1. Introduction
A complex array of different mechanisms and processes controls the growth and demise of marine carbonate depositional systems (Tucker & Wright, Reference Tucker and Wright1990; Jones & Desrochers, Reference Jones, Desrochers, Walker and James1992; Wright & Burchette, Reference Wright, Burchette and Reading1996; Schlager, Reference Schlager2005; Warrlich, Bosence & Waltham, Reference Warrlich, Bosence and Waltham2005; Wright & Burgess, Reference Wright and Burgess2005; Pomar & Kendall, Reference Pomar, Kendall, Lukasik and Simo2008; Tresch & Strasser, Reference Tresch and Strasser2011; Williams et al. Reference Williams, Burgess, Wright, Della Porta and Granjeon2011; Pomar et al. Reference Pomar, Bassant, Brandano, Ruchonnet and Janson2012; Wilson, Reference Wilson2012). The major extrinsic factors are geotectonics and climate, which together control eustatic sea level, and also prevent massive siliciclastic supply to depositional basins by determining the hinterland topography, river drainage, weathering, and erosion patterns (Tucker & Wright, Reference Tucker and Wright1990, p. 28). Tectonic regime and sea-level oscillations control the size, morphology and thickness of carbonate platforms by modifying base-level changes and accommodation space. Climate, along with geotectonics, is important for marine carbonate sedimentation by regulating temperature, salinity, water circulation, nutrient supply, current regime and wave activity (Tucker & Wright, Reference Tucker and Wright1990, p. 31). These environmental conditions (plus oxygenation levels, light penetration, substrate) and biological evolution together control the distribution of biota across the carbonate platform and its contribution to the carbonate factory, i.e. sedimentation rate (Pomar & Hallock, Reference Pomar and Hallock2008). Consequently, accommodation in carbonate systems has two interrelated components: physical accommodation, i.e. hydrodynamic conditions, and ecological accommodation, i.e. competence to build up (Pomar, Reference Pomar2001 a; Pomar & Kendall, Reference Pomar, Kendall, Lukasik and Simo2008). In addition, the chemistry of seawater (Mg/Ca ratio, Ca concentration, carbonate saturation state) strongly influences precipitation of both skeletal and abiotic carbonates (Stanley & Hardie, Reference Stanley and Hardie1998; Stanley, Reference Stanley2008; Ries, Reference Ries2010). Recently, Williams et al. (Reference Williams, Burgess, Wright, Della Porta and Granjeon2011) pointed out that stratal geometries of carbonate platforms result from a multitude of interacting controls, including sediment production and transport, differential subsidence effects, antecedent topography and relative sea-level changes.
According to the original definition of Ahr (Reference Ahr1973), carbonate ramps are characterized by gentle depositional slopes (normally less than 1°) passing gradually from a shallow high-energy environment to a deeper low-energy environment. Later, Read (Reference Read1982, Reference Read1985) distinguished two types of ramps: homoclinal (with uniform gradient from shoreline to deeper water) and distally steepened (with offshore slope break between the shallow ramp and the basin). The main characteristics of the few modern examples (e.g. Light & Wilson, Reference Light, Wilson, Wright and Burchette1998; Testa & Bosence, Reference Testa, Bosence, Wright and Burchette1998; Hine et al. Reference Hine, Brooks, Davis, Duncan, Locker, Twichell and Gelfenbaum2003; James et al. Reference James, Bone, Kyser, Dix and Collins2004; Gischler & Lomando, Reference Gischler and Lomando2005; Riegl et al. Reference Riegl, Poiriez, Janson, Bergman, Westphal, Riegl and Eberli2010) and many ancient counterparts (see summaries in Burchette & Wright, Reference Burchette and Wright1992; Wright & Burchette, Reference Wright, Burchette, Wright and Burchette1998) have been thoroughly investigated. The general zonation pattern proposed by Burchette & Wright (Reference Burchette and Wright1992), i.e. inner ramp, mid-ramp, outer ramp and basin depositional zones, has been commonly applied for most carbonate ramp successions. Despite significant progress, there is still a lack of detailed understanding about what processes and controls lead to the formation and maintenance of ramp morphology in contrast to flat-topped platforms and rimmed shelves (Burchette & Wright, Reference Burchette and Wright1992; Aurell et al. Reference Aurell, Bádenas, Bosence, Waltham, Wright and Burchette1998; Pomar, Reference Pomar2001 b; Schlager, Reference Schlager2005; Wright & Burgess, Reference Wright and Burgess2005; Pomar & Kendall, Reference Pomar, Kendall, Lukasik and Simo2008; Williams et al. Reference Williams, Burgess, Wright, Della Porta and Granjeon2011; Pomar et al. Reference Pomar, Bassant, Brandano, Ruchonnet and Janson2012). Further problematic issues include the specific controls on ramp formation in different geotectonic settings (Burchette & Wright, Reference Burchette and Wright1992; Bosence, Reference Bosence2005) and the distinction between factors that predetermine the configuration of homoclinal versus distally steepened ramps (Burchette & Wright, Reference Burchette and Wright1992; Pomar, Reference Pomar2001 b; Pomar & Kendall, Reference Pomar, Kendall, Lukasik and Simo2008; Williams et al. Reference Williams, Burgess, Wright, Della Porta and Granjeon2011). Another key question is how climate influences ramp formation (Read, Reference Read, Wright and Burchette1998), for example, why the majority of cool-water carbonates develop into carbonate ramp profiles (e.g. Jones & Desrochers, Reference Jones, Desrochers, Walker and James1992; Pedley & Carannante, Reference Pedley, Carannante, Pedley and Carannante2006). Mud production, which was especially extensive in tropical ramps and characterized in particular the offshore environments of many early to middle Mesozoic ramps (Burchette & Wright, Reference Burchette and Wright1992; Wright & Burchette, Reference Wright, Burchette, Wright and Burchette1998; Pomar & Hallock, Reference Pomar and Hallock2008), is also enigmatic and its origin has been much debated (Aurell et al. Reference Aurell, Bádenas, Bosence, Waltham, Wright and Burchette1998; Török, Reference Török, Wright and Burchette1998; Pomar & Hallock, Reference Pomar and Hallock2008; Turpin et al. Reference Turpin, Gressier, Bahamonde and Immenhauser2014). Also, there is growing insight into Proterozoic analogues and their comparison to Phanerozoic ramps in terms of size, general evolution, specific lithofacies, cyclicity, and dolomitization patterns (e.g. Sherman, Narbonne & James, Reference Sherman, Narbonne and James2001; Cozzi, Grotzinger & Allen, Reference Cozzi, Grotzinger and Allen2004; Dibenedetto & Grotzinger, Reference Dibenedetto and Grotzinger2005; Zentmyer et al. Reference Zentmyer, Pufahl, James and Hiatt2011; Delpomdor, Kant & Préat, Reference Delpomdor, Kant and Préat2014; Thomson, Rainbird & Dix, Reference Thomson, Rainbird and Dix2014; Adnan et al. Reference Adnan, Shukla, Verma and Shukla2015).
This paper focuses on the various extrinsic (allogenic) and intrinsic (autogenic) controls on the birth, growth and demise of a Triassic carbonate ramp which existed in the western part of the Tethys Ocean. The study is mainly based on sedimentological data obtained from 20 stratigraphic sections of the ramp succession which is exposed in the Western Balkanides (NW Bulgaria). Additionally, some previous results obtained by the author and other workers are also widely used and interpreted. An attempt is made to distinguish and separately discuss global, regional and local controls on the specific evolution of the ramp system. The autonomous effect and interplay of those factors are analysed in the light of their influence on the sedimentation style, stacking pattern, slope gradient, sediment thickness, facies distribution and biota development. Special emphasis is placed on the new evidence for local distal steepening of the ramp profile, dominant control on the formation of peritidal cyclicity, and the factors that caused demise of the carbonate system.
