1. Introduction
The Sierras Pampeanas of Argentina are large tilted fault-blocks of Precambrian to Carboniferous metamorphic and igneous basement in the present foreland of the southern Central Andes (Fig. 1). The Western Sierras Pampeanas contain evidence of terranes that were involved in the mainly middle to late Mesoproterozoic (Ectasian and Stenian) Grenvillian orogeny. Because of our still limited knowledge of those terranes we hereafter employ the term Grenville orogen(y) in its wider meaning to refer to a succession of tectonic events that occurred between c. 1.3 and 1.0 Ga and led to the amalgamation of Rodinia (e.g. Tollo et al. Reference Tollo, Corriveau, McLelland, Bartholomew, Tollo, McLelland, Corriveau and Bartholomew2004; Tollo, Reference Tollo, Selley, Cocks and Plimer2005, Johnson et al. Reference Johnson, Vervoort, Ramsey, Southworth and Mulcahy2020). As a consequence, these terranes (and Amazonia) were accreted to Laurentia and remained part of a larger supercontinent until the opening of the Iapetus Ocean in early Cambrian times and the subsequent northward drift (present coordinates) of the ancestral North American craton (Hoffman, Reference Hoffman1991; Thomas & Astini, Reference Thomas and Astini1996; Dalziel, Reference Dalziel1997 and references therein; Tohver et al. Reference Tohver, van der Pluijm, Van der Voo, Rizzotto and Scandolara2002, Reference Tohver, Bettencourt, Tosdal, Mezger, Leite and Payolla2004; Li et al. Reference Li, Bogdanova, Collins, Davidson, De Waele, Ernst, Fitzsimons, Fuck, Gladkochub, Jacobs, Karlstrom, Lu, Natapov, Pease, Pisarevsky, Thrane and Vernikovsky2008; Casquet et al. Reference Casquet, Rapela, Pankhurst, Baldo, Galindo, Fanning, Dahlquist and Saavedra2012).
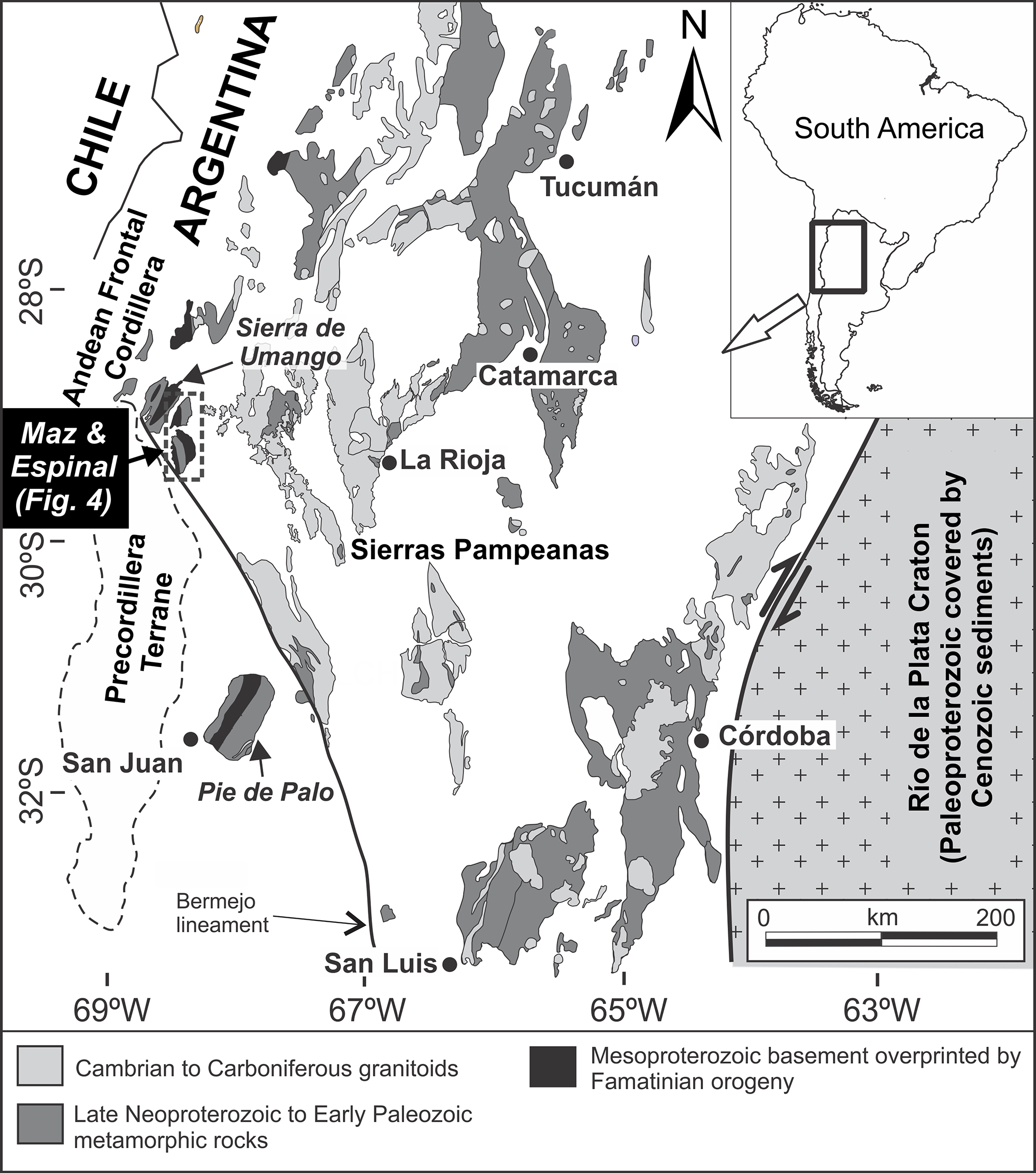
Fig. 1. Simplified geological map of the Sierras Pampeanas of Argentina.
U–Pb zircon evidence of Mesoproterozoic (1.33 to 1.0 Ga) terranes in the Western Sierras Pampeanas has been long recognized (for a review see Varela et al. Reference Varela, Basei, González, Sato, Naipauer, Neto, Cingolani and Meira2011). The first was found in the Sierra de Pie de Palo (Fig. 1) and is an oceanic terrane (McDonough et al. Reference McDonough, Ramos, Isachsen, Bowring and Vujovich1993; Ramos et al. Reference Ramos, Dallmeyer, Vujovich, Pankhurst and Rapela1998; Vujovich & Kay, Reference Vujovich, Kay, Pankhurst and Rapela1998; Casquet et al. Reference Casquet, Baldo, Pankhurst, Rapela, Galindo, Fanning and Saavedra2001; Vujovich et al. Reference Vujovich, van Staal and Davis2004). The Maz terrane in the northern area of the Western Sierras Pampeanas is continental and consists of an Andean-type magmatic arc (1.33–1.26 Ga), an Anorthosite–Mangerite–Charnockite–Granite (AMCG) complex (1.09–1.07 Ga) and older metasedimentary rocks (Casquet et al. Reference Casquet, Rapela, Pankhurst, Galindo, Dahlquist, Baldo, Saavedra, González-Casado and Fanning2005, Reference Casquet, Pankhurst, Fanning, Baldo, Galindo, Rapela, González-Casado and Dahlquist2006; Rapela et al. Reference Rapela, Pankhurst, Casquet, Baldo, Galindo, Fanning and Dahlquist2010; Martin et al. Reference Martin, Collins and Spencer2019). The latter contain no detrital zircons younger than 1.7 Ga and have Nd model ages between 2.6 and 1.7 Ga and very radiogenic common Pb, which led Casquet et al. (Reference Casquet, Pankhurst, Rapela, Galindo, Fanning, Chiaradia, Baldo, González-Casado and Dahlquist2008) to infer that the protoliths probably formed the sedimentary cover to a Palaeoproterozoic basement older than 1.7 Ga. The Maz terrane was further reworked by the Silurian Famatinian orogeny (Lucassen & Becchio, Reference Lucassen and Becchio2003; Casquet et al. Reference Casquet, Rapela, Pankhurst, Galindo, Dahlquist, Baldo, Saavedra, González-Casado and Fanning2005, Reference Casquet, Pankhurst, Rapela, Galindo, Fanning, Chiaradia, Baldo, González-Casado and Dahlquist2008; Tholt, Reference Tholt2018; Webber, Reference Webber2018).
