1. Introduction
The Central Asian Orogenic Belt (CAOB), located between the Siberian Craton to the north and the North China and Tarim cratons to the south, is one of the largest accretionary orogenic belts on Earth. It is thought to have evolved through the accretion of microcontinents, island arcs, fore-arc and back-arc basins, ophiolites, oceanic seamounts and accretionary wedges (Fig. 1a; Xiao et al. Reference Xiao, Windley, Hao and Zhai2003, Reference Xiao, Windley, Huang, Han, Yuan, Chen, Sun, Sun and Li2009; Windley et al. Reference Windley, Alexeiev, Xiao, Kröner and Badarch2007; Kröner et al. Reference Kröner, Lehmann, Schulmann, Demoux, Lexa, Tomurhuu, Stipska, Liu and Wingate2010, Reference Kröner, Kovach, Belousova, Hegner, Armstrong, Dolgopolova, Seltmann, Alexeiev, Hoffmann, Wong, Sun, Cai, Wang, Tong, Wilde, Degtyarev and Rytsk2014; Safonova, Reference Safonova2017; Safonova et al. Reference Safonova, Kotlyarov, Krivonogov and Xiao2017; Furnes & Safonova, Reference Furnes and Safonova2019). The Inner Mongolia–Daxinganling Orogenic Belt (IMDOB) is the eastern extension of the CAOB within China; it is a key to understanding the tectonic evolution of the northern margin of the North China Craton (NCC) (Miao et al. Reference Miao, Fan, Liu, Zhang, Shi and Guo2008; Xu et al. Reference Xu, Zhao, Wang, Liao, Luo, Bao and Zhou2015).
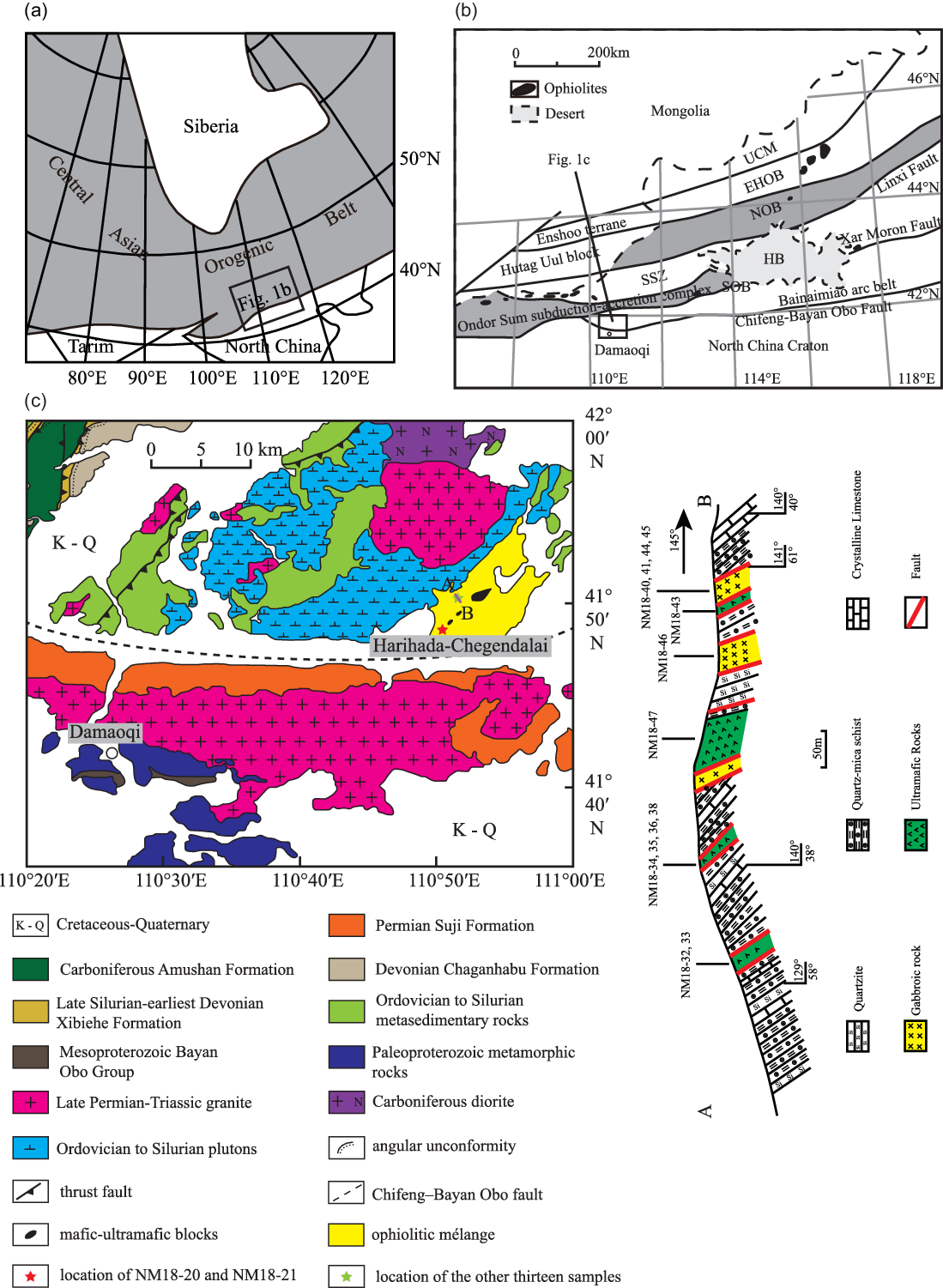
Fig. 1. (a) Simplified tectonic framework of the central-eastern CAOB (modified after Jahn, Reference Jahn, Malpas, Fletcher, Ali and Aitchison2004; Zhang et al. Reference Zhang, Li, Li, Tang, Chen and Luo2015). (b) Sketch geological map of the Inner Mongolia – Northern China tract (modified after Chen et al. Reference Chen, Zhang, Li, Chen, Tang and Li2015). Abbreviations: SOB – Southern Orogenic Belt; SSZ – Solonker Suture Zone; HB – Hunshandake block; NOB – Northern Orogenic Belt; EHOB – Erenhot–Hegenshan ophiolite belt; UCM – Uliastai continental margin. (c) Geological map of the Damaoqi area (modified after Zhang et al. Reference Zhang, Zhao, Ye, Liu and Hu2014) and geological section of the studied Harihada–Chegendalai ophiolitic mélange.
The Bainaimiao arc belt is located in the southern IMDOB; its tectonic affinity and early Palaeozoic tectonic evolution remain controversial (Xiao et al. Reference Xiao, Windley, Hao and Zhai2003; Jian et al. Reference Jian, Liu, Kröner, Windley, Shi, Zhang, Shi, Miao, Zhang, Zhang, Zhang and Ren2008; Xu et al. Reference Xu, Charvet, Chen, Zhao and Shi2013; Zhang et al. Reference Zhang, Zhao, Ye, Liu and Hu2014). Some researchers regard the Bainaimiao arc as an active continental margin formed by the southward subduction of the Palaeo-Asian Ocean beneath the NCC during early Palaeozoic time (Xiao et al. Reference Xiao, Windley, Hao and Zhai2003; Xu et al. Reference Xu, Charvet, Chen, Zhao and Shi2013; Li et al. Reference Li, Zhou, Li, Zhang, Liu, Zhao, Chen, Gu, Lin and Hu2016; Wu et al. Reference Wu, Liu, Zhu, Zhou, Jiang, Liu, Li, Wu and Ye2016). Some researchers consider the Bainaimiao arc to be a Japan-style island arc (Hu et al. Reference Hu, Xu and Niu1990; Jia et al. Reference Jia, Bao and Zhang2003). Recently, some researchers argued that the Bainaimiao arc is an exotic terrane that collided with the NCC after the northward subduction of the southern Bainaimiao Ocean (Zhang et al. Reference Zhang, Zhao, Ye, Liu and Hu2014; Eizenhöfer & Zhao, Reference Eizenhöfer and Zhao2018; Zhou et al. Reference Zhou, Pei, Zhang, Zhou, Xu, Wang, Cao and Yang2018 a; Ma et al. Reference Ma, Wang, Zhang and Sun2019; Liu et al. Reference Liu, Lai, Zhang, Zhu, Qin, Xiong and Wang2020).