Ramps were typical carbonate platforms in the Phanerozoic as the Early to Middle Triassic interval was a time of their extensive development worldwide, and especially across the Western Tethys realm and in adjacent Peri-Tethyan basins (Burchette & Wright, Reference Burchette and Wright1992; Kiessling, Flügel & Golonka, Reference Kiessling, Flügel and Golonka2003; Pomar & Hallock, Reference Pomar and Hallock2008). Therefore, unravelling the controls on Triassic analogues may comprehensively elucidate the general origin and specific evolution of ramp systems. Because carbonate ramps that formed during greenhouse, icehouse and transitional times have apparently distinctive characteristics (Read, Reference Read, Wright and Burchette1998), and because the Triassic period was a global greenhouse period (Sellwood & Valdes, Reference Sellwood and Valdes2006; Preto, Kustatscher & Wignall, Reference Preto, Kustatscher and Wignall2010), this study may contribute to improving our knowledge of ramps formed under warm climatic conditions. Another point of interest is the evidence presented for locally steepened ramp morphology which would allow comparison to other known examples from the Proterozoic and Phanerozoic sedimentary record (e.g. Gawthorpe, Reference Gawthorpe1986; Stanton & Flügel, Reference Stanton and Flügel1995; Hips, Reference Hips, Wright and Burchette1998; Pedley, Reference Pedley, Wright and Burchette1998; Sherman, Narbonne & James, Reference Sherman, Narbonne and James2001; Pomar, Obrador & Westphal, Reference Pomar, Obrador and Westphal2002; Cozzi, Grotzinger & Allen, Reference Cozzi, Grotzinger and Allen2004; Dilliard et al. Reference Dilliard, Pope, Coniglio, Hasiotis and Lieberman2010; Martin-Rojas et al. Reference Martin-Rojas, Somma, Delgado, Estévez, Iannace and Zamparelli2012; Thomson, Rainbird & Dix, Reference Thomson, Rainbird and Dix2014).
2. Brief synopsis of the Triassic ramp carbonates from the Western Balkanides
The Triassic sedimentary succession exposed in NW Bulgaria (Fig. 1) was assigned to the Balkanide type of Triassic (Ganev, Reference Ganev1974) which follows the classical tripartite subdivision of the Triassic system in the Peri-Tethyan Germanic Basin, i.e. continental Buntsandstein, marine Muschelkalk and continental Keuper. The whole succession corresponds to a symmetrical second-order transgressive-regressive (T-R) stratigraphic cycle (sensu Duval, Cramez & Vail, Reference Duval, Cramez, Vail and de Graciansky1998), i.e. defined on the basis of long-term displacements of the shoreline and controlled by a second-order eustatic cycle (Chatalov, Reference Chatalov2013). Marine deposition started during the late Early Triassic epoch when a carbonate ramp was progressively formed having gentle inclination to the east/southeast (Čatalov, Reference Čatalov1988). The ramp system evolved in the northwestern shelf area of the Tethys Ocean on the passive continental margin of Eurasia (see Chatalov, Reference Chatalov2013, and cited references), and palaeomagnetic data obtained by Muttoni et al. (Reference Muttoni, Gaetani, Budurov, Zagorchev, Trifonova, Ivanova, Petrounova and Lowrie2000) indicate palaeolatitude position of 21–24° N (Fig. 2). Diverse subtidal, intertidal and supratidal carbonate facies were generated without significant terrigenous input in several depositional environments under different hydrodynamic regimes (Fig. 3): tidal flats, inner ramp lagoons and shoals, storm-dominated and fair-weather mid-ramp areas and storm-influenced outer ramp (Assereto & Chatalov, Reference Asseretto and Chatalov1983; Tronkov, Reference Tronkov1983; Vaptsarova, Chemberski & Chatalov, Reference Vaptsarova, Chemberski and Chatalov1984; Chatalov, Reference Chatalov, Bachmann and Lerche2000 b, Reference Chatalov2002, Reference Chatalov2010, Reference Chatalov2013; Chatalov & Vangelov, Reference Chatalov and Vangelov2001). The sedimentation produced a nearly 500 m thick succession (Chatalov, Reference Chatalov2013), lithostratigraphically referred to as Iskar Carbonate Group (Tronkov, Reference Tronkov1981), which consists of limestones and dolostones (Fig. 4) plus a minor amount of siliciclastic rocks in the lowermost part (Chatalov, Stefanov & Vetseva, Reference Chatalov, Stefanov and Vetseva2015). Biostratigraphic analysis of carbonate strata outcropping in the western part of the External Balkanides, i.e. Western Balkanides, is mainly based on macrofauna (bivalves, brachiopods, rare ammonoids) and defines a chronostratigraphic range from the upper Olenekian substage to the lower Carnian substage (Tronkov, Reference Tronkov1968 a, Reference Tronkov1969, Reference Tronkov1973, Reference Tronkov1976; Benatov et al. Reference Benatov, Budurov, Trifonova and Petrunova1999; see also Chatalov, Reference Chatalov2013, and cited references).

Figure 1. Exposures of Lower to Upper Triassic marine rocks (Iskar Carbonate Group) in the Western Balkanides (yellow in the inset map) with designated location and number of the studied stratigraphic sections. Arrows indicate the advancing Tethyan transgression towards the end of the Olenekian age and at the onset of the Anisian age (see also Fig. 6).
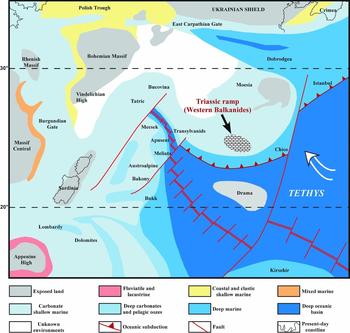
Figure 2. Palaeogeographical map of the Western Tethys in the early Ladinian age (from Gaetani et al. 2000) with inferred location of the Bulgarian carbonate ramp according to the palaeomagnetic data of Muttoni et al. (Reference Muttoni, Gaetani, Budurov, Zagorchev, Trifonova, Ivanova, Petrounova and Lowrie2000). Large arrow indicates the direction of subtropical storms based on Marsaglia & Klein (Reference Marsaglia and Klein1983).

Figure 3. Stratigraphic–lithologic log of the Iskar Carbonate Group (section 17 in Fig. 1) juxtaposed to the ramp stage or setting and the relative sea-level curve (modified from Chatalov, Reference Chatalov2013). Abbreviations: TB – Terebratula Beds, mfz – maximum flooding zone, RDT – retrogradational depositional trend, PDT – progradational depositional trend. Note: Thicknesses of the pre-ramp and post-ramp units are not to scale.

Figure 4. Panoramic view of the Triassic carbonate succession in the southern part of the study area (section 17). Arrows outline two genetic units in terms of sequence stratigraphy (T – transgressive, R – regressive) that correspond to the main stages of changing relative palaeobathymetry, i.e. retrogradational depositional trend and progradational depositional trend, respectively (Chatalov, Reference Chatalov2013). Bar indicates the maximum flooding zone (mfz), i.e. outer ramp Terebratula Beds.