The inferred Palaeoproterozoic basement of the Maz terrane was the basis of the MARA continental block (or craton) concept (Casquet et al. Reference Casquet, Rapela, Pankhurst, Baldo, Galindo, Fanning, Dahlquist and Saavedra2012). MARA is an acronym for Maz, Arequipa, Rio Apa. The Rio Apa and Arequipa terranes, south and southwest of Amazonia, have basements of Palaeoproterozoic age (Fig. 2; Loewy et al. Reference Loewy, Connelly and Dalziel2004; Casquet et al. Reference Casquet, Fanning, Galindo, Pankhurst, Rapela and Torres2010; Cordani et al. Reference Cordani, Teixeira, Tassinari, Coutinho and Ruiz2010; Teixeira et al. Reference Teixeira, Cordani, Faleiros, Sato, Maurer, Ruiz and Azevedo2020). Along with the alleged Maz terrane basement, they consist of rocks formed between 2.1 and 1.7 Ga, with Nd residence ages (TDM) between 2.6 and 1.7 Ga, which led to the hypothesis that they formed part of a single continental block (Casquet et al. Reference Casquet, Rapela, Pankhurst, Baldo, Galindo, Fanning, Dahlquist and Saavedra2012). The Paraguá block of Eastern Bolivia (Fig. 2) also has a Palaeoproterozoic basement older than c. 1.7 Ga (Boger et al. Reference Boger, Raetz, Giles, Etchart and Fanning2005) and was probably part of MARA as well. It is thought that the MARA block or craton was first accreted to Amazonia during the San Ignacio orogeny (1.34–1.32 Ga; Boger et al. Reference Boger, Raetz, Giles, Etchart and Fanning2005), forming a large hypothetical continental mass (Amazonia + MARA) that then collided with Laurentia along the western margin (present coordinates) in Ectasian and Stenian times, during the Grenvillian orogeny (s.l.) (Casquet et al. Reference Casquet, Pankhurst, Fanning, Baldo, Galindo, Rapela, González-Casado and Dahlquist2006; Varela et al. Reference Varela, Basei, González, Sato, Naipauer, Neto, Cingolani and Meira2011) (Fig. 3).
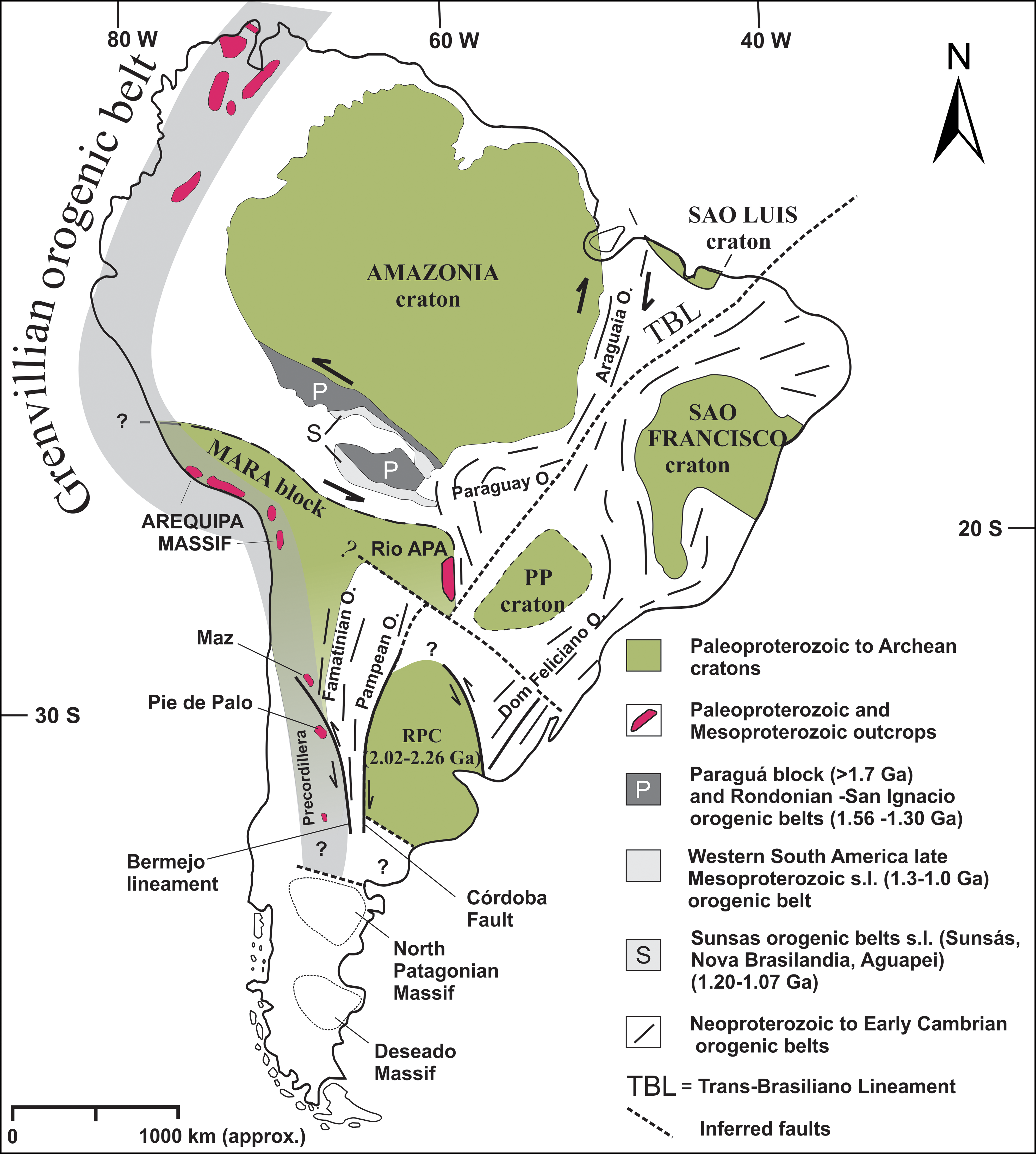
Fig. 2. Sketch map of Precambrian cratons of southern South America (modified after Casquet et al. Reference Casquet, Rapela, Pankhurst, Baldo, Galindo, Fanning, Dahlquist and Saavedra2012). MARA – acronym of Maz, Arequipa and Rio Apa; PP – Paranapanema craton; RPC – Río de la Plata craton.

Fig. 3. Palaeogeographic reconstruction of Laurentia, Amazonia and MARA in Rodinia at c. 620 Ma (modified after Ramacciotti et al. Reference Ramacciotti, Baldo and Casquet2015). Ages of Laurentian Precambrian orogenic belts and cratons according to Goodge et al. (Reference Goodge, Williams and Myrow2004) and Tohver et al. (Reference Tohver, Bettencourt, Tosdal, Mezger, Leite and Payolla2004) (Laurentia in its present position). Laurentia: TH – Trans-Hudson and related mobile belts; P – Penokean orogen; Y – Yavapay orogen; M – Mazatzal orogen; G-R – Granite–Rhyolite province.