Situated between the Bainaimiao arc and the NCC, the Harihada–Chegendalai area is important for understanding the relationship between the Bainaimiao arc and the NCC. Here, this study integrates geochronological, petrological and geochemical analyses of the gabbroic and ultramafic rocks from the Harihada–Chegendalai area in order to (1) constrain the age of the gabbroic rocks, (2) deduce the magma source and magmatic evolution of the gabbroic and ultramafic rocks, and (3) reveal the tectonic setting and the implications for the relationship between the Bainaimiao arc and the NCC.
2. Geological background and sample descriptions
The IMDOB can be divided into the Uliastai continental margin, the Erenhot–Hegenshan ophiolite accretionary belt, the Northern Orogenic Belt, the Solonker Suture Zone and the Southern Orogenic Belt from north to south (Fig. 1b; Xiao et al. Reference Xiao, Windley, Hao and Zhai2003; Xu et al. Reference Xu, Charvet, Chen, Zhao and Shi2013; Zhang, Z. C. et al. Reference Zhang, Li, Li, Tang, Chen and Luo2015, Reference Zhang, Chen, Li, Li, Yang and Qian2017; Ji et al. Reference Ji, Zhang, Chen, Li, Yang and Qian2018). The Southern Orogenic Belt is mainly composed of the Ondor Sum subduction–accretion complex and the Bainaimiao arc belt from north to south (Xiao et al. Reference Xiao, Windley, Hao and Zhai2003; Jian et al. Reference Jian, Liu, Kröner, Windley, Shi, Zhang, Shi, Miao, Zhang, Zhang, Zhang and Ren2008; Xu et al. Reference Xu, Charvet, Chen, Zhao and Shi2013).
The early to middle Palaeozoic Ondor Sum subduction–accretion complex is mainly composed of turbidites, ophiolitic mélanges, blueschists, plutons, metavolcanics, metasandstones and limestones; it is characterized by S-dipping foliations (Shao, Reference Shao1989, Reference Shao1991; Hu et al. Reference Hu, Xu and Niu1990; Tang, Reference Tang1992; Jian et al. Reference Jian, Liu, Kröner, Windley, Shi, Zhang, Shi, Miao, Zhang, Zhang, Zhang and Ren2008; Xu et al. Reference Xu, Charvet, Chen, Zhao and Shi2013; Li et al. Reference Li, Zhou, Li, Zhang, Liu, Zhao, Chen, Gu, Lin and Hu2016; Wu et al. Reference Wu, Liu, Zhu, Zhou, Jiang, Liu, Li, Wu and Ye2016; Zhang et al. Reference Zhang, Wei and Chu2018). The Ondor Sum subduction–accretion complex is unconformably overlain by Carboniferous limestones and Permian volcanic-sedimentary rocks (BGMRIM, 1991; Zhou et al. Reference Zhou, Zhao, Han and Wang2018 b). It has been suggested that the Ondor Sum Group was formed by the southward subduction of the Palaeo-Asian Ocean during early Palaeozoic time (Zhou et al. Reference Zhou, Zhao, Han and Wang2018 b). Blueschist-facies quartzite mylonites have exhibited phengite 40Ar–39Ar plateau ages of 453.2 ± 1.8 Ma and 449.4 ± 1.8 Ma (De Jong et al. Reference De Jong, Xiao, Windley, Masago and Lo2006), and glaucophanes from a blueschist have yielded 39Ar–40Ar ages of 446 ± 15 Ma and 426 ± 15 Ma (Tang & Zhang, Reference Tang, Zhang, Xiao and Tang1991). The plutons consist of minor Cambrian–Ordovician granitoids and Permian diorites, quartz diorites and granodiorites (BGMRIM, 1991; Xiao et al. Reference Xiao, Windley, Hao and Zhai2003; Zhou et al. Reference Zhou, Zhao, Han and Wang2018 b).
The Bainaimiao arc belt is bounded by the Ondor Sum subduction–accretion complex to the north, where the boundary comprises the Xar Moron fault, and is separated from the NCC by the E–W-trending Chifeng–Bayan Obo fault (Xiao et al. Reference Xiao, Windley, Hao and Zhai2003; Jian et al. Reference Jian, Liu, Kröner, Windley, Shi, Zhang, Shi, Miao, Zhang, Zhang, Zhang and Ren2008). The arc belt mainly consists of greenschist-facies–low-amphibolite-facies metasedimentary rocks, volcanic rocks and intermediate-acid intrusive rocks. These arc-related rocks are unconformably overlain by Silurian flysch deposits (Xuniwusu Formation) and Devonian continental molasse or quasi-molasse deposits (Xibiehe Formation; BGMRIM, 1991; Zhang et al. Reference Zhang, Su and Li2010; Zhang et al. Reference Zhang, Zhao, Ye, Liu and Hu2014; Zhang, Z. C. et al. Reference Zhang, Chen, Li, Li, Yang and Qian2017; Zhou et al. Reference Zhou, Zhao, Han and Wang2018 b; Fig. 1c).
The basement of the NCC is unconformably overlain by Mesoproterozoic rift-related volcanic rocks and lower Palaeozoic passive margin sediments. It consists of highly metamorphosed Archaean and Palaeoproterozoic rocks, including tonalite–trondhjemite–granodiorite rocks, high-K granite and diorite; it is intruded by late Palaeozoic plutons (Xiao et al. Reference Xiao, Windley, Hao and Zhai2003; Zhao et al. Reference Zhao, Sun, Wilde and Li2003; Zhang et al. Reference Zhang, Zhao, Song and Wu2004, Reference Zhang, Zhao, Song, Yang, Hu and Wu2007, Reference Zhang, Zhao, Song, Hu, Liu and Yang2009, Reference Zhang, Zhao, Ye, Liu and Hu2014; Zhai & Santosh, Reference Zhai and Santosh2011; Ma et al. Reference Ma, Chen, Chen and Niu2013, Reference Ma, Chen, Chen and Qu2014; Wu et al. Reference Wu, Liu, Zhu, Zhou, Jiang, Liu, Li, Wu and Ye2016).
The studied area is located at Harihada–Chegendalai in northeastern Damaoqi, north of the Chifeng–Bayan Obo fault. It lies between the NCC and the Bainaimiao arc belt (Fig. 1c). The Harihada–Chegendalai ophiolitic mélange is 15 km long and is composed of ophiolitic peridotites, pyroxene peridotites, gabbros, deep-water cherts and Ordovician–Silurian muscovite leptynites, marbles, quartzites, mica schists and plagioclase amphibole schists (Shao, Reference Shao1989, Reference Shao1991; Tang, Reference Tang1992; Zhang et al. Reference Zhang, Zhao, Ye, Liu and Hu2014).