In a recent synopsis Chatalov (Reference Chatalov2013) discussed the general characteristics and specific evolution of the carbonate ramp system. Two main stages of ramp development were distinguished on the basis of changing relative palaeobathymetry: deepening, i.e. retrogradational depositional trend, and shallowing, i.e. progradational depositional trend (Fig. 3). Sedimentation during those stages produced two genetic units (transgressive and regressive) in the carbonate succession separated by a maximum flooding zone, so-called Terebratula Beds (Fig. 4). The character of deep water sediments indicates lack of a significant break in slope angle, and hence a close correspondence to the homoclinal ramp model. The calculated average sedimentation rate is similar to other Phanerozoic shallow marine carbonates including those deposited on ramps. The inferred environmental constraints of the ramp depositional system include: normal marine to hypersalinity; warm, well-illuminated water; aerobic and rarely dysaerobic bottom conditions; dominantly soft lime mud substrate; intensive storm activity; temporal seismic influence. The vertical distribution of marine fauna indicates irreversible shifting from Germanic (Peri-Tethyan) affinity to Alpine (Tethyan) affinity during the Anisian age. The carbonate sedimentation was not accompanied by development of microbial build-ups or metazoan reefs.
As regards overall sediment production, the ramp system resembles some Early to Late Triassic carbonate platforms that existed across the Western Tethys realm. Thus, deposition was characterized by intensive formation of peloids and ooids, abundant lime mud production, growth of microbial fabrics, and development of specific biota dominated by mollusks, echinoderms, brachiopods, foraminifers and cyanobacteria (Chatalov, Reference Chatalov2013). The best correlation in terms of ramp type, general evolution, depositional settings, environmental parameters, facies distribution and biota diversity is established with respect to the Triassic ramp succession outcropping in the Tizsa Meagunit of southern Hungary (Török, Reference Török, Wright and Burchette1998, Reference Török, Bachmann and Lerche2000). Strong similarities to the German, Polish and Spanish Muschelkalk are also clearly outlined, and a parallel can be drawn with other platform carbonates from the NW Tethys shelf area and Peri-Tethys basins, the so-called Alpine realm and Germanic realm, respectively (see Chatalov, Reference Chatalov2013, and cited references).
3. General and specific controls on carbonate ramp sedimentation
The aforementioned general controls on the development of marine carbonate systems apply to the deposition on carbonate ramps (Burchette & Wright, Reference Burchette and Wright1992). Allogenic factors, antecedent topography and some environmental conditions control changes in the accommodation space and accumulation rates as well as the spatial and temporal distribution of ramp facies. Intrinsic processes, i.e. inherent to the depositional system, are related to the biotic and abiotic modes of carbonate production and influence the ramp geometry, sedimentation rate and facies diversity. Like all marine carbonate systems (e.g. Léonide, Floquet & Villier, Reference Léonide, Floquet and Villier2007), ramps are very sensitive to the magnitude and frequency of all controlling factors but their respective responses remain problematic, i.e. the precise differentiation of global, regional and local processes is often uncertain. Moreover, many factors are interdependent and superimposed on each other so that their relative importance and interplay are difficult to estimate in the dynamic carbonate ramp system (Wright & Burchette, Reference Wright, Burchette, Wright and Burchette1998). It has also been demonstrated that sedimentation in the shallow ramp, i.e. inner ramp environments, and in the deep ramp, i.e. mid-ramp to outer ramp environments, may have different sets of controlling mechanisms (Kim et al. Reference Kim, Rhee, Woo and Park2014).
The combined effect of global eustasy (tectono-eustatism or glacio-eustatism) and subsidence (basin-scale or local) is crucial in generating accommodation space for the ramp formation. Thus, rapid base-level rises controlled by eustasy and/or subsidence may lead to drowning, while different magnitudes of sea-level fall may change the ramp profile, subaerially expose the whole ramp or make the ramp evolve into rimmed shelf. Due to the low depositional relief, relative sea-level changes result in abrupt facies shifts between the inner ramp and outer ramp to basinal facies (Burchette & Wright, Reference Burchette and Wright1992), and in particular, homoclinal ramps have a linear response (migration of facies belts) to long-term sea-level oscillations (Tucker, Calvet & Hunt, Reference Tucker, Calvet, Hunt and Posamentier1993). According to Williams et al. (Reference Williams, Burgess, Wright, Della Porta and Granjeon2011), high-amplitude glacio-eustatic changes tend to create low-gradient ramp systems by distributing sediment accumulation across the whole width of the platform, thereby suppressing progradation and steepening. Both climate and sea-level changes control the accommodation space, slope gradient, water depth and circulation, facies distribution, stacking patterns, and formation of cyclic successions. Tectonic regime alone may favour the accumulation of thick ramp deposits, with optimal ramp development being promoted by slow subsidence rate, low gradient basement and relatively shallow basinal water depth (Read, Reference Read1985; Burchette & Wright, Reference Burchette and Wright1992; Bosence, Reference Bosence2005). Rotational subsidence tends to favour ramp formation by increasing the topographic gradient, which leads to enhanced rates of sediment transport and suppressed in situ accumulation (Williams et al. Reference Williams, Burgess, Wright, Della Porta and Granjeon2011). Likewise, tectonics may ultimately create distally-steepened ramp morphology through differential subsidence associated with synsedimentary active faults (Read, Reference Read1985; Burchette & Wright, Reference Burchette and Wright1992). Antecedent topography inherited from the preceding depositional or tectonic regime may favour the formation of low-gradient relief, and hence the configuration and size of carbonate ramps (Burchette & Wright, Reference Burchette and Wright1992), but may also predetermine the initialization of distal steepening (e.g. Seyedmehdi, George & Tucker, Reference Seyedmehdi, George and Tucker2016).
Ramp morphology is largely controlled by the rate of sediment input and hydrodynamic material dispersal along the bathymetric gradient, commonly with strong offshore sediment transport (Aurell et al. Reference Aurell, Bádenas, Bosence, Waltham, Wright and Burchette1998; Pomar, Reference Pomar2001 b; Bádenas et al. Reference Bádenas, Aurell, Rodríguez-Tovar and Pardo-Igúzquiza2003; Beavington-Penney, Wright & Racey, Reference Beavington-Penney, Wright and Racey2005; Wright & Burgess, Reference Wright and Burgess2005; Williams et al. Reference Williams, Burgess, Wright, Della Porta and Granjeon2011; Puga-Bernabéu et al. Reference Puga-Bernabéu, Martín, Braga and Aguirre2014). Carbonate productivity, locus of accumulation and sedimentation rate may change the ramp profile, for example, by favouring the initialization of distal steepening (Burchette & Wright, Reference Burchette and Wright1992; Pedley, Reference Pedley, Wright and Burchette1998; Pomar, Reference Pomar2001 b; Pomar et al. Reference Pomar, Bassant, Brandano, Ruchonnet and Janson2012), or the evolution into another type of carbonate platform which is mostly rimmed shelf (Read, Reference Read1985). Because ramps are relatively low-productivity systems, decreased carbonate production may lead to drowning (Burchette & Wright, Reference Burchette and Wright1992). Ramp geometries result from a relatively homogeneous accumulation rate along dip (Burchette & Wright, Reference Burchette and Wright1992; Read, Reference Read, Wright and Burchette1998; Pomar, Reference Pomar2001 a, b; Williams et al. Reference Williams, Burgess, Wright, Della Porta and Granjeon2011) which is due to decreased differentiation of depth-dependent production (Koerschner & Read, Reference Koerschner and Read1989; Wright & Faulkner, Reference Wright and Faulkner1990; Burchette & Wright, Reference Burchette and Wright1992), or depth-enhanced carbonate production (Pomar, Reference Pomar2001 a, b; Brandano & Corda, Reference Brandano and Corda2002; Pomar, Brandano & Westphal, Reference Pomar, Brandano and Westphal2004). Minimum capacity in infilling the shallow-water space, i.e. for building above the hydrodynamic equilibrium profile (ecological accommodation), is shown by homoclinal ramps, while physical accommodation prevails in distally steepened ramps (Pomar, Reference Pomar2001 a, b; Pomar & Kendall, Reference Pomar, Kendall, Lukasik and Simo2008). Palaeogeographical position and oceanographic regime (tides, storms, wave energy and oceanic currents) can be important for sediment transport by shaping sediment bodies and/or controlling resedimentation dynamics. Carbonate systems with high rates of offshore transport and relatively low production rates tend to drape the underlying topography and thus maintain low-gradient ramp profiles preventing formation of a steep platform margin (Williams et al. Reference Williams, Burgess, Wright, Della Porta and Granjeon2011).