Here we recognize, for the first time, the Maz Metasedimentary Series (MMS) within the Maz terrane, which provides important information about the pre-Grenvillian history of the MARA block. The aim of this contribution is to evaluate the probable depositional age of the MMS and the sedimentary sources in order to constrain the pre-Grenvillian history of the MARA craton and its probable link with the Columbia supercontinent. This supercontinent contained almost all the Earth’s current continental blocks, amalgamated mainly between 2.1 and 1.8 Ga (Rogers & Santosh, Reference Rogers and Santosh2002; Zhao et al. Reference Zhao, Cawood, Wilde and Sun2002) although it is possible that parts of it completed assembling even later, e.g. Australia to Laurentia at c. 1.6 Ga (Kirscher et al. Reference Kirscher, Liu, Li, Mitchell, Pisarevsky, Denyszyn and Nordsvan2019).
2. Geological setting
The Sierra de Maz is one of the westernmost of the Sierras Pampeanas (Fig. 1). We present here the first detailed geological map of the Sierra de Maz and the adjacent Sierra del Espinal based on satellite imagery and several field campaigns (Fig. 4). The first survey of the Sierra de Maz was carried out by Kilmurray & Dalla Salda (Reference Kilmurray and Dalla Salda1971) who described the lithological units and presented a very schematic map of the sierra. The Sierra del Espinal is the northern continuation of the Sierra de Maz but was left-laterally displaced along E–W-striking faults (Fig. 4): it abuts the Andean frontal belt via a steep Cenozoic thrust that is younger than the transcurrent faults. The Sierra de Maz (which reaches an altitude of 3030 m) is the more accessible of the two and shows a more complete view of the stratigraphy and structure of the Proterozoic to early Palaeozoic basement in the Andean foreland. To the east, and separated by Andean faults and late Palaeozoic-to-present sedimentary cover, is the Sierra de Ramaditas and other smaller basement outcrops near Villa Castelli (Fig. 4).
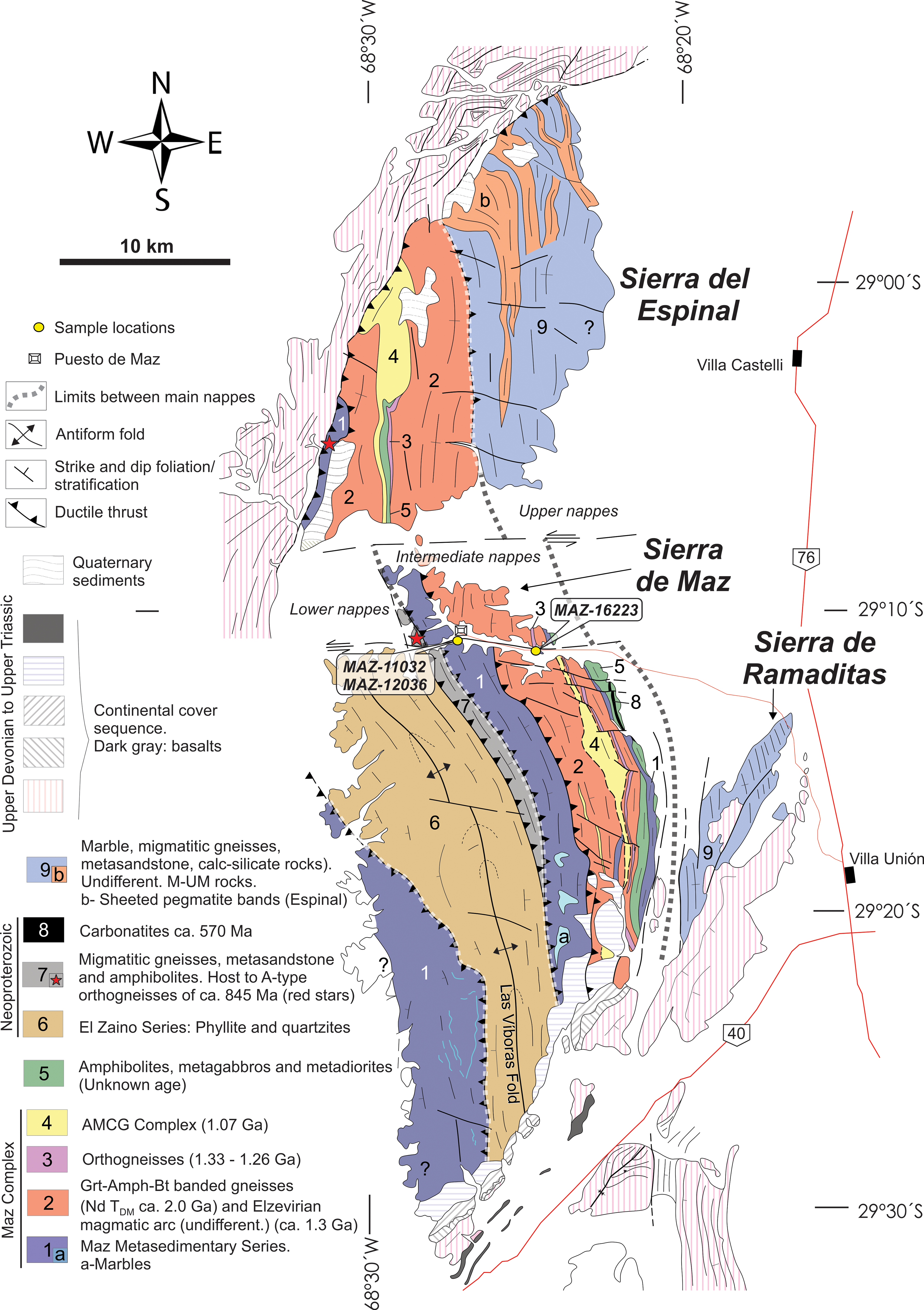
Fig. 4. Geological map of the Sierra de Maz, Sierra del Espinal and Sierra de Ramaditas. Modified after Fauqué et al. (Reference Fauqué, Limarino, Vujovich, Fernandes, Cegarra and Ecosteguy2004), Porcher et al. (Reference Porcher, Fernandes, Vujovich and Chernicoff2004) and Casquet et al. (Reference Casquet, Rapela, Pankhurst, Galindo, Dahlquist, Baldo, Saavedra, González-Casado and Fanning2005, Reference Casquet, Pankhurst, Fanning, Baldo, Galindo, Rapela, González-Casado and Dahlquist2006). AMCG – Anorthosite–Mangerite–Charnockite–Granite. El Zaino Series after Kilmurray & Dalla Salda (Reference Kilmurray and Dalla Salda1971).
The overall structure of the Sierra de Maz is an antiformally folded thrust stack (nappes) of metamorphic rocks with a discontinuous inverted metamorphic arrangement, i.e. downward decrease in metamorphic grade. The antiform (named here the Las Víboras fold) is an upright, wide, open fold striking NNW–SSE in the central part of the sierra. The eastern flank of the antiform shows a complete sequence of thrust sheets. We distinguish the structurally lowest unit in the core of the Las Víboras antiform, the intermediate group of nappes, and the upper high-grade nappes that crop out in the eastern Sierra del Espinal and in the Sierra de Ramaditas (Fig. 4). Metasedimentary rocks of Neoproterozoic to early Palaeozoic age with Nd model ages (TDM) peaking at c. 1.3 Ga occur in both the lower unit and in the upper nappes. The intermediate nappes record a complex Mesoproterozoic history of sedimentation and orogeny (Grenvillian orogeny s.l.). This complex was called the Maz Group by Kilmurray & Dalla Salda (Reference Kilmurray and Dalla Salda1971), the Maz Complex by Porcher et al. (Reference Porcher, Fernandes, Vujovich and Chernicoff2004) and the Maz suspect terrane by Casquet et al. (Reference Casquet, Pankhurst, Rapela, Galindo, Fanning, Chiaradia, Baldo, González-Casado and Dahlquist2008). Hereafter we call it the Maz Complex.
