ti (a.p.f.u.) against Ca+Na (a.p.f.u.) and Al2O3 against silica content (Fig. 9) shows a sub-alkaline character for the quartz diorite.

Figure 9. (a) Ti (a.p.f.u.) against Na+Ca (a.p.f.u.) diagram (Leterrier et al. Reference Leterrier, Maury, Thonon, Girard and Marchal1982). (b) Sub-alkaline characteristic of the granitoids on the SiO2 (wt%)–Al2O3 (wt%) diagram. The positions of boundaries between sub-alkaline, alkaline and peralkaline magma types are from Le Bas (Reference Le Bas1962).
6.d. Titanite
Analysed titanite from the quartz diorites has Ti and Ca contents of 0.94 (a.p.f.u.) and 0.99 (a.p.f.u.), respectively. Al concentration is also low (0.06 a.p.f.u.). ZnO and Cr2O3 contents are very low (0.07 and 0.05 to 0.10 wt%, respectively).
6.e. Epidote
The structural formula is calculated for 8 cations and 12.5 oxygens (Table 3). All iron is considered as Fe3+. Al occupies the tetrahedral site only in small amounts (up to 0.06 a.p.f.u.). AlVI/(AlVI+Fe3+) is 0.69. The mean composition of the end-members in the analysed epidote from the granodiorite is Cz11Ep79Fe–Ep10.
6.f. Ilmenite
The structural formula is calculated on the basis of 2 cations and 3 oxygens (Table 3). All iron is considered as Fe2+. Ilmenite (FeTiO3) and pyrophanite (MnTiO3) are the dominant end-members, whereas the end-member geikielite (MgTiO3) is absent. The mean composition of the analysed ilmenite from the quartz diorite is Ilm63Prh36.
7. Magmatic and subsolidus pressures and temperatures estimates
The composition of clinopyroxene was used to determine the crystallization pressure in quartz diorite, based on the McCarthy & Patiño Douce (Reference McCarthy and Patiño Douce1998) barometer which is calibrated for P–T ranges of ≥4 kbar and ≥700°C. The crystallization pressure obtained for the quartz diorite is 7.8 (±2.5) kbar, which is consistent with a depth of about 24 km, reflecting crystallization at a crustal level. The activity of the Ca-tschermak component in clinopyroxene was calculated with the ideal activity model. Table 4 shows mole fraction and ideal activity expressions used for pressure estimations by the clinopyroxene barometer of McCarthy & Patiño Douce (Reference McCarthy and Patiño Douce1998).
Table 4. Mole fraction and ideal activity expressions used for clinopyroxene geobarometry in the quartz diorite

T – temperature in K.
The significant exchange vector for amphiboles from the Takab granodiorites is Al2IV□AMgVISiIV(Na, K)−1A(Fe3+, Al)−1VI indicating a combination of both the pressure- and temperature-sensitive substitutions. Applying the Al in amphibole barometers of Hollister et al. (Reference Hollister, Grissom, Peters, Stowell and Sisson1987) and Johnson & Rutherford (Reference Johnson and Rutherford1989) (considering the proper amphibole formula calculation for 13eCNK cations (13 cations excluding Ca, Na, and K) and 23 oxygens) yields crystallization pressure of P~5 (±1) kbar (Hollister et al. Reference Hollister, Grissom, Peters, Stowell and Sisson1987) and P~4.3 (±1) kbar (Johnson & Rutherford, Reference Johnson and Rutherford1989) for the granodiorites. The aluminium in the amphibole geobarometer of Hammarstrom & Zen (Reference Hammarstrom and Zen1986) gives a pressure of 4.8 (±3) kbar. The estimated crystallization pressure for the granodiorites is consistent with the emplacement at a depth of about 13–15 km. Within the uncertainty limits of the estimated pressures, the existence of primary magmatic epidote in the paragenesis of the Takab granodiorites and quartz diorites supports the estimated pressures, since epidote in magmatic rocks crystallizes at 6–8 kbar (e.g. Naney, Reference Naney1983; Zen & Hammarstrom, Reference Zen and Hammarstrom1984, Reference Zen and Hammarstrom1988). Subsolidus recrystallization pressure of the quartz diorite is determined using the Al barometer for the composition of the secondary amphibole, which grows around clinopyroxene. It is about 5 kbar (Table 5). Al barometry for the amphibole rim composition of the granodiorite yields a subsolidus pressure of about 3 kbar (Table 5).