Six gabbroic samples (NM18-21, NM18-40, NM18-41, NM18-44, NM18-45 and NM18-46) and nine ultramafic samples (NM18-20, NM18-32, NM18-33, NM18-34, NM18-35, NM18-36, NM18-38, NM18-43 and NM18-47) were collected from the Harihada–Chegendalai ophiolitic mélange (Fig. 1c). These gabbroic and ultramafic rocks were in fault contact with each other, and were also in fault contact with mica-quartz schists (Fig. 2a). The gabbroic samples consist of altered plagioclase (30–45 %), hornblende (25–55 %), epidote (5–10 %), chlorite (3–8 %), quartz (2–4 %) and opaque minerals (5–10 %; Fig. 2b). Sample NM18-20 is a harzburgite and contains orthopyroxene (35–40 %), serpentine (55–65 %) and chromite (5–10 %; Fig. 2c). The other ultramafic samples contain serpentine (85–90 %), carbonate minerals (1–10 %), chromite (3–7 %) and spinel (1–3 %; Fig. 2d). Although the degree of serpentinization is high, bastites with orthopyroxene pseudomorphs can be found, and the ultramafic protoliths were deduced to be harzburgites (Fig. 2d).

Fig. 2. (a) Contact relationship between gabbroic rocks and ultramafic rocks. (b) Gabbroic rock. (c) Serpentinized harzburgite. (d) Bastites with orthopyroxene pseudomorphs in a serpentinized peridotite. Abbreviations: Pl – plagioclase; Hb – hornblende; Opx – orthopyroxene; Serp – serpentine; Bas – bastite.
3. Analytical methods
3.a. Zircon U–Pb dating
Zircon grains were separated from gabbroic rock samples using conventional heavy liquid and magnetic separation techniques. They were manually picked under a binocular microscope. Randomly selected grains were mounted using epoxy resin and polished to expose their interiors. Cathodoluminescence (CL) images were obtained using a FEI Quanta 200F scanning electron microscope (SEM) at the Electron Microscopy Laboratory of Peking University. This permitted observation of the internal structures of the zircon grains. The U–Th–Pb isotope analyses were guided by reflected and transmitted light micrographs and CL images.
Samples NM18-21, NM18-40 and NM18-46 were chosen for zircon U–Pb dating. U–Pb dating and trace-element analyses of zircons were performed synchronously using a laser ablation inductively coupled plasma mass spectrometer (LA-ICP-MS) at the Key Laboratory of Orogenic Belts and Crustal Evolution, Peking University. Zircon 91500 was used as the external standard for age calibration, and the NIST 610 silicate glass was applied as an external standard to calculate concentrations. 29Si was the internal standard. Isotopic ratios, apparent ages and concentrations were calculated using GLITTER software (ver. 4.4.2, Macquarie University). The reported ages were calculated and concordia diagrams were made using Isoplot (version 4.15; Ludwig, Reference Ludwig2003). Details of the analytical methods can be found in Tang et al. (Reference Tang, Zhang, Li, Li, Chen and Luo2014).
3.b. In situ zircon Hf isotope analyses
Samples NM18-21, NM18-40 and NM18-46 were chosen for in situ zircon Hf isotopic analyses. Zircon Hf isotopic analyses were carried out on a Coherent Geolas HD laser-ablation system, attached to a Nu Plasma II multi-collector inductively coupled plasma mass spectrometer (MC-ICP-MS) at the Key Laboratory of Orogenic Belts and Crustal Evolution, Peking University. Readings were taken from the same zircons used in U–Pb dating, but different sites were used. A beam diameter of 60 μm and a pulse rate of 4 Hz were used during laser ablation with a laser beam energy of 10 J cm−2. Standards 91500, Penglai and Plešovice were used to calibrate the results.
The decay constant of 176Lu is 1.867 × 10−11 year−1 (Söderlund et al. Reference Söderlund, Patchett, Vervoort and Isachsen2004). The present-day 176Hf/177Hf and 176Lu/177Hf ratios of chondrite are 0.282785 and 0.0336, respectively (Bouvier et al. Reference Bouvier, Vervoort and Patchett2008). Depleted mantle reservoir has a present-day 176Lu/177Hf ratio of 0.0384 and a 176Hf/177Hf ratio of 0.28325 (Griffin et al. Reference Griffin, Belousova, Shee, Pearson and O’Reilly2004). The Hf depleted mantle model ages (TDM-Hf) were calculated by using the measured 176Lu/177Hf and 176Hf/177Hf ratios of the samples and the present-day 176Lu/177Hf and 176Hf/177Hf ratios for the depleted mantle.
3.c. Whole-rock geochemical analyses
Whole-rock samples were crushed and milled to ∼200 mesh. Major- and trace-element compositions of the samples were obtained from the Key Laboratory of Orogenic Belts and Crustal Evolution, Peking University, Beijing. The range of analytical uncertainty was monitored by analyses of Chinese national standard samples GSR-2 and GSR-3. Major oxides were analysed through X-ray fluorescence using a Jarrell-AshICAP 9000SP spectrometer on fused-glass discs. Loss on ignition (LOI) was determined using the gravimetric method. After acid digestion of whole-rock powders (50 mg) in Teflon bombs, trace elements were analysed by VGAXIOM MC-ICP-MS.
3.d. Mineral chemical analyses
Photos were taken using an environmental SEM under backscattered electron mode; the compositions of minerals were analysed using a JXA-8230 electron microprobe at Peking University. The operating conditions were a 15 kV accelerating voltage and a 10 nA beam current. The beam diameter was set to 1–2 μm. The PRZ correction method was used for standardization. The detailed analytical method can be found in Li et al. (Reference Li, Zhang, Wei, Slabunov and Bader2018).
4. Analytical results
4.a. Zircon U–Pb dating
Gabbroic samples (NM18-21, NM18-40 and NM18-46) were dated in this study. The results of LA-ICP-MS zircon U–Pb dating are listed in Table 1. Zircon grains from the three samples were colourless, stubby to elongate, euhedral to subhedral, and 160–320 μm in length with aspect ratios of 1.2–4. CL imaging revealed that they have straight and wide oscillatory growth bands (Fig. 3).
Table 1. LA-ICP-MS zircon U–Pb data for gabbroic rocks in Harihada–Chegendalai, northeastern Damaoqi

a Disc. = ((207Pb–235U age)/(206Pb–238U age) – 1) × 100.

Fig. 3. Cathodoluminescence images of representative zircon grains from (a) sample NM18-21, (b) sample NM18-40, and (c) sample NM18-46. Red and blue circles represent U–Pb and Lu–Hf analysed spots, respectively.
Zircons from sample NM18-21 exhibited varying U (76–218 ppm) and Th (35–113 ppm) concentrations with Th/U ratios of 0.39–0.85. All 30 zircons were concordant or nearly concordant, yielding apparent 206Pb–238U ages of 439–459 Ma, except one spot that exhibited an apparent 206Pb–238U age of 476 Ma; they formed a concordia age of 450 ± 2 Ma (Fig. 4a).

Fig. 4. LA-ICP-MS zircon U–Pb concordia diagrams and weighted average ages of (a) sample NM18-21, (b) sample NM18-40, and (c) sample NM18-46 (the white circles represent the concordia ages). (d) Correlations between ϵ Hf(t) values and ages of zircon grains of samples NM18-21, NM18-40 and NM18-46.
Zircons from sample NM18-40 showed U concentrations from 86 to 276 ppm, Th concentrations from 42 to 213 ppm and Th/U ratios from 0.44 to 0.89. All 30 zircons were concordant or nearly concordant, with apparent ages ranging from 437 to 460 Ma. They yielded a concordia age of 449 ± 1 Ma (Fig. 4b).
Zircons from sample NM18-46 displayed Th contents, U contents and Th/U ratios of 27–136 ppm, 62–293 ppm and 0.34–0.75, respectively. All 30 zircons were concordant or nearly concordant. Their apparent 206Pb–238U ages ranged from 436 to 462 Ma, forming a concordia age of 448 ± 1 Ma (Fig. 4c).