The Maz Complex consists of at least two nappes. The lower one is formed by a mainly medium-grade metasedimentary series, i.e. the MMS, with Nd model ages (TDM) of c. 2.0 Ga (Casquet et al. Reference Casquet, Pankhurst, Rapela, Galindo, Fanning, Chiaradia, Baldo, González-Casado and Dahlquist2008). The series comprises garnet, ± staurolite, ± kyanite/sillimanite schists, white quartzites, calc-silicate rocks and marbles. Amphibolites, metagabbros, metadiorites, transposed felsic dykes, rare anthophyllite–garnet gneisses and granitic orthogneisses are also found within the MMS. The upper nappe of the Maz Complex includes banded garnet–amphibole–biotite gneisses and a metamorphosed juvenile Andean-type magmatic arc of 1.33–1.26 Ga ranging from gabbro to granite, and an AMCG complex of c. 1.07 Ga (Porcher et al. Reference Porcher, Fernandes, Vujovich and Chernicoff2004; Casquet et al. Reference Casquet, Rapela, Pankhurst, Galindo, Dahlquist, Baldo, Saavedra, González-Casado and Fanning2005; Rapela et al. Reference Rapela, Pankhurst, Casquet, Baldo, Galindo, Fanning and Dahlquist2010; Martin et al. Reference Martin, Collins and Spencer2019).
Regional metamorphism is inverted ranging from high grade in the upper nappes to low grade in the core of the Las Víboras antiform. Structures in outcrop (foliation and folding), as well as the thrusts, mainly resulted from Silurian orogenic reworking (Famatinian orogeny) with peak metamorphism at c. 440 Ma (Lucassen & Becchio, Reference Lucassen and Becchio2003; Casquet et al. Reference Casquet, Rapela, Pankhurst, Galindo, Dahlquist, Baldo, Saavedra, González-Casado and Fanning2005, Reference Casquet, Pankhurst, Rapela, Galindo, Fanning, Chiaradia, Baldo, González-Casado and Dahlquist2008; Tholt, Reference Tholt2018; Webber, Reference Webber2018). However, evidence for high-grade metamorphism and deformation of Grenvillian age is also recognized (Porcher et al. Reference Porcher, Fernandes, Vujovich and Chernicoff2004; Casquet et al. Reference Casquet, Pankhurst, Fanning, Baldo, Galindo, Rapela, González-Casado and Dahlquist2006; Martin et al. Reference Martin, Collins and Spencer2019).
3. Sampling and petrography
Three samples from the MMS were collected for U–Pb SHRIMP zircon dating. One is a schist (MAZ-11032) from near Puesto de Maz, a homestead in the main E–W creek (Quebrada de Maz) that crosses the northern part of the sierra (Fig. 4). The schist contains quartz, biotite, muscovite, garnet, staurolite and plagioclase as framework minerals with accessory sillimanite, K-feldspar, monazite, zircon, allanite, tourmaline, apatite and ilmenite. The second sample is an almost pure white quartzite (MAZ-16223) collected from a small outcrop of metasedimentary rocks isolated by alluvial deposits in the same creek as the schist, on the dirt road to Puesto de Maz. This rock contains minor amounts of muscovite, chlorite (after biotite), sericitized plagioclase and accessory zircon and opaque minerals. The third sample (MAZ-12036), collected at the same locality as MAZ-11032, is from a thick grey metadacite bed with sharp contacts with the schists and concordant with foliation. This rock is interpreted as a dyke, probably related to the early Grenvillian arc magmatism (see Section 7). It is fine grained and consists of quartz, plagioclase (≈ An35), biotite, garnet, ilmenite, zircon and apatite. Coordinates of the three samples are 29° 11′ 10″ S, 68° 27′ 24″ W (MAZ-11032 and MAZ-12036), and 29° 11′ 32″ S, 68° 24′ 30″ W (MAZ-16223).
4. Analytical methods
U–Pb analyses were carried out using a sensitive high-resolution ion microprobe with reverse geometry (SHRIMP RG) at the Research School of Earth Sciences, The Australian National University, Canberra, Australia, following the methods of Williams (Reference Williams, McKibben, Shanks and Ridley1998 and references therein), as in Rapela et al. (Reference Rapela, Pankhurst, Casquet, Fanning, Baldo, González-Casado, Galindo and Dahlquist2007). Spot analyses were generally 25–30 μm. Data were reduced using ISOPLOT/Ex (Ludwig, Reference Ludwig2003). Analytical results and the reproducibility of the standard during the relevant analytical session are presented in online Supplementary Material Table S1. Errors for individual analyses are reported at one sigma level, those for averages at the 95 % confidence level. 204Pb-corrected 207Pb–206Pb ages were used, discarding those with common lead >5 %, discordance >10 % or age error (one sigma) >5 %.
Whole-rock powder of sample MAZ-12036 was analysed at Activation Laboratories Ltd (ACTLABS, Ontario, Canada) following the 4Lithores routine (https://actlabs.com/geochemistry/lithogeochemistry-and-whole-rock-analysis/lithogeochemistry/). Major elements were determined by inductively coupled plasma atomic emission spectroscopy, whereas minor and trace elements were determined by inductively coupled plasma mass spectrometry. Chemical data are shown in online Supplementary Material Table S2.
Rb–Sr and Sm–Nd isotope analyses were carried out at the Geochronology and Isotope Geochemistry Center (CIGC) of the Complutense University of Madrid on a Phoenix automated multi-collector mass spectrometer following analytical procedures as in Dahlquist et al. (Reference Dahlquist, Galindo, Morales Cámera, Moreno, Alasino, Basei and Macchioli Grande2020). Concentrations of Rb and Sr, as well as Rb/Sr ratios, were based on the chemical analysis from ACTLABS. Sm and Nd concentrations were determined by isotope dilution at CIGC. Three replicate analyses of the NBS-987 Sr-isotope standard yielded an average 87Sr/86Sr of 0.710256 ± 0.000005 (2σ) (accepted value 0.71025 ± 0.00005; Faure, Reference Faure2001), and the La Jolla Nd-isotope standard yielded an average 143Nd/144Nd ratio of 0.511848 ± 0.000004 (2σ) for six analyses (accepted value 0.511858 ± 0.00007; Lugmair & Carlson, Reference Lugmair and Carlson1978). Results are shown in online Supplementary Material Table S2.
5. Whole-rock chemical composition of metadacite MAZ-12036
The chemical composition of MAZ-12036 is: SiO2 = 67.08 %; Al2O3 = 14.5 %; Na2O = 2.72 %; K2O = 2.1 %; Rb = 72 ppm; Sr = 269 ppm; Zr = 206 ppm (online Supplementary Material Table S2), which corresponds to dacite (e.g. Middlemost, Reference Middlemost1985). The aluminium saturation index (ASI) is 1.17, i.e. peraluminous, as evidenced by garnet in the mineral assemblage. The chondrite-normalized rare earth element (REE) pattern is characterized by relatively high REE contents (total 186 ppm), with a (La/Yb)N of 4.4 and a moderate negative Eu anomaly of 0.7. The metadacite has a low 10 000*Ga/Al of 2.3, typical of S- and I-type granitoids.
This rock yielded a Nd model age TDM (single stage) of 1.5 Ga, which contrasts with the host metasedimentary rocks nearby (TDM = 2.5 to 2.0 Ga; Casquet et al. Reference Casquet, Pankhurst, Rapela, Galindo, Fanning, Chiaradia, Baldo, González-Casado and Dahlquist2008). The calculated 87Sr/86Sr at 1300 Ma is 0.7063 (online Supplementary Material Table S2).