Table 5. Estimated temperatures and pressures for the Takab granitoids

B&H – Blundy & Holland, Reference Blundy and Holland1990; O – Otten, Reference Otten1984; H&Z – Hammarstrom & Zen, Reference Hammarstrom and Zen1986; H – Hollister et al. Reference Hollister, Grissom, Peters, Stowell and Sisson1987; M&P – McCarthy & Patiño Douce, Reference McCarthy and Patiño Douce1998; J&R – Johnson & Rutherford, Reference Johnson and Rutherford1989.
Crystallization temperatures of the investigated rocks were calculated for the core composition of the amphiboles, using the plagioclase–amphibole calibration of Blundy & Holland (Reference Blundy and Holland1990) as a reliable method for geothermometry of granitoids (Moazzen & Droop, Reference Moazzen and Droop2005), given that the reported uncertainty for Blundy & Holland's (Reference Blundy and Holland1990) calibration is ±75°C (T~850°C ±75°C for the quartz diorites and T~790°C ±75°C for the granodiorites). Estimated temperatures using the Ti content of amphibole, calibrated by Otten (Reference Otten1984), are consistent with crystallization at T~760°C and T~700°C for the quartz diorite and the granodiorite, respectively. The Ti content of the analysed amphiboles in the quartz diorite and the granodiorite decreases towards the rim. The Ti content of amphibole rims using the Otten (Reference Otten1984) method gives subsolidus temperatures of 620°C and 600°C for the quartz diorites and the granodiorites, respectively. A study by Stern, Huang & Wyllie (Reference Stern, Huang and Wyllie1975) showed that the solidus temperature for andesitic melt is about 700°C at 3 kbar under water-saturated conditions. Since the studied rocks here are more mafic than andesite, it is more likely that they had higher solidus temperatures, therefore the calculated temperatures indicate subsolidus re-equilibration conditions.
8. Discussion and conclusions
Mineralogical characteristics (Ishihara, Reference Ishihara1977; Whalen & Chappell, Reference Whalen and Chappell1988; Vyhnal, McSween & Speer, Reference Vyhnal, McSween and Speer1991) were used to verify the I-type magmatic series for the Takab granitoids. Some obvious mineralogical indications supporting the I-type character of the Takab granitoids are: (1) amphibole is the dominant constituent mafic mineral, (2) minor titanite and epidote occur in textural equilibrium with plagioclase and hornblende with a granular texture, (3) magnetite and ilmenite are the accessory opaque phases, (4) Al-rich mineral phases such as garnet and muscovite are absent in the investigated rocks, (5) the modal percentage of plagioclase is greater than K-feldspar and quartz.
The granitoids show a low aluminium saturation index (ASI), low concentration of SiO2 and high FeO, MgO and CaO contents, which are typical features for I-type granitoids. The K2O content varies from low-K to high-K series (Rickwood, Reference Rickwood1989). The low K2O content in some of the analysed granitoids can be explained by the K2O-poor nature of the source. Low Nb, Y, K2O values and FeOt/(FeOt+MgO) <0.75 for the granitoids in the Takab area are analogous values to those of calc-alkaline rocks (Guimarães et al. Reference Guimarães, Da Silva Filho, Almeida, Melo, Araújo and Sales1998; Guimarães & Da Silva Filho, Reference Guimarães and Da Silva Filho2000).