4.b. Zircon Lu–Hf isotopic data
Lu–Hf isotopic data of 30 zircons from samples NM18-21, NM18-40 and NM18-46 are listed in Table 2.
Table 2. Lu–Hf isotopic data for gabbroic rocks in Harihada–Chegendalai, northeastern Damaoqi

Ten zircon grains from sample NM18-21 yielded 176Hf/177Hf ratios of 0.282540–0.282601, 176Lu/177Hf ratios of 0.000459–0.001069 and ϵ Hf(t) values of 1.15–3.27, corresponding to TDM-Hf ages of 915 to 1002 Ma. Ten zircon grains from sample NM18-40 exhibited 176Hf/177Hf ratios of 0.282533–0.282608 and 176Lu/177Hf ratios of 0.000482–0.001444. The ϵ Hf(t) values are positive, ranging from 1.01 to 3.49, corresponding to a TDM-Hf range of from 901 to 1009 Ma. Ten zircons from sample NM18-46 showed 176Hf/177Hf ratios of 0.282531–0.282631 and 176Lu/177Hf ratios of 0.000295–0.001076. The ϵ Hf(t) values for these zircons are 0.87–4.34, corresponding to TDM-Hf ages of 872 to 1005 Ma. The correlations between the ϵ Hf(t) values and ages of these zircon grains are presented in Figure 4d.
4.c. Major and trace elements
The analytical results of the major and trace elements of 15 samples and standard samples GSR-2 and GSR-3 are listed in Table 3.
Table 3. Major- (wt %) and trace-element (ppm) data for gabbroic and ultramafic rocks in Harihada–Chegendalai, northeastern Damaoqi
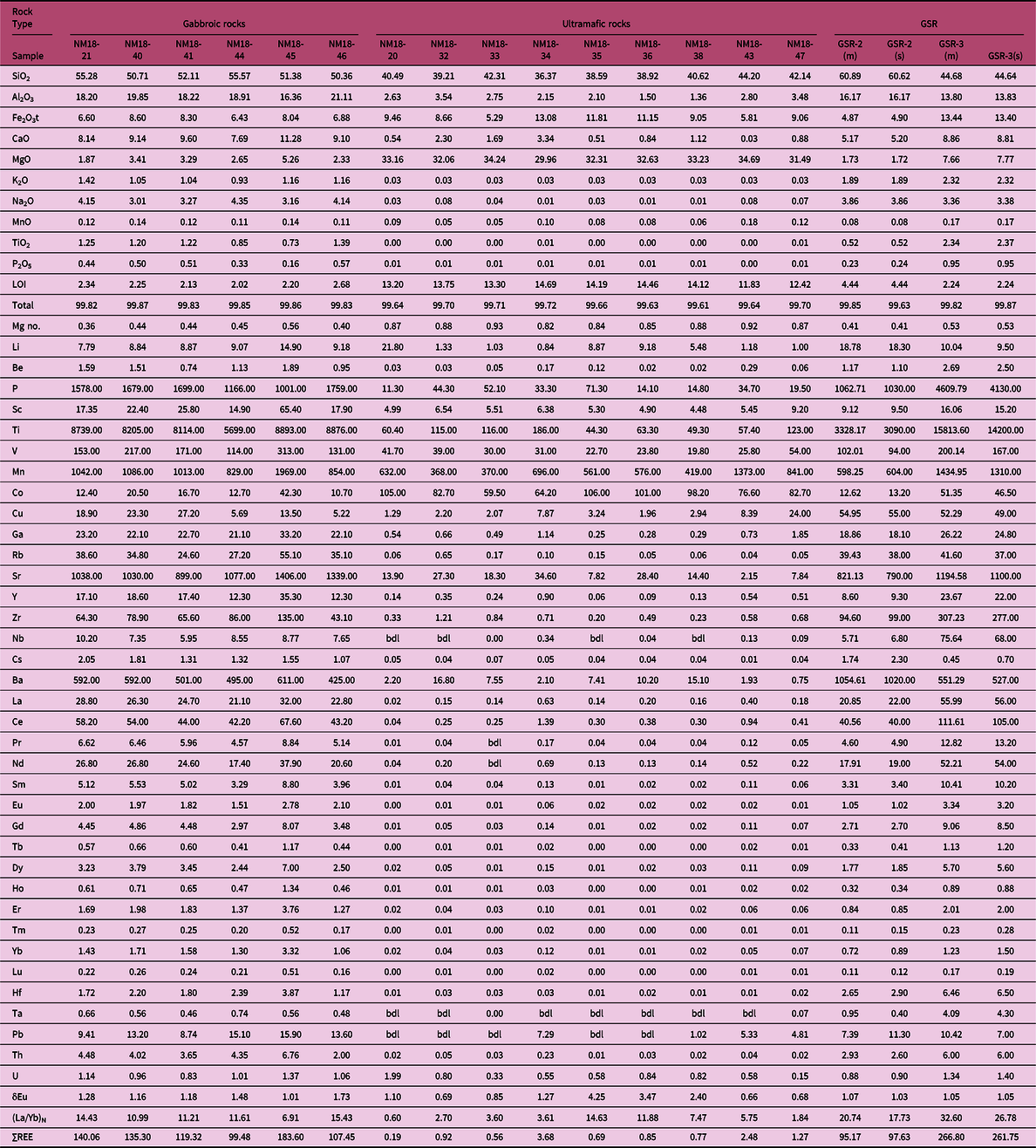
bdl – below detection limit; Mg no. = (MgO/40.3)/(MgO/40.3 + 0.9 * Fe2O3/71.84); δEu = EuN/(SmN * GdN)1/2; GSR-2(m) – measured data for GSR-2; GSR-2(s) – standard data for GSR-2; GSR-3(m) – measured data for GSR-3; GSR-3(s) – standard data for GSR-3.
4.c.1. Major elements
The gabbroic samples yielded moderate SiO2 (50.36–55.57 wt %) and K2O (0.93–1.42 wt %); low TiO2 (0.73–1.39 wt %); and high Na2O (3.01–4.35 wt %), Al2O3 (16.36–21.11 wt %), CaO (7.69–11.28 wt %), MgO (1.87–5.26 wt %) and total Fe2O3 (6.43–8.60 wt %); Mg no. values ranged from 0.36 to 0.56. The major elements of the gabbroic rocks were recalculated on an anhydrous basis before plotting. On the diagram of Zr/TiO2 versus Nb/Y, the gabbroic rocks mainly plotted in the field of basalt (Fig. 5a). On the K2O (wt %) versus SiO2 (wt %) diagram, six gabbroic samples plotted in the medium-K field (Fig. 5b).

Fig. 5. Geochemical classification plots for the gabbroic rocks: (a) Nb/Y v. Zr/TiO2 diagram (after Winchester & Floyd, Reference Winchester and Floyd1977; modified by Pearce, Reference Pearce and Wyman1996) and (b) SiO2 v. K2O diagram (Peccerillo & Taylor, Reference Peccerillo and Taylor1976).
The compositions of the ultramafic samples showed ranges of SiO2 = 36.37–44.20 wt %, Al2O3 = 1.36–3.54 wt %, total Fe2O3 = 5.29–13.08 wt %, CaO = 0.03–3.34 wt %, MgO = 29.96–34.69 wt %, K2O = 0.03 wt %, Na2O = 0.01–0.08 wt % and TiO2 = 0.001–0.01 wt %. The major elements are recalculated based on anhydrous ultramafic rocks. The Mg no. values of the ultramafic rocks range from 0.82 to 0.93, with an average of 0.87.