6. Zircon U–Pb SHRIMP results
6.a. MAZ-11032: garnet-staurolite schist
Abundant zircon grains (c. 80 to 180 µm long) are rounded to sub-rounded. Almost all consist of a core and a surrounding mantle. Cores are sub-rounded (presumably of detrital origin) with variable internal structures. Mantles are thick, continuous and discordant to the core zoning; many show an internal zone of higher cathodoluminescence (CL) and an external one with lower CL, implying increasing U concentration as they crystallized. A few grains show a highly luminescent rim, too thin (<20 μm) to be analysed by SHRIMP. Dated spots (118) included mantles and cores, although in some cases the sampled area seems to have represented a mixture of both. Many of the analysed areas show significant discordance and scatter on a Wetherill Concordia plot, largely between c. 2 Ga and c. 1 Ga (Fig. 5a). This appears to be mainly the result of radiogenic-Pb loss during Grenvillian times, with slight additional loss during the Famatinian orogeny, or later, represented by the youngest apparent ages. Sixty of the measurements were sufficiently concordant to discriminate the times of zircon growth.

Fig. 5. U–Pb zircon data of schist MAZ-11032. (a) Wetherill plot and CL images of typical zircons with core(c)–mantle(m) arrangement. Grey ellipses correspond to rejected spots due to high discordance (>10 %). (b) Probability density plot of cores. (c) Th/U versus age diagram. (d) Probability density plot of mantles with two weighted mean ages corresponding to metamorphic events.
Most cores yield dates between 1.86 and 2.02 Ga, with four grains giving extrapolated Archaean dates at c. 2.7 and 3.1 Ga (Fig. 5b). The most prominent group shows an apparently bimodal peak at 1.98 and 2.01 Ga with a secondary peak at 1.86–1.90 Ga. The three youngest cores yielded a weighted mean age of 1860 ± 10 Ma (MSWD = 0.23).
Mantles gave ages between c. 970 and c. 1230 Ma: all have very low Th contents and low Th/U ratios (≤0.04; Fig. 5c), which together with the internal structure indicates a metamorphic origin (e.g. Rubatto, Reference Rubatto2017). Twenty-two mantles give a precise weighted mean age of 1037 ± 7 Ma (MSWD = 1.5), and four more give 1159 ± 15 Ma (MSWD = 0.8; Fig. 5d), suggesting two possible Grenvillian metamorphic events, the younger one resulting in more new zircon growth. The thin rims probably resulted from a Famatinian metamorphic overprint.
6.b. MAZ-16223: white quartzite
Zircon is abundant and has equant to prismatic forms with moderate aspect ratios (c. 80 to 160 µm long). Internally, the grains have rounded to sub-angular cores, presumably detrital, often with oscillatory or complex zoning shown by CL images. In most cases dark grey mantles, mostly homogeneous, surround the cores. As with MAZ-11032, many grains are further surrounded by a thin bright CL rim that follows the external boundary and even textural details of the grain such as embayments. Mantles are usually continuous but some of them were partially disrupted during growth of the outer rim.
Fifty-one areas were measured, of which 21 were discarded owing to discordance >10 %. Two groups of ages are distinguished (Fig. 6a): (a) concordant to slightly discordant Palaeoproterozoic to Archaean ages in cores, and (b) nearly concordant Grenvillian ages of c. 1080 to c. 1240 Ma determined in mantles. The core ages range widely, from c. 1840 to c. 3020 Ma, but with notable Palaeoproterozoic peaks at 2.08 and 2.42 Ga (Fig. 6b). The discordant pattern in Figure 6a suggests a significant Archaean population.

Fig. 6. U–Pb zircon data of quartzite MAZ-16223. (a) Wetherill plot and CL images of typical zircons with core(c)–mantle(m)–rim(r) arrangement. Grey ellipses correspond to rejected spots due to high discordance (>10 %). (b) Probability density plot of cores. (c) Th/U versus age diagram. (d) Probability density plot of mantles and weighted mean age of one metamorphic event.
Some of the mantles show a tendency to low Th/U ratios of c. 0.1 (Fig. 6c) and could represent metamorphic growth (e.g. Rubatto, Reference Rubatto2017). The distribution of mantle ages shows possibly discrete peaks at c. 1110 Ma and c. 1170 Ma (Fig. 6d). Only one bright rim was wide enough to analyse (spot no. 33.1), yielding an age of 461 ± 12 Ma, which presumably records Famatinian metamorphism.
6.c. MAZ-12036: metadacite
Zircon grains are mostly smaller than in the other samples, between 40 to 100 μm, and most exhibit a core with oscillatory zoning and a continuous dark CL mantle. Some grains have equant shapes and homogeneous low-luminescent CL internal structures. Thirty spots were dated, two of which were discarded owing to high discordance (>10 %). The Wetherill plot (Fig. 7a) distinguishes two groups, corresponding to cores and mantles (together with neoformed crystals).

Fig. 7. U–Pb zircon data of metadacite MAZ-12036. (a) Wetherill plot and CL images of typical zircons with core(c)–mantle(m) arrangement and neoformed crystals. Orange ellipses correspond to cores, purple to mantles and grey to rejected spots due to high discordance (>10 %). (b) Probability density plot of igneous cores and metamorphic mantles. (c) Th/U versus age diagram. (d) Weighted mean age of mantles corresponding to the metamorphic event.
The cores are mostly prismatic and show resorption to a variable extent. A few are fragments and oscillatory zoning is common. They have Th/U ratios in the range 0.3–0.6, which, along with zoning, is compatible with igneous zircon (e.g. Kirkland et al. Reference Kirkland, Smithies, Taylor, Evans and McDonald2015). Their ages range from c. 1140 to c. 1380 Ma with a broad peak in the density probability plot showing a maximum at c. 1190 Ma (Fig. 7b). The scattered older ages up to 1400 Ma are interpreted as inherited with respect to the dacite magma.
The mantles show low to very low Th/U ratios <0.04 (Fig. 7c, except no. 28.1: Th/U = 0.22 and 959 ± 51 Ma). Together with the weak zoning this suggests a metamorphic origin for the mantle zircon (e.g. Rubatto, Reference Rubatto2017). Ten of these analyses give a mean weighted age of 1033 ± 12 Ma (MSWD = 1.2; Fig. 7d). The two youngest ages (<1000 Ma) probably resulted from Pb loss during the Famatinian metamorphism. We conclude that the dacite crystallized between 1.38 and 1.19 Ga and underwent Grenvillian metamorphism at 1033 ± 12 Ma.
7. Discussion
7.a. Depositional age and metamorphism of the Maz Metasedimentary Series
The youngest detrital grains (zircon cores) found in the metasedimentary samples are 1855 ± 9 Ma (spot no. 3.2 in MAZ-11032) and 1837 ± 9 Ma (spot no. 50.1 in MAZ-16223), with a 1.86 Ga weighted mean age for the three youngest igneous zircons in MAZ-11032. If the Grenvillian metamorphic zircon mantles found in the three analysed samples grew in situ, as seems possible given their generally complete development around the igneous cores, then 1.86 Ga is the most probable maximum depositional age of the MMS.