Furthermore, the high-Si and low-Ti contents of the analysed clinopyroxenes characterize the sub-alkaline nature of the magmatic rocks (e.g. Nisbet & Pearce, Reference Nisbet and Pearce1977; Moazzen & Oberhansli, Reference Moazzen and Oberhänsli2008). This feature may have two interrelated explanations: (1) high aSiO2 in the sub-alkaline magma causes a high proportion of Si and hence a low proportion of Al in the tetrahedral site of clinopyroxene, the charges being balanced by a low proportion of Ti in the octahedral site (Nisbet & Pearce, Reference Nisbet and Pearce1977); (2) low contents of Ti may reflect the crystallization history of the melt (probably the earlier crystallization of pyroxene in relation to plagioclase or early crystallization of magnetite and/or slow rates of cooling: e.g. Nisbet & Pearce, Reference Nisbet and Pearce1977). Ti and Al contents of clinopyroxene are related to the silica activity of the melt from which they crystallized (Kushiro, Reference Kushiro1960; Le Bas, Reference Le Bas1962). Therefore, low Ti contents in the analysed clinopyroxenes may characterize silica-saturated sub-alkaline magmas (Verhoogen, Reference Verhoogen1962).
The geochemical variations of the Takab granitoids indicate that they were probably generated by fractionation of basic to intermediate calc-alkaline compositions (e.g. Hall, Reference Hall1987; Opiyo-Akech, Tarney & Hoshino, Reference Opiyo-Akech, Tarney and Hoshino1999). The overall decrease in FeOt and MgO contents of the Takab granitoids while SiO2 increases can be related to the fractionation of the mafic minerals, mainly hornblende. The Mg no. (Mg/(Mg+Fe)) of the granitoids, ranging from 25 to 65, may support this. Compatible elements such as Cr and Co are strongly depleted in the silicic rocks, indicating lower abundance of mafic minerals (Fig. 6). The decrease of TiO2 content in the silicic compositions is more likely due to crystallization of accessory minerals such as titanite/rutile from the melt. The decrease of V content with increasing SiO2 (Fig. 6) suggests fractionation of Fe–Ti oxides. Negative correlation between Ca and Sr versus SiO2 indicates crystallization of plagioclase during differentiation. The steady increase in Rb and K2O concentrations precludes alkali feldspar fractionation in the intermediate compositions. The P2O5 versus SiO2 array displays an abrupt change in concentration of P2O5 in the intermediate compositions, which may be explained by the crystallization of apatite. The variation of Zr content, with respect to SiO2 variation, is not systematic (Fig. 6).
The major and trace element behaviour for the migmatitic leucosomes is similar to the trends defined by the granitoids (Fig. 6).
Primitive mantle normalized trace element patterns for the Takab granitoids are shown in Figure 8. The leucosome composition is also plotted to compare the results. Ba anomalies and relatively high K in these sets of rocks (Fig. 8) can be attributed to release of these elements from hornblende and/or rare K-feldspar in the source materials during partial melting (Rollinson, Reference Rollinson1993). The Sr anomaly probably indicates contribution of plagioclase as a dominant phase in the magma evolution. Negative anomalies of P and Ti indicate involvement of apatite and rutile as residual phases in the magma source. Negative Nb and Ti anomalies in the rocks can be considered as characteristic of the crustal source and/or subduction-related magmatic effects, that is, fluids (or melts) derived from subducted sediments (Saunders, Tarney & Weaver, Reference Saunders, Tarney and Weaver1980).
8.a. Tectonic setting
In order to find the tectonic setting for the granitoid magmatism in the Takab area, the compositions of the granitoids and the leucosomes of migmatites are plotted on appropriate diagrams. Both the granitoids and leucosome parts of the migmatites show affinities with volcanic arc granitoids (VAG) and syn-collisional granitoids (syn-COLG) (Fig. 10a, b). The same result is reached on the basis of major elements (FeO, MgO and CaO wt%; Maniar & Piccoli, Reference Maniar and Piccoli1989), indicating geochemical affinities similar to volcanic arc and collisional granitoids (Fig. 10c). Most of the granitoids and migmatitic leucosomes plot in the collision field of the Zr versus (Nb/Zr)N diagram of Thiéblemont & Tégyey (Reference Thiéblemont and Tégyey1994) (Fig. 10d). The collisional setting for the studied granitoids cannot be proved unequivocally and the volcanic arc origin cannot be ruled out easily, but considering what is shown in Figure 10d, a collisional setting for them is more likely. In addition, the geochemical volcanic arc features can be attributed to the nature of the materials inherited from previous arc-related protoliths.