4.c.2. Trace elements
The primitive mantle-normalized trace-element patterns of six gabbroic samples showed enrichment in Rb, Ba, K, Pb and Sr, and depletion in Nb, Ta, Zr, Hf and Ti (Fig. 6a). The total rare earth element (ΣREE) concentrations of six gabbroic samples ranged from 99.48 ppm to 183.60 ppm, with an average of 130.87 ppm. The chondrite-normalized REE patterns showed enriched light rare earth elements (LREEs) and slightly depleted heavy rare earth elements (HREEs): the (La/Yb)N, (La/Sm)N and (Gd/Yb)N ratios were 6.91–15.43, 2.35–4.14 and 1.88–2.71, respectively. The gabbroic samples exhibited positive Eu anomalies (δEu = 1.01–1.73, average = 1.31; Fig. 6b).
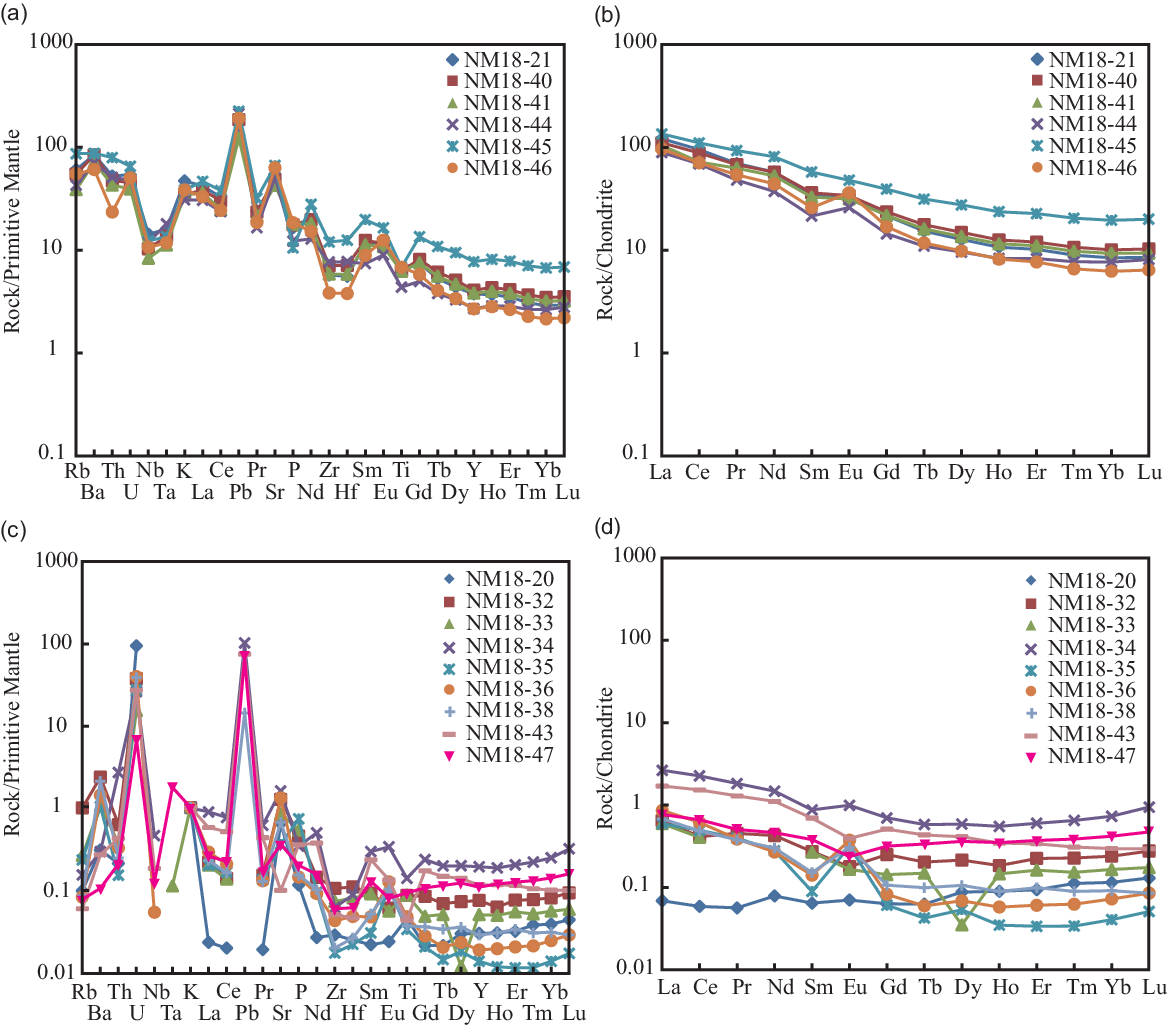
Fig. 6. Primitive mantle-normalized spider patterns and chondrite-normalized REE diagrams for (a, b) gabbroic rocks and (c, d) ultramafic rocks. Primitive mantle- and chondrite-normalized values are from Sun & McDonough (Reference Sun, McDonough, Saunders and Norry1989).
The primitive mantle-normalized trace-element patterns of the ultramafic samples were characterized by enrichment in fluid-mobile elements (e.g. Ba, U, K, Pb and Sr) and negative Nb, Ta, Zr and Hf anomalies (Fig. 6c). The chondrite-normalized REE diagrams show LREE-enriched profiles. Furthermore, the HREE profiles vary from being depleted to slightly enriched, with (La/Yb)N, (La/Sm)N and (Gd/Yb)N ratios of 0.60–14.63, 1.07–6.63 and 0.55–1.72, respectively. The ultramafic samples exhibited both positive and negative Eu anomalies (δEu = 0.66–4.25, average = 1.71; Fig. 6d).
4.d. Spinel chemistry
Fresh Cr-spinel cores and the alteration products of Cr-spinels in sample NM18-32 were analysed by electron probe micro-analyser (EPMA), the compositions were calculated on the basis of 32 oxygens (Jiang & Zhu, Reference Jiang and Zhu2020) and the results are listed in Table 4. Fresh Cr-spinel cores were enriched in Cr (47.73–55.81 wt % Cr2O3), with Cr no. values varying from 0.70 to 0.83 (av. 0.75). They exhibited Mg no. values varying between 0.28 and 0.40, with TiO2 contents of 0.02–0.21 wt % and Al2O3 contents of 7.5–14.66 wt %. The alteration products of the analysed Cr-spinels comprised ferritchromite and Cr-magnetite.
Table 4. Electron-microprobe analyses of Cr-spinels in sample NM18-32 (by EPMA, in wt %)
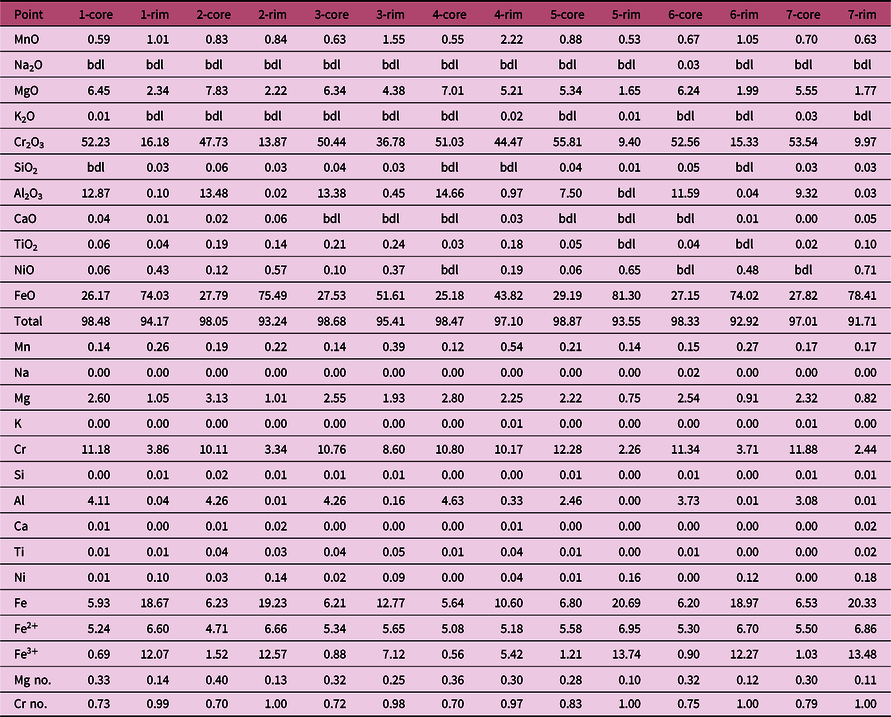
bdl – below detection limit; Cr no. = Cr/(Cr + Al) atomic ratio; Mg no. = Mg/(Mg + Fe2+) atomic ratio.