Casquet et al. (Reference Casquet, Pankhurst, Fanning, Baldo, Galindo, Rapela, González-Casado and Dahlquist2006) found two zircon populations in sample MAZ-6063, a high-grade garnet schist (P = 780 ± 140 MPa, and T = 775 ± 95 °C) from the eastern edge of the Sierra de Maz that we correlate with the MMS. These corresponded to rather discordant core ages of 1.9–1.7 Ga and mantle metamorphic overgrowths peaking at c. 1230 Ma. Figure 8 shows the distribution of zircon ages attributed to metamorphism of the MMS, combining data from the three samples of this study with similarly filtered results published by Casquet et al. (Reference Casquet, Pankhurst, Fanning, Baldo, Galindo, Rapela, González-Casado and Dahlquist2006) for sample MAZ-6063. The pattern suggests three possible discrete tectonothermal events of Grenvillian age: 1234 ± 18 Ma, 1157 ± 11 Ma and 1035 ± 6 Ma. The oldest further sets a minimum value for the sedimentation age of the MMS. Moreover, the absence of significant Grenvillian igneous zircons common in syn- or post-Grenvillian sediments (Casquet et al. Reference Casquet, Pankhurst, Rapela, Galindo, Fanning, Chiaradia, Baldo, González-Casado and Dahlquist2008) suggests that the MMS pre-dates the Andean-type magmatism of 1.33–1.26 Ga recorded in the Sierra de Maz (Rapela et al. Reference Rapela, Pankhurst, Casquet, Baldo, Galindo, Fanning and Dahlquist2010). Crystallization of the metadacite MAZ-12036 is poorly constrained to between 1.38 and 1.19 Ga and does not help to specify the minimum age of sedimentation of the MMS. The dyke was then metamorphosed at 1033 ± 12 Ma, which coincides with the 1037 ± 7 Ma metamorphic age peak of schist MAZ-11032.

Fig. 8. Probability density plot of metamorphic zircon ages from our three samples (MAZ-11032, MAZ-16223 and MAZ-12036) together with mantles of MAZ-6063 (Casquet et al. Reference Casquet, Pankhurst, Fanning, Baldo, Galindo, Rapela, González-Casado and Dahlquist2006). Weighted mean ages were calculated at 1035 ± 5 Ma, 1157 ± 11 Ma and 1234 ± 18 Ma.
The ages of the Andean-type arc magmatism between 1.33 and 1.26 Ga proposed by Rapela et al. (Reference Rapela, Pankhurst, Casquet, Baldo, Galindo, Fanning and Dahlquist2010) and those of metamorphism recorded in the MMS are broadly coincident with those of tectonothermal events recorded in the Grenville Province of eastern Canada and its continuation in Mesoproterozoic Appalachian inliers (Rivers, Reference Rivers1997, Reference Rivers2012, Reference Rivers2015; McLelland et al. Reference McLelland, Selleck, Bickford, Tollo, Bartholomew, Hibbard and Karabinos2010). The latter similarity is remarkable because Laurentia was probably juxtaposed to southern South America cratons in the supercontinent Rodinia by the end of the Grenville orogeny (Hoffman, Reference Hoffman1991; Li et al. Reference Li, Bogdanova, Collins, Davidson, De Waele, Ernst, Fitzsimons, Fuck, Gladkochub, Jacobs, Karlstrom, Lu, Natapov, Pease, Pisarevsky, Thrane and Vernikovsky2008). However, correlation is hindered by our still limited knowledge and complications arising from the widespread Famatinian overprint.
In summary, we interpret the MMS as deposited after 1.86 Ga and before the Andean-type arc magmatism at 1.33–1.26 Ga recorded in the Maz Complex. Moreover, it is probable that deposition occurred between 1.86 and 1.80–1.75 Ga based on palaeogeographic and source areas considerations (see Section 7.b.3).
7.b. Provenance of the Maz Metasedimentary Series
Detrital zircon grains from the two samples of the MMS are Palaeoproterozoic (1.86 Ga and older) with only a few Archaean dates, and a notable absence of c. 1.3–1.0 Ga (Grenvillian) ages. Consequently, it is likely the sedimentary protoliths of the MMS were deposited within a basin that received sediment from Palaeoproterozoic continental basement prior to the onset of the Grenvillian-age magmatism and metamorphism recorded in the Maz Complex.
7.b.1. The Columbia (Nuna) supercontinent
There is growing evidence that most Palaeoproterozoic and Archaean cratons were contained in a supercontinent that was named Columbia (or Nuna) (Rogers & Santosh, Reference Rogers and Santosh2002; Meert, Reference Meert2002). Columbia was mainly amalgamated between 2.1 and 1.9 Ga by collisional orogenies. One of those collisional events of interest to this contribution is the 2.25–2.05 Ga Trans-Amazonian orogeny, recognized in the Maroní–Itacaiunas geological province of the Amazonia craton (Cordani & Teixeira, Reference Cordani, Teixeira, Hatcher, Carlson, McBride and Martinez Catalán2007). It is also recognized in other Palaeoproterozoic blocks in southern South America, such as the Río de la Plata craton (Hartmann et al. Reference Hartmann, Santos, Cingolani and McNaughton2002; Pankhurst et al. Reference Pankhurst, Ramos and Linares2003; Santos et al. Reference Santos, Hartmann, Bossi, Campal, Schipilov, Piñeyro and McNaughton2003; Rapela et al. Reference Rapela, Pankhurst, Casquet, Fanning, Baldo, González-Casado, Galindo and Dahlquist2007; Cingolani, Reference Cingolani2011). After amalgamation, Columbia was fringed with juvenile accretionary orogenic belts dated between c. 1.9 and 1.8 Ga (Chaves, Reference Chaves2020). The Amazonian Ventuari–Tapajós province is one of those accretionary orogens that has yielded ages between 2.0 and 1.8 Ga (Cordani & Teixeira, Reference Cordani, Teixeira, Hatcher, Carlson, McBride and Martinez Catalán2007).
The break-up of this supercontinent started at c. 1.6 Ga (Zhao et al. Reference Zhao, Sun, Wilde and Li2004), marked by widespread anorogenic magmatism (e.g. Anderson & Morrison, Reference Anderson, Morrison and Condie1992; Wiebe, Reference Wiebe and Condie1992; Åhäll & Connolly, Reference Åhäll and Connelly1998), and ended at c. 1.3–1.2 Ga through discrete mantle-plume related rifting events that produced mafic dyke swarms of global distribution (see review in Chaves, Reference Chaves2020). The dispersal of continental blocks away from Columbia concluded with a renewed amalgamation to form the Rodinia supercontinent, whose final assembly occurred during the collisional Rigolet event of the Grenvillian orogeny at c. 1.1–0.9 Ga (e.g. Li et al. Reference Li, Bogdanova, Collins, Davidson, De Waele, Ernst, Fitzsimons, Fuck, Gladkochub, Jacobs, Karlstrom, Lu, Natapov, Pease, Pisarevsky, Thrane and Vernikovsky2008; Rivers, Reference Rivers2015; Cawood et al. Reference Cawood, Strachan, Pisarevsky, Gladkochub and Murphy2016).
7.b.2. Position of the Maz Metasedimentary Series within Columbia
Columbia palaeogeography is still very speculative and the location of its constituent cratons remains uncertain, including those of South America (e.g. Amazonia and Río de la Plata) and West Africa. The South American cratons were placed south of Laurentia and adjacent to South Africa (Kalahari–Madagascar) by Rogers & Santosh (Reference Rogers and Santosh2002), whereas Zhao et al. (Reference Zhao, Cawood, Wilde and Sun2002) suggested that they were adjacent to Baltica and northeastern Laurentia. More recent palaeogeographic reconstructions place South America cratons near the southwestern continental margin of Columbia (Teixeira et al. Reference Teixeira, D’Agrella-Filho, Hamilton, Ernst, Girardi, Mazzucchelli and Bettencourt2013; Chaves, Reference Chaves2020).