Figure 10. (a, b) Discrimination diagrams of Y v. Nb and SiO v. Y (wt%) (after Pearce, Harris & Tindle, Reference Pearce, Harris and Tindle1984) classify the Takab granitoids as volcanic arc and syn-collisional rocks. VAG – volcanic arc granitoids; COLG – collisional granitoids; syn-COLG – syn-collisional granitoids; ORG – ocean ridge granitoids; WPG – within-plate granitoids. (c) Major discrimination diagram of Maniar & Piccoli (Reference Maniar and Piccoli1989), indicating geochemical affinities similar to volcanic arc and collisional granitoids. (d) On the Zr v. (Nb/Zr)N diagram (Thiéblemont & Tégyey, Reference Thiéblemont and Tégyey1994) the granitoids from the study area mostly plot in the continental collision field. Volcanic arc features in some samples are most likely related to the features inherited from previous arc-related protoliths. The migmatitic leucosomes are presented for comparison. A – volcanic and plutonic rocks from subduction zones (island arcs or continental margins) setting; B – rocks emplaced in continent–continent collision zone; C – lavas and plutons from within-plate continental alkaline to transitional provinces and oceanic islands; D – peraluminous rocks of continent–continent collision zones. Nb/Zr ratio normalized to the primordial mantle value (Hoffman, Reference Hoffman1988). IAG – island arc granitoids; CAG – continental arc granitoids; CCG – continental collision granitoids; POG – post-orogenic granitoids; RRG – rift-related granitoids; CEUG – continental epeirogenic uplift granitoids; OP – oceanic plagiogranites.
8.b. Crustal source material
Some field geological features such as the limited volume of the granitoids and the lack of mantle xenoliths, as well as the existence of metabasic enclaves in the magmatic rocks and existence of the mafic migmatites, lead to the proposition that the investigated granitoids generated from partial melting of a crustal source and are not mantle derived.
Chemically, the crustal source for the investigated granitoids is deduced from their Nb/Y versus Rb/Y data (Fig. 11a). The granitoids in the study area have predominantly low Rb/Nb values (0.3–3.0) and plot close to the lower crust values (Rudnick & Fountain, Reference Rudnick and Fountain1995) (Fig. 11a). High concentrations of CaO, MgO and FeOt and low K2O/Na2O values classify the source of the investigated rocks as metabasites. To constrain the source material, they were plotted in the partial melts from the metabasaltic source field diagram of Altherr & Siebel (Reference Altherr and Siebel2002) (Fig. 11b–d). The ternary plot of CaO–ASI/30–2K2O (molar) (Christofides et al. Reference Christofides, Perugini, Koroneos, Soldatos, Poli, Eleftheriadis, Del Moro and Neiva2007) verifies the results.

Figure 11. (a) Nb/Y v. Rb/Y diagram for the Takab granitoids. The lower and middle crustal compositions are from Rudnick & Fountain (Reference Rudnick and Fountain1995), and the upper crustal compositions are from Taylor & McLennan (Reference Taylor and McLennan1985). (b–d) According to Altherr & Siebel (Reference Altherr and Siebel2002), high concentrations of CaO and low K2O/Na2O ratio classify parental rocks as metabasalts. The fields on the diagram are chemical composition of melts from experimental studies of dehydration melting.