Last but not least, the absence of metazoan and other framework-builders capable of building steep platform margins may favour the configuration and maintenance of ramp morphology (e.g. Wright & Faulkner, Reference Wright and Faulkner1990; Burchette & Wright, Reference Burchette and Wright1992; Hips, Reference Hips, Wright and Burchette1998; Török, Reference Török, Wright and Burchette1998; Beavington-Penney, Wright & Racey, Reference Beavington-Penney, Wright and Racey2005; Bakhtiar, Taheri & Vaziri-Moghaddam, Reference Bakhtiar, Taheri and Vaziri-Moghaddam2011; Shao et al. Reference Shao, Wang, Cai, Wang, Lu and Zhang2011; Wilson et al. Reference Wilson, Chambers, Manning and Nas2012; Kietzmann et al. Reference Kietzmann, Palma, Riccardi, Martín-Chivelet and López-Gómez2014). Therefore, the development of carbonate ramps may be crucially influenced by biological evolution related to mass extinction, biota recovery and diversification. Such dependence was particularly well demonstrated during some time intervals of the Phanerozoic with predominant ramp development (Burchette & Wright, Reference Burchette and Wright1992).
In historical perspective, various aspects of coupled allogenic and autogenic controls on the carbonate ramp sedimentation were discussed in numerous case studies (e.g. Markello & Read, Reference Markello and Read1981; Aigner, Reference Aigner1985; Gawthorpe, Reference Gawthorpe1986; Calvet, Tucker & Helton, Reference Calvet, Tucker, Helton and Tucker1990; Wright & Faulkner, Reference Wright and Faulkner1990; Rüffer, Reference Rüffer1995; Elrick, Reference Elrick1996; Pedley, Reference Pedley, Wright and Burchette1998; Rosales, Reference Rosales1999; Pomar, Reference Pomar2001 a; Brandano & Corda, Reference Brandano and Corda2002; Bádenas et al. Reference Bádenas, Aurell, Rodríguez-Tovar and Pardo-Igúzquiza2003), and some recent researches have greatly improved our knowledge about Phanerozoic examples of different ages (Martin-Rojas et al. Reference Martin-Rojas, Somma, Delgado, Estévez, Iannace and Zamparelli2012; Pomar et al. Reference Pomar, Bassant, Brandano, Ruchonnet and Janson2012; Chow et al. Reference Chow, George, Trinajstic and Chen2013; Laya, Tucker & Perez-Huerta, Reference Laya, Tucker and Perez-Huerta2013; Brigaud et al. Reference Brigaud, Vincent, Carpentier, Robin, Guillocheau, Yven and Huret2014; Kim et al. Reference Kim, Rhee, Woo and Park2014; Puga-Bernabéu et al. Reference Puga-Bernabéu, Martín, Braga and Aguirre2014). The most systematic and comprehensive reviews of the controlling factors on Triassic ramp systems from the Western Tethys realm were presented by Hips (Reference Hips, Wright and Burchette1998) and Török (Reference Török, Wright and Burchette1998).
4. Controls on the Triassic carbonate ramp system
4.a. Global and regional controls
The Triassic carbonate ramp of the Western Balkanides evolved under the influence of several controlling factors of global or regional significance: geotectonic setting, antecedent topography, climate and oceanographic regime, relative sea-level changes, lack of frame-builders, and carbonate production (Fig. 5). Their interplay favoured the onset of marine carbonate sedimentation, the configuration of a homoclinal ramp, long-term maintenance of the ramp morphology, and final demise of the carbonate depositional system.
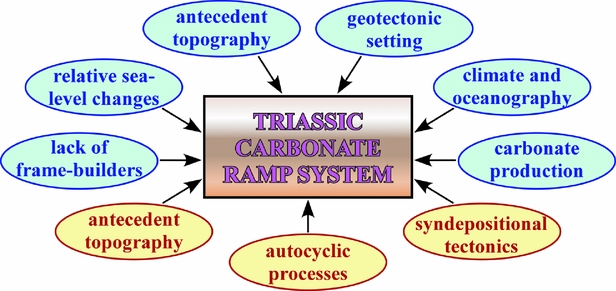
Figure 5. Summary diagram of controlling factors on the development of the Triassic ramp depositional system (global and regional controls in blue-filled ovals, local controls in yellow-filled ovals).
4.a.1. Geotectonic setting
A broad belt of carbonate platforms developed across the NW Tethys shelf area during the Triassic period (Marcoux et al. Reference Marcoux, Dercourt, Ricou and Vrielynck1993; Michalík, Reference Michalík1994; Haas et al. Reference Haas, Kovacs, Krystyn and Lein1995; Gaetani et al. Reference Gaetani and Dercourt2000a ; Golonka, Reference Golonka2007). The widespread formation of carbonate ramps has been proved on the basis of well-investigated outcrops in the Northern Calcareous Alps (Rüffer, Reference Rüffer1995; Rüffer & Zamparelli, Reference Rüffer and Zamparelli1997), the Southern Alps (Senowbari-Daryan et al. Reference Senowbari-Daryan, Zühlke, Bechstädt and Flügel1993; Zühlke, Reference Zühlke2000), the Western Carpathians (Michalík et al. Reference Michalík, Masaryk, Lintnerová, Papšová, Jendrejáková and Reháková1992; Rychliński & Szulc, Reference Rychliński and Szulc2005), several regions of Hungary (Haas & Budai, Reference Haas and Budai1995; Hips, Reference Hips, Wright and Burchette1998; Török, Reference Török, Wright and Burchette1998), and other countries in Europe (see summary by Kovács et al. Reference Kovács2011). Tectonic regime of passive continental margin has been suggested for some areas and time intervals with ramp sedimentation (Rüffer & Zamparelli, Reference Rüffer and Zamparelli1997; Török, Reference Török, Wright and Burchette1998; Mandl, Reference Mandl2000; Kovács et al. Reference Kovács2011; Haas, Budai & Raucsik, Reference Haas, Budai and Raucsik2012).