5. Discussion
5.a. Late Ordovician ophiolite suite between the Bainaimiao arc belt and the NCC
Previous studies have identified contemporary magmatic events in the Bainaimiao arc belt. Sensitive high-resolution ion microprobe (SHRIMP) zircon U–Pb dating yielded ages of 453.7 ± 3.1 Ma and 457.9 ± 2.6 Ma for a quartz diorite and a dacite in the Tulinkai area, respectively, and a diorite and two quartz diorite adakites yielded ages of 451.5 ± 2.9 Ma, 440.3 ± 2.4 Ma and 446.2 ± 2.2 Ma, respectively (Jian et al. Reference Jian, Liu, Kröner, Windley, Shi, Zhang, Shi, Miao, Zhang, Zhang, Zhang and Ren2008). Li et al. (Reference Li, Zhong, Xu, Song and Qu2012) reported a molybdenite Re–Os isochron age of 445 ± 3.4 Ma and a weighted 206Pb–238U mean age of 445 ± 6 Ma for a granodiorite porphyry intrusion in the Bainaimiao Cu–Mo deposit. The age of a Bainaimiao meta-volcanic rock was determined to be 449 Ma (Liu et al. Reference Liu, Liu and Zhou2014).
However, magmatic events in the southern margin of Bainaimiao arc belt have not been studied extensively. SHRIMP zircon U–Pb dating yielded ages of 452 ± 3 Ma, 446 ± 2 Ma and 440 ± 2 Ma for a diorite, a quartz diorite and a granodiorite sample in northern Damaoqi, respectively (Zhang & Jian, Reference Zhang and Jian2008). LA-ICP-MS zircon U–Pb dating yielded an age of 458 ± 2 Ma for a gabbroic diorite in the Damaoqi area (Zhou et al. Reference Zhou, Zhao, Han and Wang2018 b).
In this study, the zircon LA-ICP-MS U–Pb geochronology of three gabbroic samples yielded crystallization ages of 450 ± 2 Ma, 449 ± 1 Ma and 448 ± 1 Ma, indicating that the ophiolite suite formed during Late Ordovician time.
5.b. Petrogenesis of the gabbroic and ultramafic rocks
5.b.1. Assessment of element mobility
Zr is used as an alteration-independent index for geochemical variations because of its immobility during interactions between igneous rocks and hydrothermal fluids (Gibson et al. Reference Gibson, Kirkpatrick, Emmermann, Schmincke, Pritchard, Okay, Thorpe and Marriner1982; Pearce et al. Reference Pearce, Thirlwall, Ingram, Murton, Arculus, Van der Laan, Fryer, Pearce, Stokking, Ali, Arculus, Ballotti, Burke, Ciampo, Haggerty, Haston, Heling, Hobart, Ishii, Johnson, Lagabrielle, McCoy, Maekawa, Marlow, Milner, Motti, Murton, Phipps, Rigsby, Saboda, Stabell, van der Laan and Xu1992; Polat et al. Reference Polat, Hofmann and Rosing2002). Thus, correlations between trace elements and Zr were used to assess the mobility of trace elements.
Gabbroic samples exhibited LOI values of 2.02–2.68 and exhibited no obvious Ce anomalies (δCe = CeN/Sqrt(LaN × PrN)) (0.89–1.05). This indicates that the primary chemical signatures of the samples were not significantly affected by alteration and metamorphism (Polat & Hofmann, Reference Polat and Hofmann2003). The REEs and high field strength elements (HFSEs) of the gabbroic samples exhibited good correlations with Zr, indicating that these elements were not significantly affected by alteration processes, and thus can be used to discuss petrogenesis. Large-ion lithophile elements (LILEs) also showed correlations with Zr (online Supplementary Material Fig. S1). The results were the same when using correlations between trace elements and TiO2 to assess the mobility of the trace elements (online Supplementary Material Fig. S1; Furnes et al. Reference Furnes, Robins and De Wit2012). The above-mentioned findings are also supported by the nearly parallel patterns of the gabbroic samples on the chondrite-normalized REE and primitive mantle-normalized multi-element diagrams. This indicates that the primary chemical signatures of the gabbroic samples were not significantly obliterated.
Spinels in sample NM18-32 exhibited dark grey cores surrounded by light grey rims (Fig. 7a). The Cr–Al–Fe3+ triangular plot can be used to distinguish fresh and altered Cr-spinels (Fig. 7b; Azer et al. Reference Azer, Samuel, Ali, Gahlan, Stern, Ren and Moussa2013; Khalil et al. Reference Khalil, Obeid and Azer2014). Fresh Cr-spinel cores plotted along the Cr–Al join on this diagram. Ferritchromite and Cr-magnetite rims plotted along the Cr–Fe3+ join, indicating that there was a decrease in Al2O3 and an increase in Fe2O3. The conversion of Cr-spinels to ferritchromite and Cr-magnetite may have resulted from post-magmatic processes, such as serpentinization and ophiolite emplacement, and the cores remain unaffected (Barnes, Reference Barnes2000; Azer et al. Reference Azer, Samuel, Ali, Gahlan, Stern, Ren and Moussa2013; Kapsiotis et al. Reference Kapsiotis, Rassios, Uysal, Grieco, Akmaz, Saka and Bussolesi2018).

Fig. 7. (a) Spinels with fresh cores and altered rims, and the analysed sites. (b) Cr–Al–Fe3+ diagram of cores and rims of Cr-spinels from sample NM18-32.