8.c. Tectonic model for the Takab complex
Geological and lithological characteristics of the Takab metamorphic complex show affinities to the Central Iran micro-continent (Hajialioghli et al. Reference Hajialioghli, Moazzen, Droop, Oberhänsli, Bousquet, Jahangiri and Ziemann2007a). An intrusion age of the gneiss in the Takab complex is dated at 560 Ma (U/Pb zircon: Stockli et al. Reference Stockli, Hassanzadeh, Stockli, Axen, Walker and Dewane2004), which is similar to a U/Pb date from basement rocks of the Saghand area in the Central Iran Zone (Ramezani & Tucker, Reference Ramezani and Tucker2003), related to the Pan-African orogeny. Migmatization of the metamorphic rocks in both the Takab area (U/Pb zircon dating, c. 25 Ma: R. Hajialioghli, unpub. Ph.D. thesis, Univ. Tabriz, 2007; Moazzen & Hajialioghli, Reference Moazzen and Hajialioghli2008) and the Central Iran Zone (U/Pb zircon dating, c. 40 Ma: Ramezani & Tucker, Reference Ramezani and Tucker2003) occurred in relation to the Alpine orogeny. If, as we have argued, the Takab metamorphic basement correlates with equivalent rocks in the Central Iran Zone, it seems reasonable to compare granitoids in the study area with those in the Central Iran Zone related to the subduction of Neo-Tethys oceanic crust during Alpine orogeny. Considering the field evidence as well as U/Pb isotope geochemistry ages of the mafic migmatites from the Takab area (c. 25 Ma; R. Hajialioghli, unpub. Ph.D. thesis, Univ. Tabriz, 2007), a Tertiary age for the Takab granitoids is very likely. Although the accurate timing of collision between the Arabian plate and the Iranian block and associated magmatic activity is highly controversial (see Section 1), recent studies based on structural and metamorphic data suggest that continental collision occurred during Oligocene times (Agard et al. Reference Agard, Omrani, Jolivet and Mouthereau2005). Widespread Neogene volcanic activities related to the extensional phase of the Alpine orogeny and U/Pb data from the migmatites provide evidence that the time of collision was Oligocene in the Takab area.
The heat required for partial melting of the crustal rocks may have been supplied from two possible sources. (1) The first is upwelling of mantle plume and advective heating of the crust by deep-seated magma chambers. This can occur in an extensional system with lithospheric thinning (breaking-off of a downward oceanic slab) or may be related to delaminating events in the area (e.g. Ghasemi & Talbot, Reference Ghasemi and Talbot2006). Such a model requires crystallization of an unreasonably large volume of mafic magma generated after cessation of subduction and crustal thickening, which is in disagreement with the scarcity of exposed mafic suites in the study area and so is ruled out on the basis of field and lithological evidence in the Takab area. (2) The heat required for partial melting of the crust has been provided by the continental collision causing crustal thickening (e.g. Agard et al. Reference Agard, Omrani, Jolivet and Mouthereau2005). The second possibility seems to be responsible for generation of the metaluminous granitoids in the Takab area. The ultimate nature of the crustal materials involved and melting conditions are significant factors which caused formation of the collisional sub-alkaline granitoids in the study area.
Melting experiments on basalts demonstrate that H2O-saturated melting occurs between 750°C and 800°C at 5 kbar (Helz, Reference Helz1976). Rapp (Reference Rapp1995) stated that melts formed by dehydration melting of amphibolites are increasingly metaluminous beyond the amphibole-out boundary. Studies by Jung, Hoernes & Mezger (Reference Jung, Hoernes and Mezger2002), Saleh, Dawood & Abdel-Naby (Reference Saleh, Dawood and Abdel-Naby2002) and Suda (Reference Suda2004) indicate that dehydration melting of amphibolites at temperatures of 900°C –1100°C produces 10–60% mafic magma (SiO2 ~50 wt%) to form tonalite, depending on bulk composition. Based on the experimental data, production of magma with compositions analogous to that of the Takab granitoids takes place under dehydration melting conditions due to hornblende breakdown in amphibolites at a temperature of 900°C and a pressure range of 5–10 kbar (cf. Wolf & Wyllie, Reference Wolf and Wyllie1991; Wyllie & Wolf, Reference Wyllie, Wolf, Pritchard, Alabaster, Harris and Neary1993; Rushmer, Reference Rushmer1991). Considering field evidence and analogous geochemical characteristics and P – T relations between the granitoids and the migmatitic leucosomes, it seems that the melt which originated from the partial melting of metabasites may have accumulated and the calc-alkaline granitoids of the Takab area have been formed from this melt.
Acknowledgements
We gratefully acknowledge A. Musiol from Potsdam University for her help with XRF analysis and Dr Rhede and Mrs Appelt from GeoForschungsZentrum Potsdam (GFZ) for access to microprobe analysis. We thank Dr Giles Droop from Manchester University for his encouragement, support and help during the course of this research and Dr Marlina Elburg for her help with an earlier version of the manuscript. Constructive comments by Dr Adel Saki and an anonymous reviewer improved the manuscript. Editorial handling of the paper by Dr Mark Allen is acknowledged.