During most of the Triassic period Bulgaria was part of the Tethyan passive continental margin of Eurasia (see Chatalov, Reference Chatalov2013, and cited references). The regional tectonic regime strongly favoured the development of a gently sloping ramp environment (Read, Reference Read1982; Burchette & Wright, Reference Burchette and Wright1992; Bosence, Reference Bosence2005) because passive margin basins are considered to be the major site of carbonate platform sedimentation (Read, Reference Read1982; Tucker & Wright, Reference Tucker and Wright1990, p. 38; Einsele, Reference Einsele2000, p. 138). Passive continental margins facilitate ramp formation by having slow, relatively uniform subsidence rates, low-relief initial bathymetry for the carbonate to drape, and usually minimal influence of regional tectonics such as lack of large-scale fault influence (Williams et al. Reference Williams, Burgess, Wright, Della Porta and Granjeon2011). The absence of significant siliciclastic input from the low-relief hinterland during the early development of the passive margin further promotes widespread carbonate deposition (Burchette & Wright, Reference Burchette and Wright1992; Bosence, Reference Bosence2005). The particular geotectonic setting can also explain the relatively great thickness of accumulated Triassic carbonates (~500 m) because vertical accretion characterizes ramps formed on passive margins (Burchette & Wright, Reference Burchette and Wright1992). Moreover, the calculated average sedimentation rate for the ramp succession (Chatalov, Reference Chatalov2013) is in conformity with the passive margin setting, showing that the carbonate sedimentation was able to keep up with the subsidence rate in order to maintain the ramp morphology. It is noteworthy that some tectonic activity related to an Early Triassic embryonic rifting in this part of the Western Tethys (see Chatalov, Reference Chatalov2013, and cited references) probably conduced to the west/northwestward advancing transgression (Chemberski et al. Reference Chemberski, Rankova, Antova and Nikolov1996) along with the ramp initialization. Similarly, westward propagation of the Tethys rift system provided avenues for Triassic transgressions of the Tethys Sea onto Europe (Ziegler, Reference Ziegler1988; Ziegler & Stampfli, Reference Ziegler and Stampfli2001).
The regional influence of tectonics as a major control on the Late Triassic demise of the Bulgarian carbonate ramp is controversial. According to Zagorchev & Budurov (Reference Zagorchev and Budurov2007), intensive tectonic activity during the early Carnian subage promoted breakdown of the carbonate platform as a result of differential uplift. The latter was related to the early Cimmerian geodynamics, the so-called Eo-Cimmerian orogenic event, which occurred in adjacent Tethyan domains coevally with the final closure of the Paleotethys Ocean (Şengor, Yılmaz & Sungurlu, Reference Şengor, Yılmaz, Sungurlu, Dixon and Robertson1984). However, the timing of recognized early Cimmerian structures in the Western Balkanides has been unambiguously proved to postdate the marine carbonate deposition, being concomitant with the subsequent accumulation of continental red-bed siliciclastics during the Late Triassic (Tronkov, Reference Tronkov1963). Therefore, while the hypothesis for some tectonic control on the early Carnian ramp demise cannot be ruled out (see Hornung & Brandner, Reference Hornung and Brandner2005; Hornung et al. Reference Hornung, Brandner, Krystyn, Joachimski, Keim, Lucas and Spielman2007; Hornung, Krystyn & Brandner, Reference Hornung, Krystyn and Brandner2007; Martin-Rojas et al. Reference Martin-Rojas, Somma, Delgado, Estévez, Iannace and Zamparelli2012), there is evidence that another extrinsic factor was more important for the termination of marine carbonate sedimentation (Section 4.a.4 below).
4.a.2. Antecedent topography
Towards the close of the Early Triassic epoch a low-gradient topography developed across the study area as a result of prolonged continental and subsequent marginal-marine sedimentation (Tronkov, Reference Tronkov1966; Mader & Čatalov, Reference Mader, Čatalov and Mader1992 a; Chemberski et al. Reference Chemberski, Rankova, Antova and Nikolov1996; Zagorchev & Dabovski, Reference Zagorchev, Dabovski and Zagorchev2009). The general fining-upward trend in the Lower Triassic strata of the Petrohan Terrigenous Group and the overlying Svidol Formation (Fig. 3) reflects extensive formation of alluvial braidplain and floodplain systems (Mader & Čatalov, Reference Mader, Čatalov and Mader1992 a) and later transition to a coastal marine sandy–muddy flat (Chatalov, Stefanov & Vetseva, Reference Chatalov, Stefanov and Vetseva2015), thus implying advanced peneplanation of the palaeorelief. Such a pattern of antecedent topography is also inferred from the vast area of fluvial-dominated siliciclastic deposition during the Early Triassic epoch as is illustrated by proposed palaeogeographical reconstructions for Bulgaria (Vaptsarova, Chemberski & Chatalov, Reference Vaptsarova, Chemberski and Chatalov1984; Chemberski et al. Reference Chemberski, Rankova, Antova and Nikolov1996; Zagorchev & Budurov, Reference Zagorchev and Budurov1997) and Europe (Gaetani et al. Reference Gaetani and Dercourt2000b ). Other evidence comes from the widespread development of tidal flat environment across the ramp at the time of its initialization, i.e. Spathian subage, which is compatible with the absence of significant topographic relief (cf. Sumner & Beukes, Reference Sumner and Beukes2006).
The flat basement inherited from the preceding depositional regime was an important regional control on the configuration of extensive ramp setting by allowing the accumulated carbonates to drape the low-gradient antecedent relief. Therefore, the gentle palaeotopography combined with the quiescent tectonic regime was a major prerequisite that favoured the formation of homoclinal ramp morphology. Because the upper Olenekian to middle Anisian peritidal succession (Opletnya Mb of Mogilata Fm) deposited during the early stage of ramp retrogradation has maximum and persistent thickness in the southern part of the Western Balkanides (Figs 1, 3), it can be presumed that greatly variable topographic gradients (highs and/or depressions) did not influence the gross carbonate stratal geometry. However, in the rest exposures to the northwest the same peritidal deposits display progressively smaller total thicknesses (Fig. 6) and fewer peritidal cycles (see also Section 4.b.2 below). Furthermore, the basal marine strata in the northwesternmost part of the study area have early Anisian age (Tronkov, Reference Tronkov1973, Reference Tronkov1976; Muttoni et al. Reference Muttoni, Gaetani, Budurov, Zagorchev, Trifonova, Ivanova, Petrounova and Lowrie2000). These facts can be explained with a Tethyan transgression advancing from the east/southeast direction (Section 4.a.4 below) towards the end of the Olenekian age and at the beginning of the Anisian age.

Figure 6. Correlation of selected stratigraphic–lithologic logs along a NW–SE transect across the Western Balkanides. The decreasing total thickness of the upper Olenekian – lower Anisian peritidal succession (Mogilata Fm) in NW direction and the early Anisian age of the basal marine strata (Edivetar Fm) in the Belogradchik region are explained by Tethyan transgression advancing from the east/southeast (see also Fig. 1). Some characteristics of the pre-ramp basement and overlying subtidal deposits in sections 1 and 2 suggest local control of the antecedent topography at the time of ramp initialization. Note: thicknesses of the Petrohan Terrigenous Group are not to scale.
4.a.3. Climate and oceanographic regime
The onset of the Mesozoic era was marked by extreme ‘hothouse’ conditions that existed during the Early Triassic epoch while mainly greenhouse conditions persisted until the Middle Jurassic epoch (Holz, Reference Holz2015). The Bulgarian carbonate ramp was located in the subtropical belt (20–30° N) of the NW Tethys, and semi-arid to arid climate prevailed in those palaeolatitudes during most of the Triassic period (Sellwood & Valdes, Reference Sellwood and Valdes2006; Feist-Burkhardt et al. Reference Feist-Burkhardt and McCann2008; Preto, Kustatscher & Wignall, Reference Preto, Kustatscher and Wignall2010; Franz et al. Reference Franz, Kaiser, Fischer, Heunisch, Kustatscher, Luppold, Berner and Röhling2015). Triassic case studies from the Western Tethys realm based on different proxies also attest to such a climatic regime which was presumably interrupted by short humid pulses (e.g. Hips, Reference Hips, Wright and Burchette1998; Török, Reference Török, Wright and Burchette1998; Rychliński & Szulc, Reference Rychliński and Szulc2005; Stefani, Furin & Gianolla, Reference Stefani, Furin and Gianolla2010; Haas, Budai & Raucsik, Reference Haas, Budai and Raucsik2012).