5.b.2. Petrogenesis of the gabbroic rocks
Zircons from the gabbroic samples exhibited slightly positive ϵ Hf(t) values (0.87–4.34; Fig. 4d), reflecting the major contribution of the mantle and the limited involvement of the continental crust (Griffin et al. Reference Griffin, Wang, Jackson, Pearson, O’Reilly, Xu and Zhou2002). The Nb/La ratios of the gabbroic samples (0.24–0.41) indicate that they were derived from the lithospheric mantle (Smith et al. Reference Smith, Sanchez, Walker and Wang1999). The Sm/Yb versus Sm diagram suggests that the gabbroic rocks were sourced from the spinel–garnet transitional zone (Fig. 8). It has been suggested that (Th/Nb)PM >1 (Saunders et al. Reference Saunders, Storey, Kent, Norry, Storey, Alabaster and Pankhurst1992) and (Nb/La)PM <1 (Kieffer et al. Reference Kieffer, Arndt, Lapierre, Bastien, Bosch, Pecher, Yirgu, Ayalew, Weis, Jerram, Keller and Meugniot2004) are two reliable indicators of crustal contamination. The gabbroic rocks exhibited (Th/Nb)PM and (Nb/La)PM values of 2.20–6.47 and 0.23–0.39, respectively, indicating crustal contamination. On the chondrite-normalized diagrams, the gabbroic samples were slightly enriched in LREEs and slightly depleted in HREEs (Fig. 6b). On the primitive mantle-normalized spider diagrams, gabbroic samples were enriched in LILEs (e.g. Rb, Ba, U, K and Sr) and depleted in HFSEs (e.g. Nb, Ta, Zr, Hf and Ti; Fig. 6a). These characteristics indicate a subduction-related origin (Stern, Reference Stern2002; Pearce & Robinson, Reference Pearce and Robinson2010; Ma et al. Reference Ma, Zhong, Furnes, Zhaxi, Pang, Liu and Xia2021). On the Ce/Nb versus Ce diagram, the gabbroic rocks plotted near the field of sediments, indicating the contribution of sediments (Fig. 9a). The gabbroic rocks also exhibited the trends of sediments on the diagrams of Th/Yb versus Ba/La (Fig. 9b), Ba/Th versus Th (Fig. 9c) and U/Th versus Th (Fig. 9d). The positive anomaly in Eu (δEu = 1.01–1.73) indicates the accumulation of plagioclase (Huang & Frey, Reference Huang and Frey2003), which can be corroborated by the observed high Sr and Al2O3 contents of the gabbroic rocks. On the diagram of Th/Yb versus Nb/Yb, the gabbroic rocks plotted in the field of continental arcs (Fig. 10). It has been suggested that the Zr content of fore-arc basin basalt (FABB) is lower than that of mid-ocean ridge basalt (MORB) and back-arc basin basalt (BABB; Pearce & Norry, Reference Pearce and Norry1979). On the diagram of Zr/Y versus Zr, the gabbroic rocks mainly plotted in the FABB field (Fig. 11a). On the diagram of La/Nb versus Y, the gabbroic rocks mainly plotted in the FABB field or near the FABB field (Fig. 11b). Consequently, the gabbroic rocks could be derived from a mantle wedge in a fore-arc tectonic setting, metasomatized by subduction-derived melts from continental-derived sediments with continental crust contamination, and has experienced the accumulation of plagioclase.
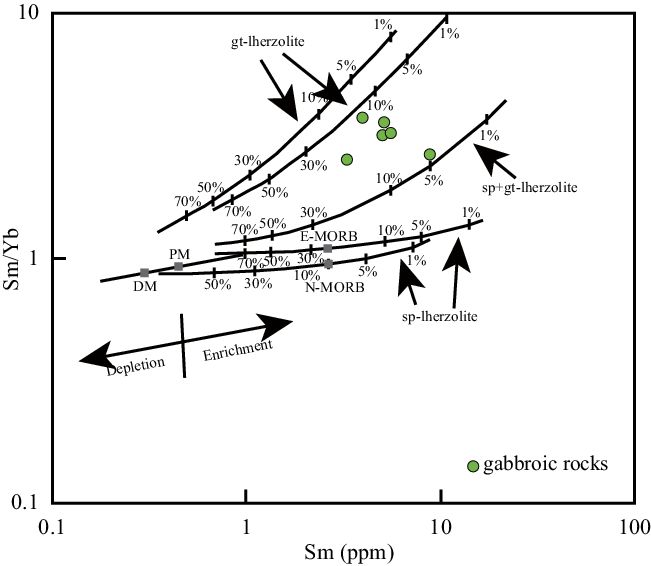
Fig. 8. Sm/Yb v. Sm diagram. Numbers along curves represent the degree of partial melting. The compositions of depleted mantle (DM), primitive mantle (PM), normal mid-ocean ridge basalt (N-MORB), enriched mid-ocean ridge basalt (E-MORB) and melting curves are from Aldanmaz et al. (Reference Aldanmaz, Pearce, Thirlwall and Mitchell2000).

Fig. 9. (a) Ce/Nb–Ce diagram for gabbroic rocks (Ma et al. Reference Ma, Zhong, Furnes, Zhaxi, Pang, Liu and Xia2021); data for depleted mantle (DM) and ocean-island basalt (OIB) are from Sun & McDonough (Reference Sun, McDonough, Saunders and Norry1989), and sediment data are from Plank & Langmuir (Reference Plank and Langmuir1998). (b) Th/Yb–Ba/La diagram for gabbroic rocks (Woodhead et al. Reference Woodhead, Hergt, Davidson and Eggins2001). (c) Ba/Th–Th diagram for gabbroic rocks (Hawkesworth et al. Reference Hawkesworth, Turner, McDermott, Peate and Calsteren1997). (d) U/Th–Th diagram for gabbroic rocks (Hawkesworth et al. Reference Hawkesworth, Turner, McDermott, Peate and Calsteren1997).

Fig. 10. (a) Th/Yb–Nb/Yb diagram for gabbroic rocks (Pearce, Reference Pearce2014). E-MORB – enriched mid-ocean ridge basalt; N-MORB – normal mid-ocean ridge basalt; OIB – ocean-island basalt; SZ – subduction zone.

Fig. 11. (a) Zr/Y–Zr diagram for gabbroic rocks (Ma et al. Reference Ma, Zhong, Furnes, Zhaxi, Pang, Liu and Xia2021). (b) La/Nb–Y diagram for gabbroic rocks (Thanh et al. Reference Thanh, Rajesh, Itaya, Windley, Kwon and Park2012). BABB – back-arc basin basalt; FABB – fore-arc basin basalt; IAT – island arc tholeiite; MORB – mid-ocean ridge basalt.
5.b.3. Petrogenesis of the ultramafic rocks
Harzburgites are depleted, refractory residual mantle peridotites that are formed by the partial melting of clinopyroxene-bearing harzburgites and lherzolites (Stern et al. Reference Stern, Reagan, Ishizuka, Ohara and Whattam2012). Depleted mantle can be found in fore-arc tectonic settings, because water can reduce the high melting temperature (Azer & Stern, Reference Azer and Stern2007; Khalil et al. Reference Khalil, Obeid and Azer2014). In primitive mantle-normalized spider diagrams, the enrichment of fluid-mobile elements results from metasomatism with fluids/melts, and low HFSE contents reflect high degrees of melt extraction (Deschamps et al. Reference Deschamps, Godard, Guillot and Hattori2013). Enrichment of LREEs indicates the interaction of peridotites with LREE-enriched melts/fluids, such as boninitic melts and crustal material (Sharma & Wasserburg, Reference Sharma and Wasserburg1996; Gruau et al. Reference Gruau, Bernard-Griffiths and Lecuyer1998; Parkinson & Pearce, Reference Parkinson and Pearce1998; Zhou et al. Reference Zhou, Robinson, Malpas, Edwards and Qi2005; Dai et al. Reference Dai, Wang, Hébert, Santosh, Li and Xu2011). The chemical compositions of chromian spinels can indicate different tectonic settings and magma processes (Dick & Bullen, Reference Dick and Bullen1984; Barnes & Roeder, Reference Barnes and Roeder2001; Kamenetsky et al. Reference Kamenetsky, Crawford and Meffre2001; Arai et al. Reference Arai, Okamura, Kadoshima, Tanaka, Suzuki and Ishimaru2011). The spinels from northeastern Damaoqi exhibited high Cr no. values (av. 0.75), indicating that they were formed in the suprasubduction zone tectonic setting, where the melts/fluids released from the subducted slab interacted with the mantle wedge, and the mantle peridotites experienced a high degree of partial melting (Roberts & Neary, Reference Roberts, Neary, Prichard, Alabaster, Harris and Neary1993; Büchl et al. Reference Büchl, Brügmann and Batanova2004; Zhou et al. Reference Zhou, Robinson, Su, Gao, Li, Yang and Malpas2014). The Cr no. values of the spinels were comparable to those of spinels from modern fore-arcs, and distinctly higher than those of spinels from the back-arc basin and mid-ocean ridge (MOR) peridotites (Azer & Stern, Reference Azer and Stern2007). The spinels plotted within the fore-arc peridotite field on the Cr no. versus Mg no. discrimination diagram (Fig. 12a). The contents of TiO2 in the analysed spinels reflect a magma-producing tectonic setting (Arai, Reference Arai1992; Arai et al. Reference Arai, Okamura, Kadoshima, Tanaka, Suzuki and Ishimaru2011; Arai & Miura, Reference Arai and Miura2016). The TiO2 versus Cr no. diagram suggests that the spinels may have been generated in fore-arc peridotites, which interacted with the boninitic melt (Fig. 12b). The Al2O3 wt % and TiO2 wt % contents of the spinels indicate a suprasubduction zone origin (Fig. 12c). On the Fe2+/Fe3+ atomic ratio versus Al2O3 wt % diagram, all of the spinels plotted in the suprasubduction zone peridotite field (Fig. 12d). The characteristics of the spinels and the whole-rock geochemistry of the peridotites jointly indicate that the peridotites may have been residual mantle which has experienced a high degree of partial melting and has interacted with fluids/melts released from a subducted slab in a fore-arc tectonic setting.