Figure 9 shows a palaeogeographic model of Columbia at c. 1.79 Ga according to palaeomagnetic evidence by D’Agrella-Filho et al. (Reference D’Agrella-Filho, Trindade, Elming, Teixeira, Yokoyama, Tohver, Geraldes, Pacca, Barros and Ruiz2012) and Teixeira et al. (Reference Teixeira, D’Agrella-Filho, Hamilton, Ernst, Girardi, Mazzucchelli and Bettencourt2013), with Baltica and Amazonia attached to West Africa as in the SAMBA model of Johansson (Reference Johansson2009, Reference Johansson2014) and Bispo-Santos et al. (Reference Bispo-Santos, D’Agrella-Filho, Trindade, Janikian and Reis2014). This supercontinent reconstruction shows the location of the subduction zone that fringed Columbia immediately after assembly. The Río de la Plata craton is placed in one of the three possible palaeomagnetic locations proposed by Teixeira et al. (Reference Teixeira, D’Agrella-Filho, Hamilton, Ernst, Girardi, Mazzucchelli and Bettencourt2013) but slightly eastward in continuation with Rhyacian Amazonia. The Kalahari craton location is according to Chaves (Reference Chaves2020). In this scenario the MMS basin could well be located at the margin of Palaeoproterozoic Amazonia, coincident with the Rio Apa block position proposed by Teixeira et al. (Reference Teixeira, D’Agrella-Filho, Hamilton, Ernst, Girardi, Mazzucchelli and Bettencourt2013). In this interpretation the Amazonia, Río de la Plata and proto-Kalahari cratons, as well as Rio Apa, could be potential sources of sediments to the MMS basin. (As recommended by Jacobs et al. (Reference Jacobs, Pisarevsky and Thomas2008) the term proto-Kalahari is preferred to Kalahari. The first comprises an Archaean crustal core along with accreted Palaeoproterozoic rocks, which were assembled by c. 1750 Ma. The second involves crust formed in the Mesoproterozoic. However, we have retained the term Kalahari where used by other authors with the same meaning.) We assume here that MARA, i.e. the basement of the MMS of which the Rio Apa block was part (Casquet et al. Reference Casquet, Rapela, Pankhurst, Baldo, Galindo, Fanning, Dahlquist and Saavedra2012), and Amazonia were continuous in early Statherian times, in agreement with Teixeira et al. (Reference Teixeira, Cordani, Faleiros, Sato, Maurer, Ruiz and Azevedo2020).

Fig. 9. Palaeogeographic reconstruction of Columbia supercontinent modified from Teixeira et al. (Reference Teixeira, D’Agrella-Filho, Hamilton, Ernst, Girardi, Mazzucchelli and Bettencourt2013) and Chaves (Reference Chaves2020), showing the hypothetical location of the Maz Metasedimentary Series (MMS) basin. Black arrows show the probable direction of sediment transport. KAL – Kalahari; RA – Rio Apa; RPC – Río de la Plata craton.
7.b.3. Source areas of the Maz Metasedimentary Series
As the MMS was deposited after c. 1.86 Ga, and before the Andean-type magmatism recorded in the Maz Complex at 1.33–1.26 Ga, the issue arises as to whether the basin formed before, during or after the break-up of the Columbia supercontinent. In both metasedimentary samples analysed here the main zircon age population ranges between c. 1.86 and 2.08 Ga, with minor peaks at c. 2.24, 2.42 and 2.66–2.70 Ga and 2.9–3.1 Ga, although that at 2.42 Ga is only recognized in MAZ-16223.
Casquet et al. (Reference Casquet, Rapela, Pankhurst, Baldo, Galindo, Fanning, Dahlquist and Saavedra2012) proposed that the basement of the MMS, along with the Rio Apa outcrop in southern Brazil, and the Grenvillian Arequipa inlier in southern Peru were parts of a single Palaeoproterozoic block (MARA) on the basis of similar Nd TDM ages of c. 2.0 Ga. This block would fill the space south of Amazonia prior to collision of both (MARA and Amazonia) with Laurentia, and is an enlargement of the Arequipa–Antofalla basement of the central Andean margin of South America (Loewy et al. Reference Loewy, Connelly and Dalziel2004 and references therein). So far we have not found outcrops of the basement underlying the MMS in the Western Sierras Pampeanas. However, Palaeoproterozoic igneous rocks are exposed in the Rio Apa and Arequipa outcrops that could be potential sources of zircon grains in the overlying sedimentary cover. In the Rio Apa block, Teixeira et al. (Reference Teixeira, Cordani, Faleiros, Sato, Maurer, Ruiz and Azevedo2020) reported many U–Pb zircon ages from an igneous complex (granitoids) with peaks at 1.75–1.80 Ga, 1.82–1.87 Ga and 1.94–2.07 Ga. In Arequipa, orthogneisses of c. 1.79 Ga were recognized by Loewy et al. (Reference Loewy, Connelly and Dalziel2004) and Casquet et al. (Reference Casquet, Fanning, Galindo, Pankhurst, Rapela and Torres2010). The Rio Apa zircon ages between 1.82 and 2.07 Ga can be correlated with those found in the MMS between 1.86 and 2.08 Ga (Fig. 10). This group of ages is also found in the nearby Ventuari–Tapajós and Maroní–Itacaiunas provinces of the Amazonia craton (Cordani & Teixeira, Reference Cordani, Teixeira, Hatcher, Carlson, McBride and Martinez Catalán2007). However, the 1.75–1.80 Ga ages of Arequipa and Rio Apa granitoids are not found in the MMS, possibly suggesting that the MMS was deposited before that magmatic episode. In consequence, the depositional age could be as old as between 1.86 and 1.80–1.75 Ga. In fact, no detrital zircon has been found in the MMS corresponding to the Rio Negro–Jurena (1.82–1.60 Ga) or Rondonia–San Ignacio (1.59–1.30 Ga) orogenic belts between Amazonia and MARA (Teixeira et al. Reference Teixeira, Cordani, Faleiros, Sato, Maurer, Ruiz and Azevedo2020 and references therein).