Sedimentological analysis of the Triassic marine succession in the Western Balkanides unequivocally indicates warm, dry climatic conditions during the ramp development. The most important evidence includes the presence of penecontemporaneous dolomites with desiccation cracks, tepee structures, flat-pebble layers, fenestral fabrics, spar-filled former sulphate nodules and pseudomorphs after evaporites (Figs 7e, 12a, b). Moreover, the low siliciclastic input accompanying the carbonate deposition (see lithology in Figs 3, 4) implies pronounced aridity of the hinterland. Other textural and compositional characteristics of the rocks (Fig. 10, further below) are typical for warm-water tropical carbonates deposited in the non-equatorial (see Wilson, Reference Wilson2012) tropics: fossil assemblage with elements of the Photozoan asociation, e.g. green algae (Fig. 7a) and rare scleractinian corals; cyanobacteria (Fig. 7b) and microbial laminites (Fig. 12b); non-skeletal grain association dominated by ooids and well-preserved peloids (Fig. 7c, i) plus other coated grains (oncoids, cortoids) and various types of aggregate grains (Fig. 7d, f); abundant lime mud and marine phreatic cements (Fig. 7g); aragonite and high-Mg calcite primary mineralogies of abiotic carbonates (Fig. 7h–j). The overall warm and dry climatic regime favoured the shallow water carbonate sedimentation and this relationship is well illustrated by the global latitudinal distribution of carbonate platforms in Triassic times (Kiessling, Flügel & Golonka, Reference Kiessling, Flügel and Golonka2003; Philip, Reference Philip2003; Golonka, Reference Golonka2007).

Figure 7. Compositional and textural rock characteristics attesting to deposition in the non-equatorial tropics under warm, dry climate. (a) Dasycladacean alga (i.e. Photozoan association). Toshkovdol Fm, section 4. (b) Calcified Girvanella filaments forming hemispheroid of microstromatolite. Mogilata Fm, section 17. (c) Well-preserved peloids in peloidal packstone. Toshkovdol Fm, section 2. (d) Aggregate grain (lump) consisting of peloids and micritized ooids. Toshkovdol Fm, section 4. (e) Dolomite pseudomorphs after rosettes of calcium sulphate in peritidal dolomudstone. Mogilata Fm, section 17. (f) Large grapestone intraclast representing cluster of bound peloids. Toshkovdol Fm, section 4. (g) Acicular marine cement filling the whole pore space in ooidal grainstone. Growth competition between cement fringes on adjacent ooids produced polygonal sutures and hourglass forms (arrows). Toshkovdol Fm, section 1. (h) Originally aragonitic ooids with locally developed brickwork fabrics (arrow). Mogilata Fm, section 19. (i) Ooidal–peloidal grainstone showing different ooid primary mineralogies. The radial ooids were originally calcitic (C) while the shrunken ooids were both aragonitic (A) and bimineralic (B). Toshkovdol Fm, section 2. (j) Bimineralic ooid with inner brickwork layers (originally aragonitic) and outer micritic/microsparitic layers (originally calcitic). Mogilata Fm, section 16. Scale bar in all microphotographs is 0.3 mm across. Note: the Toshkovdol Fm from sections 1, 2 and 4 is a non-dolomitized chronostratigraphic and facies equivalent of the Milanovo Fm (see Fig. 10).
The windward palaeoceanographic position of the ramp was favourable for the influence of subtropical storms (Fig. 2) which considerably affected the style of carbonate deposition (Chatalov & Vangelov, Reference Chatalov and Vangelov2001; Chatalov, Reference Chatalov2010, Reference Chatalov2013). The recognized Pelsonian proximal and distal tempestites (Fig. 10, further below) were formerly interpreted as products of common winter storms and occasional hurricanes on the basis of the assumed palaeogeographic position in the belt of 25−45° N (Chatalov & Vangelov, Reference Chatalov and Vangelov2001), i.e. according to the palaeolatitudinal zonation of Marsaglia & Klein (Reference Marsaglia and Klein1983). However, the palaeomagnetic data published by Muttoni et al. (Reference Muttoni, Gaetani, Budurov, Zagorchev, Trifonova, Ivanova, Petrounova and Lowrie2000) indicate a position in the belt of 5–25° N, and therefore only occasional winter storms (one or two per year) and hurricanes (once in 3000 years) can be predicted (Marsaglia & Klein, Reference Marsaglia and Klein1983). The oceanographic regime of storm influence was important for sediment redistribution across the ramp by favouring offshore transport towards deep ramp areas through storm-generated bottom currents. This conclusion is based on the following observations (cf. Aurell et al. Reference Aurell, Bádenas, Bosence, Waltham, Wright and Burchette1998; Török, Reference Török, Wright and Burchette1998; Pérez-López & Pérez-Valera, Reference Pérez-López and Pérez-Valera2012; Kietzmann et al. Reference Kietzmann, Palma, Riccardi, Martín-Chivelet and López-Gómez2014): (1) large volume of storm deposits and particularly thin-bedded distal tempestites (Fig. 8a, b), (2) coarse-grained character of the storm layers compared to the associated fair-weather lithofacies, i.e. calcimudstones and bioclastic wackestones, (3) presence of shallow water grains such as ooids, ooidal intraclasts (Fig. 8c) and reworked oncoids (Fig. 8d) in the proximal tempestites, (4) variable amount of resedimented bioclasts from shallow to deep ramp areas, for example, reworked crinoid ossicles (see Chatalov, Reference Chatalov2013) or gastropod shells with darker micrite infill than the surrounding matrix (Fig. 8e), and (5) presence of terrigenous quartz silt and mica flakes in the distal tempestites (Fig. 8f). The inferred increased rates of offshore transport with probably significant amounts of lime mud promoted maintenance of a low-gradient ramp profile by draping the flat palaeotopography and preventing the basin-scale development of steep platform margin. The interpretation of substantial storm influence is in conformity with numerous studies on Triassic ramp carbonates from other localities in the Western Tethys shelf area and the Peri-Tethyan Germanic Basin (Aigner, Reference Aigner1985; Michalík et al. Reference Michalík, Masaryk, Lintnerová, Papšová, Jendrejáková and Reháková1992; Hips, Reference Hips, Wright and Burchette1998; Török, Reference Török, Wright and Burchette1998; Rychliński & Szulc, Reference Rychliński and Szulc2005; Knaust & Costamagna, Reference Knaust and Costamagna2012; Pérez-López & Pérez-Valera, Reference Pérez-López and Pérez-Valera2012).

Figure 8. Anisian storm deposits in the Zimevitsa Mb of Babina Fm. (a) Thick bed of proximal tempestite having ‘matrix’ of monomict pebbles derived from fair-weather calcimudstones and containing outsized clasts (arrows) cannibalized from another tempestite. Outcrop between sections 16 and 17. Scale: hammer is 33 cm long. (b) Thin-bedded alternation of fair-weather nodular calcimudstones and coarse-grained distal tempestites (T) with locally developed gutter casts (arrows). Section 17. Scale: marker pen (encircled) is 13.5 cm long. (c) Redeposited radial ooids and ooidal intraclasts in proximal tempestite (sh – spar-filled shelter pore). Section 17. (d) Reworked oncoid in proximal tempestite. Section 16. (e) Gastropod shells with darker micrite infill than the surrounding matrix in proximal tempestite. Section 7. (f) Silt-sized quartz grains (green arrows) and mica flakes (red arrows) in distal tempestite. Section 17. Scale bar in all microphotographs is 0.3 mm across.