Fig. 12. (a) Cr no.–Mg no. plot for fresh Cr-spinels (after Stern et al. Reference Stern, Johnson, Kröner and Yibas2004). (b) Relationship between Cr no. and TiO2 content in fresh Cr-spinels (after Tamura & Arai, Reference Tamura and Arai2006). (c) TiO2–Al2O3 diagram for fresh Cr-spinels (after Kamenetsky et al. Reference Kamenetsky, Crawford and Meffre2001). (d) Fe2+/Fe3+ atomic ratio–Al2O3 wt % plot for fresh Cr-spinels (after Kamenetsky et al. Reference Kamenetsky, Crawford and Meffre2001). LIP – large igneous province; MOR – mid-ocean ridge; MORB – mid-ocean ridge basalt; OIB – ocean-island basalt; SSZ – suprasubduction zone.
5.c. Tectonic implications
Previous studies have suggested that, from north to south, the Ondor Sum subduction–accretion complex, Bainaimiao arc and Xuniwusu Formation form a trench–arc–basin system. This system was thought to have resulted from the southward subduction of the Palaeo-Asian Ocean beneath the NCC (Xiao et al. Reference Xiao, Windley, Hao and Zhai2003; Zhang, J. F. et al. Reference Zhang, Liu, Guan, Xu, Wang and Zhu2017). Located 133 km ENE of the studied area, the Xuniwusu Formation was regarded as a back-arc basin deposit because of its location, flysch sedimentary characteristics and detrital zircon ages (Zhang, J. F. et al. Reference Zhang, Liu, Guan, Xu, Wang and Zhu2017).
Both the gabbroic rocks and peridotites were formed in a fore-arc tectonic setting. Furthermore, they crop out together in the schists, so they can be considered as part of the Harihada–Chegendalai ophiolitic mélange. Ophiolites are defined as ‘suites of temporally and spatially associated ultramafic, mafic and felsic rocks related to separate melting episodes and processes of magmatic differentiation in particular oceanic environments’ (Dilek & Furnes, Reference Dilek and Furnes2011). They are interpreted to be the remnants of ancient oceanic crust and upper mantle (Dilek & Furnes, Reference Dilek and Furnes2014). Ophiolites can be classified based on the geochemical fingerprints of their mafic lavas and dykes (Dilek & Furnes, Reference Dilek and Furnes2011, Reference Dilek and Furnes2014; Furnes & Dilek, Reference Furnes and Dilek2017; Furnes et al. Reference Furnes, Dilek, Zhao, Safonova and Santosh2020). As mentioned above, the gabbroic rocks and peridotites analysed in this study are part of a suprasubduction zone ophiolitic mélange; their geochemical characteristics indicate that they were formed in a fore-arc tectonic setting. In recent years, some studies have proposed that there was a South Bainaimiao Ocean between the exotic Bainaimiao arc and the NCC, and that the northward subduction of the South Bainaimiao Ocean resulted in the collision between the Bainaimiao arc and the NCC during late Silurian to early Carboniferous times (Zhang et al. Reference Zhang, Zhao, Ye, Liu and Hu2014; Eizenhöfer & Zhao, Reference Eizenhöfer and Zhao2018; Zhou et al. Reference Zhou, Pei, Zhang, Zhou, Xu, Wang, Cao and Yang2018 a; Ma et al. Reference Ma, Wang, Zhang and Sun2019). The northern NCC was a passive continental margin during early Palaeozoic time, and the Bainaimiao arc has been shown to have a different basement from that of the NCC (Zhang et al. Reference Zhang, Zhao, Ye, Liu and Hu2014). The Xuniwusu Formation can be divided into three sediment cycles; the provenances of the first two sediment cycles comprise solely the Bainaimiao arc (Zhang, J. F. et al. Reference Zhang, Liu, Guan, Xu, Wang and Zhu2017). In the last sediment cycle, the Xuniwusu Formation was considered to have received detritus from both the Bainaimiao arc and the NCC, deduced from the detrital zircon ages ranging from Precambrian to Silurian, with the Precambrian detrital zircons considered to have been derived from the NCC (Zhang, J. F. et al. Reference Zhang, Liu, Guan, Xu, Wang and Zhu2017). However, the Bainaimiao arc belt also has Precambrian basement, which could have provided detritus to the Xuniwusu Formation. It is also possible that the Xuniwusu Formation received detritus from the NCC because of the shortening of the South Bainaimiao Ocean (Chen et al. Reference Chen, Zhang, Qian, Li, Ji and Wu2020). The Harihada–Chegendalai ophiolitic mélange may have formed in a fore-arc setting, resulting from the northward subduction of the South Bainaimiao Ocean during Late Ordovician time (Fig. 13).

Fig. 13. Tectonic evolution model of the Bainaimiao arc and the north margin of the North China Craton (modified after Zhang et al. Reference Zhang, Zhao, Ye, Liu and Hu2014).
6. Conclusions
-
(1) Based on the whole-rock geochemical characteristics, the Late Ordovician (c. 448–450 Ma) gabbroic rocks in the Harihada–Chegendalai area (northern Damaoqi) were deduced to have been derived from a mantle wedge that was metasomatized by subduction-derived fluids/melts with continental crust contamination.
-
(2) According to the whole-rock geochemical characteristics and mineral chemical characteristics, the ultramafic rocks were deduced to comprise part of the depleted residual mantle, which experienced a high degree of partial melting and interacted with fluids/melts released from a subducted slab.
-
(3) The ultramafic and gabbroic rocks were found to be in fault contact with each other, and to occur together in quartz-mica schists. They comprise part of the Harihada–Chegendalai ophiolitic mélange, which formed in a fore-arc tectonic setting.
-
(4) Combined with the results of previous studies, the evidence presented here suggests that the South Bainaimiao Ocean may have subducted northward beneath the Bainaimiao arc during Late Ordovician time, resulting in the formation of the Harihada–Chegendalai fore-arc ophiolitic mélange.
Acknowledgements
We are grateful to Dr Guido Meinhold, Prof. Harald Furnes, Dr Qiangtai Huang and an anonymous reviewer for their precious comments and suggestions. We thank Prof. Shuguang Song and Dr Zeng Lü for their constructive proposals. This study is financially supported by the National Key Research and Development Project of China (grant no. 2017YFC0601302) and the National Key Basic Research Programme of China (grant no. 2013CB429801).
Supplementary material
To view supplementary material for this article, please visit https://doi.org/10.1017/S0016756821000662