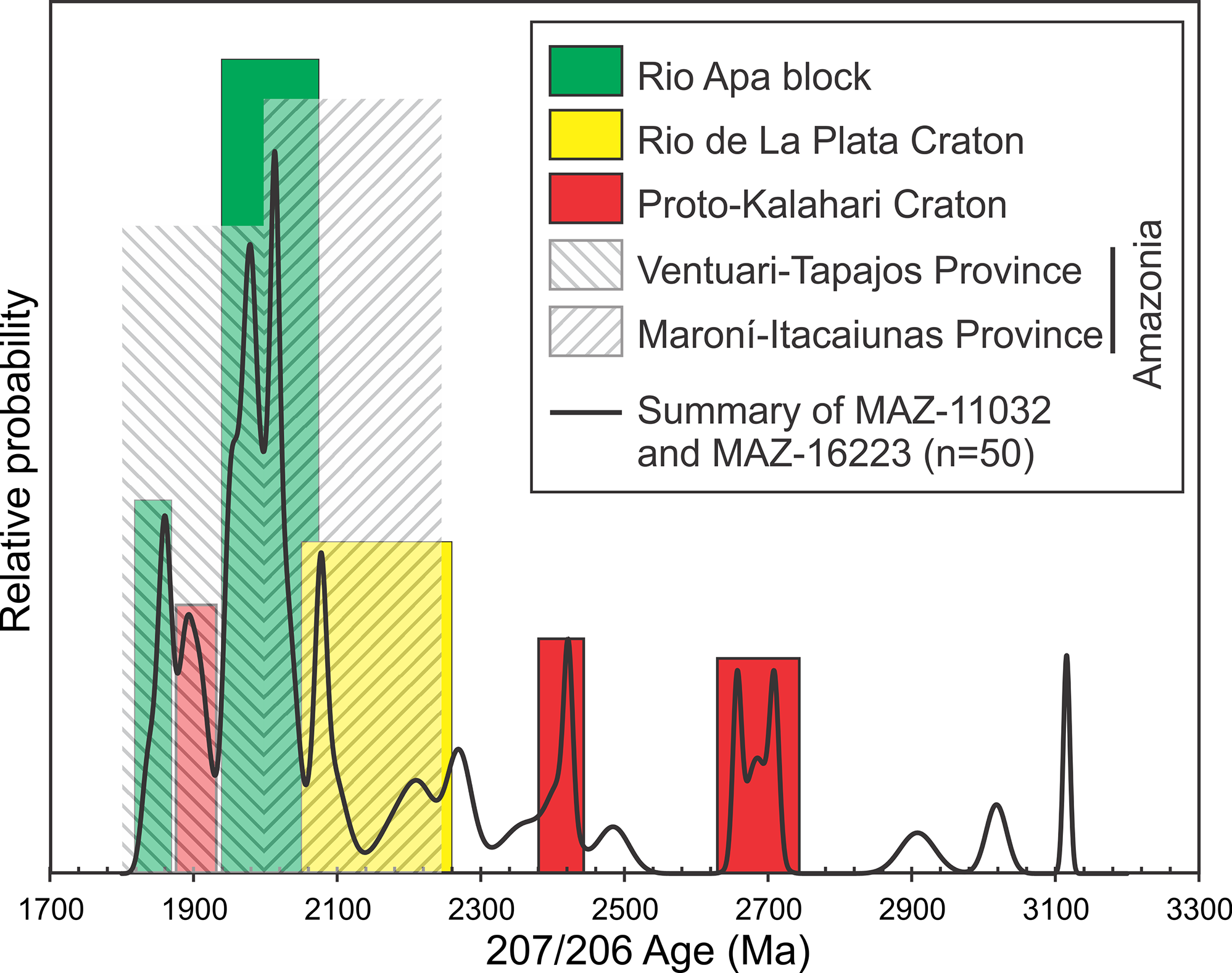
Fig. 10. Probability density plot of the Maz Metasedimentary Series detrital (cores) zircon ages. Dashed and coloured columns show age range of basements as probable sources of sediments.
The minor detrital zircon ages of c. 2.2 and 2.4 Ga in the MMS, most notable in the quartzite sample MAZ-16223, could not be sourced from either Rio Apa or Arequipa, where those ages have not been recorded. One likely source of c. 2.2 Ga zircons could be the Río de la Plata craton, another Palaeoproterozoic block in eastern Argentina and southern Uruguay. The Río de la Plata craton has yielded igneous zircon ages between 2.05 and 2.26 Ga equivalent to the Trans-Amazonian orogeny of the Amazonian Craton (Hartmann et al. Reference Hartmann, Santos, Cingolani and McNaughton2002; Rapela et al. Reference Rapela, Pankhurst, Casquet, Fanning, Baldo, González-Casado, Galindo and Dahlquist2007; Cingolani, Reference Cingolani2011 among many others) (Fig. 10). Also, the Maroní–Itacaiunas province of the Amazonia craton could have supplied the c. 2.2 Ga zircons (Cordani & Teixeira, Reference Cordani, Teixeira, Hatcher, Carlson, McBride and Martinez Catalán2007) considering the palaeogeographic reconstruction.
The c. 2.4 Ga age recognized in quartzite MAZ-16223 is not common in Palaeoproterozoic terranes. Zircon of this age is poorly recorded in Palaeoproterozoic terranes of southern South America, either because of poor preservation between major continental collisions (Hawkesworth et al. Reference Hawkesworth, Cawood, Kemp, Storey and Dhuime2009) or because it was a period of mafic magmatism and, in consequence, with minor zircon formation. This is probably also the case for the prominent c. 2.4 Ga peak of detrital zircon ages that Schröder et al. (Reference Schröder, Beukes and Armstrong2016) found in the Pretoria Group (Transvaal Supergroup; c. 2.06–2.65 Ga), a cover sequence on the Kaapvaal craton. The Duitschland Formation, at the base of the Pretoria Group, yields zircon ages with a main peak at 2.41–2.57 Ga, a secondary population at 2.69–2.81 Ga, and minor grains between 3.0 and 3.4 Ga. Remarkably, this pattern closely resembles that of the MMS for ages ≥2.4 Ga. Because Schröder et al. (Reference Schröder, Beukes and Armstrong2016) did not find exposures of equivalent-age volcanic rocks on the Kaapvaal craton, they invoked the Woongarra Rhyolite (c. 2.45 Ga) in the Pilbara craton of Western Australia, which was allegedly joined to the Kaapvaal craton at the time (Cheney, Reference Cheney1996; de Kock et al. Reference de Kock, Evans and Beukes2009; Schröder et al. Reference Schröder, Beukes and Armstrong2016 and references therein). More recently, however, mafic volcanism and dyking of the c. 2.4 Ga Ongeluk event has been recognized in the Kaapvaal and Zimbabwe cores of the composite proto-Kalahari craton (de Kock et al. Reference de Kock, Gumsley, Klausen, Söderlund, Djeutchou, Srivastava, Ernst and Peng2018). A potential source for the 2.4 Ga detrital zircon, and other grains older than this, in the MMS can thus be found in Southern Africa.
Figure 10 shows the coincidence of detrital zircon ages found in the MMS with sources in the Rio Apa block and the Río de la Plata, Amazonia and proto-Kalahari cratons. This suggests that sedimentation probably occurred before Columbia break-up. If so, available sources could be very variable, from Archaean to variably Palaeoproterozoic.
The extended region from the Sierras Pampeanas of Argentina and the Rio Apa block to the Arequipa block is covered by Phanerozoic sedimentary rocks and basement is not accessible. Thus, most of the hypothetical MARA block remains unknown and potential sedimentary sources of the MMS, other than those referred to above, could be still unrecognized.
8. Conclusions
The MMS consists of medium-grade garnet–staurolite–kyanite–sillimanite schists and quartzites, with minor amounts of marble and calc-silicate rocks. Transposed metadacite dykes have been recognized along with orthoamphibolites, metagabbros, metadiorites and orthogneisses. Deposition of the MMS sedimentary protoliths occurred after 1.86 Ga and before 1.33–1.26 Ga, and probably between 1.86 and 1.80–1.75 Ga. They were subsequently affected by Grenvillian metamorphic events at 1234 ± 18 Ma, 1157 ± 11 Ma and 1035 ± 6 Ma, and finally variably overprinted by the Silurian Famatinian metamorphism. Detrital zircons in the MMS are mainly Palaeoproterozoic, with a minor component of Meso-Neoarchaean origin. The diversity of peak detrital zircon ages indicates multiple basements sources, which is compatible with sedimentation in a basin along the Columbia (Nuna) supercontinent before its break-up. The main sources areas were probably the Rio Apa block, and the Río de la Plata, Amazonia and proto-Kalahari cratons, which have nearby locations in some Columbia reconstructions.
Acknowledgements
Funding was provided by Argentine public grants PUE 2016-CONICET-CICTERRA, CONICET PIP 2015 11220150100901CO, FONCYT PICT 2017 0619 and SECyT 2018-2020, and Spanish grants CGL2009-07984 from Ministerio de Ciencia e Innovación, and GR58/08 from UCM-Santander. This paper is a contribution to project CGL2016-76439-P of MINECO (Spain). We appreciate the help of all the colleagues of the PAMPRE research group, especially Carlos W. Rapela who participated in the U–Pb data acquisition. We acknowledge reviews and constructive comments by D. T. Brennan and an anonymous reviewer that led to improvements in the manuscript.
Supplementary material
To view supplementary material for this article, please visit https://doi.org/10.1017/S0016756821000935