The uppermost part of the ramp succession consists of lower Carnian peritidal strata (Rusinovdel Fm) which are covered by Upper Triassic continental red beds (Moesian Group) (Fig. 3). The abrupt lithological and facies contact between the two units (Fig. 9a) implies that the Late Triassic demise of the shallow ramp system may have been caused by intensive siliciclastic input. Temporary shutdown or termination of carbonate platforms across the Western Tethys shelf occurred in the late Julian subage along with reduced carbonate productivity, increased nutrient supply, reef crisis, biotic turnover, freshwater influx and enhanced siliciclastic deposition (Simms, Ruffell & Johnson, Reference Simms, Ruffell, Johnson, Fraser and Sues1995; Hornung & Brandner, Reference Hornung and Brandner2005; Keim, Spötl & Brandner, Reference Keim, Spötl and Brandner2006; Sýkora, Siblík & Soták, Reference Sýkora, Siblík and Soták2011; Escudero-Mozo et al. Reference Escudero-Mozo, Martín-Chivelet, Goy and López-Gómez2014). The latter was also recorded in adjacent marginal marine, deep marine and continental environments (Rigo et al. Reference Rigo, Preto, Roghi, Tateo and Mietto2007; Kozur & Bachmann, Reference Kozur and Bachmann2010; Roghi et al. Reference Roghi, Gianolla, Minarelli, Pilati and Preto2010; Haas, Budai & Raucsik, Reference Haas, Budai and Raucsik2012) and was controlled by a humid climate perturbation known as the ‘Carnian Pluvial Episode’ or under other names (Ruffell, Simms & Wignall, Reference Ruffell, Simms and Wignall2015, and references therein). The middle Carnian decline or demise of marine carbonate systems has been commonly explained by enhanced weathering, development of large fluvial systems and significant siliciclastic input which suffocated the carbonate factory (Hornung & Brandner, Reference Hornung and Brandner2005; Hornung, Krystyn & Brandner, Reference Hornung, Krystyn and Brandner2007; Gattolin et al. Reference Gattolin, Preto, Breda, Franceschi, Isotton and Gianolla2015) and led to the so-called ‘Carnian Crisis’ (Hornung et al. Reference Hornung, Brandner, Krystyn, Joachimski, Keim, Lucas and Spielman2007). While some researchers claim that the Carnian Pluvial Episode had only regional character, there is growing evidence for its global nature that affected vast areas of the northern hemisphere spanning from tropical to high latitudes (e.g. Hornung, Krystyn & Brandner, Reference Hornung, Krystyn and Brandner2007; Arche & López-Gómez, Reference Arche and López-Gómez2014; Nakada et al. Reference Nakada, Ogawa, Suzuki, Takahashi and Takahashi2014; Ruffell, Simms & Wignall, Reference Ruffell, Simms and Wignall2015). Consequently, fundamental impact of the middle Carnian humid climate phase/episode on the demise of the Bulgarian carbonate ramp is a possible scenario considering its Tethyan-wide manifestation and effects. Moreover, the base of the Moesian Group in most outcrops comprises quartz arenites (Fig. 9a) having thickness up to several metres, and this compositional type of sandstones may record extreme conditions of formation including warm and humid climate (Suttner, Basu & Mack, Reference Suttner, Basu and Mack1981; Avigad et al. Reference Avigad, Sandler, Kolodner, Stern, McWilliams, Miller and Beyth2005; Van de Kamp, Reference Van de Kamp2010; Garzanti et al. Reference Garzanti, Padoan, Andò, Resentini, Vezzoli and Lustrino2013). Preliminary micropetrographic observations (Chatalov, unpub. data) have shown that these rocks greatly resemble first-cycle quartz arenites (i.e. with high compositional but low textural maturity), and hence, their formation may have been related to increased humidity, i.e. the Carnian Pluvial Episode. Nevertheless, other sedimentological evidence reveals the prime importance of a different extrinsic control that caused termination of the marine carbonate sedimentation (Section 4.a.4 below).

Figure 9. Subaerial unconformity between lower Carnian marine strata (Iskar Carbonate Group) and Upper Triassic continental deposits (Moesian Group). (a) Karstified exposure surface developed on peritidal dolomudstones (dm) and covered by quartz arenites (qa). The latter have high compositional but low textural maturity (Chatalov, unpub. data) and their deposition was probably related to the effect of the ‘Carnian Pluvial Episode’. Section 7. (b) Paleodoline (palaeosinkhole) with siliciclastic infill (S) reflecting formation of epikarst morphology on the carbonate substrate (C) before the onset of continental sedimentation. Outcrop north of section 16.
4.a.4. Relative sea-level changes
The slow continuous subsidence, low-gradient antecedent topography and stable climatic conditions imply that relative sea-level changes were a major control on the evolution of the carbonate ramp system. The time of ramp initialization and subsequent deepening (Fig. 3) corresponds to the global Olenekian to Anisian sea-level rise (Haq, Hardenbol & Vail, Reference Haq, Hardenbol and Vail1987) and this eustatic signal is well documented across the Western Tethys realm where extensive shallow shelves were flooded (Gianolla & Jaquin, Reference Gianolla, Jaquin and de Graciansky1998; Gaetani et al. Reference Gaetani and Dercourt2000a ; Kiessling, Flügel & Golonka, Reference Kiessling, Flügel and Golonka2003; Golonka, Reference Golonka2007). The aforesaid transgression in the west/northwest direction (Fig. 1) is also manifested on a wider regional scale as the Tethys waters advanced from the east/southeast (Chemberski et al. Reference Chemberski, Rankova, Antova and Nikolov1996; Gaetani et al. Reference Gaetani and Dercourt2000a , b; Feist-Burkhardt et al. Reference Feist-Burkhardt and McCann2008; Bourquin et al. Reference Bourquin, Bercovici, López-Gómez, Díez, Broutin, Ronchi, Durand, Arche, Linol and Amour2011). Similarly to other Triassic ramps (e.g. Calvet, Tucker & Helton, Reference Calvet, Tucker, Helton and Tucker1990; Aigner & Bachmann, Reference Aigner and Bachmann1992; Hips, Reference Hips, Wright and Burchette1998; Török, Reference Török, Wright and Burchette1998; Rychliński & Szulc, Reference Rychliński and Szulc2005; Escudero-Mozo et al. Reference Escudero-Mozo, Martín-Chivelet, Goy and López-Gómez2014), the Bulgarian carbonate ramp was a low-relief system which deepened gradually as a response to long-term sea-level rise. From the Pelsonian subage onwards the regressive character of carbonate sedimentation, i.e. progradational depositional trend (Fig. 3), reflects a relative sea-level fall, and therefore the entire ramp succession suggests a close relationship to a second-order T-R eustatic cycle (cf. Aigner & Bachmann, Reference Aigner and Bachmann1992; Török, Reference Török, Wright and Burchette1998).
The above-mentioned abrupt lithological and facies contact between Upper Triassic peritidal dolostones and overlying continental siliciclastics represents a sharp discontinuity surface (Clari, Della Pierre & Martire, Reference Clari, Della Pierre and Martire1995; Hillgärtner, Reference Hillgärtner1998; Sattler et al. Reference Sattler, Immenhauser, Hillgärtner and Esteban2005). To be more precise, it is interpreted as an exposure surface showing in section view depressions with maximum amplitude of several decimetres, steep or gentle sides, and subplanar to uneven bases (Fig. 9a). In plan view, distinct palaeodolines (palaeosinkholes) having depths to about 1 m and siliciclastic infill are recognized in some outcrops (Fig. 9b). These dissolution features reflect formation of minor epikarst morphology on the carbonate substrate, and therefore, imply moderate subaerial exposure with development of near-surface karst processes (Esteban & Klappa, Reference Esteban, Klappa, Scholle, Bebout and Moore1983). The fine-grained parent material of the subaerially exposed peritidal deposits probably facilitated early consolidation while the low topographic relief of the exposure surface provided favourable conditions for karst formation (cf. Chow & Wendte, Reference Chow and Wendte2011). The explicit evidence for long-term regression since the Pelsonian subage and termination of the carbonate production in the Julian subage suggests that a relative sea-level fall caused subaerial emergence of the carbonate seafloor and consequent demise of the ramp. Moreover, the development of palaeokarst features indicates that the presumably climate-related initial continental deposition (Section 4.a.3 above) was not a direct result of progradation of siliciclastic systems under normal regressive conditions but postdated the shutdown of the carbonate factory after some period of subaerial exposure and non-deposition. The relatively poor development of karst morphology may have been controlled by short duration of the emergence and/or semi-arid climate conditions. A sea-level fall in the late Julian subage has been inferred from other studies of Western Tethys and Peri-Tethyan carbonate successions, for example, in the Northern Calcareous Alps (Mandl, Reference Mandl2000; Haas et al. Reference Haas, Piros, Budai, Görög, Mandl and Lobitzer2010), Dolomites (De Zanche et al. Reference De Zanche, Gianolla, Mietto, Siorpaes and Vail1993; Keim, Spötl & Brandner, Reference Keim, Spötl and Brandner2006), Iberia (Arche & López-Gómez, Reference Arche and López-Gómez2014), Balearic Islands (Escudero-Mozo et al. Reference Escudero-Mozo, Martín-Chivelet, Goy and López-Gómez2014), Balaton Highland (Nagy, Reference Nagy1999) and Central European Basin (Kozur & Bachmann, Reference Kozur and Bachmann2010). According to Arche & López-Gómez (Reference Arche and López-Gómez2014) the change from marine sedimentation during the early Carnian subage to continental sedimentation during the middle Carnian in many European basins can only be explained by a rapid sea-level drop coeval with the short-lived pluvial event. In Minorca Island, this sea-level fall resulted in emersion, karstification and erosion of a carbonate ramp, and its eustatic origin is further suggested by the widespread demise of carbonate systems across the Western Tethys and the Germanic Basin (Escudero-Mozo et al. Reference Escudero-Mozo, Martín-Chivelet, Goy and López-Gómez2014). A recent hypothesis by Berra (Reference Berra2012) postulated that global cooling in the early Carnian subage caused a shift of the poleward boundary of the arid belt toward the equator and triggered a sea-level fall by increased storage of fresh water in continental settings and change in seawater density.
The influence of relative sea-level changes on the formation of third-order sedimentary cycles in the Bulgarian carbonate ramp is debatable. For example, five mesocycles of ‘allogenic origin’, corresponding to the third-order cycles (0.5-3 Ma) of Vail et al. (Reference Vail, Audemard, Bowman, Eisner, Perez-Cruz, Einsele, Ricken and Seilacher1991) and to the ‘large-scale sequences’ of Strasser et al. (Reference Strasser, Pittet, Hillgärtner and Pasquier1999), have been distinguished in the Olenekian–Anisian peritidal succession, i.e. Opletnya Mb of Mogilata Fm (Fig. 3), by Ajdanlijski, Strasser & Tronkov (Reference Ajdanlijski, Strasser, Tronkov and Sinnyovsky2004). Each mesocycle consists of four submesocycles which in turn include four to five elementary cycles. According to Ajdanlijski, Strasser & Tronkov (Reference Ajdanlijski, Strasser, Tronkov and Sinnyovsky2004), the elementary cycles have different thicknesses and are dominated by different lithofacies (allochemic limestones, micritic limestones and dolostones) in the lower, middle and upper part of each mesocycle, respectively. In general, third-order sequences are depositional cycles bounded by exposure surfaces and consist of lowstand, transgressive and highstand systems tracts (Haq, Hardenbol & Vail, Reference Haq, Hardenbol and Vail1987; Vail et al. Reference Vail, Audemard, Bowman, Eisner, Perez-Cruz, Einsele, Ricken and Seilacher1991). While their origin has been related to both climatic and tectonic influences (Vail et al. Reference Vail, Audemard, Bowman, Eisner, Perez-Cruz, Einsele, Ricken and Seilacher1991; Strasser et al. Reference Strasser, Hillgärtner, Hug and Pittet2000), orbitally forced climate changes have recently been demonstrated to be the main control via glacioeustasy, thermoeustasy and/or other processes (Boulila et al. Reference Boulila, Galbrun, Miller, Pekar, Browning, Laskar and Wright2011). New field observations in the study area (Section 4.b.2 below) have shown that the individual thicknesses, intracycle lithofacies distribution and stacking patterns of the elementary cycles (sensu Ajdanlijski, Strasser & Tronkov, Reference Ajdanlijski, Strasser, Tronkov and Sinnyovsky2004) do not outline any particular trend upsection (Fig. 13, further below), and hence these cycles cannot be grouped into third-order depositional sequences (cf. Tucker & Garland, Reference Tucker and Garland2010; Zhang et al. Reference Zhang, Chen, Zhou, Guo, Wei and Mutti2015). Furthermore, boundary surfaces indicated by long-term subaerial exposure (i.e. karstification, pedogenesis, brecciation, black pebbles) have not been recognized as separating the presumed mesocycles. To sum up, eustatically controlled third-order depositional sequences like those known from other Triassic Western Tethys and Peri-Tethys basins (Calvet, Tucker & Helton, Reference Calvet, Tucker, Helton and Tucker1990; Aigner & Bachmann, Reference Aigner and Bachmann1992; Rüffer, Reference Rüffer1995; Gianolla & Jaquin, Reference Gianolla, Jaquin and de Graciansky1998; Haas & Budai, Reference Haas and Budai1999; Szulc, Reference Szulc2000; Török, Reference Török, Bachmann and Lerche2000; Zühlke, Reference Zühlke2000; Jaglarz & Szulc, Reference Jaglarz and Szulc2003; Götz & Török, Reference Götz and Török2008) cannot be detected convincingly in the whole marine part of the second-order T-R stratigraphic cycle from the Western Balkanides.
4.a.5. Lack of frame-builders
The general absence of frame-building taxa in the Triassic ramp setting (Section 4.a.6 below) promoted production of mainly loose carbonate deposits including skeletal components (Fig. 10) not organically bound during deposition, i.e. easily prone to remobilization and transport (cf. Török, Reference Török, Wright and Burchette1998; Beavington-Penney, Wright & Racey, Reference Beavington-Penney, Wright and Racey2005; Pérez-López & Pérez-Valera, Reference Pérez-López and Pérez-Valera2012; Bassi et al. Reference Bassi, Nebelsick, Puga-Bernabéu and Luciani2013). Some in situ or redeposited concentrations of crinoids, bivalves, brachiopods and dasycladaceans (Chatalov, Reference Chatalov2002, Reference Chatalov2010, Reference Chatalov2013) suggest formation of small isolated skeletal banks but not reefs proper. Therefore, microbial- or metazoan-dominated mounds and reefs such as those known from other Triassic carbonate ramps of the Western Tethys realm (Senowbari-Daryan et al. Reference Senowbari-Daryan, Zühlke, Bechstädt and Flügel1993; Calvet & Tucker, Reference Calvet, Tucker and Monty1995; Hips, Reference Hips2007; Mercedes-Martín, Arenas & Salas, Reference Mercedes-Martín, Arenas and Salas2014) were not constructed.

Figure 10. Vertical occurrence of some skeletal and non-skeletal carbonate components plus other specific features in the ramp succession. Symbols: thick line – abundant; thin line – common; dashed line – rare; TB – Terebratula Beds. Note: Dasycladaceans occur only in the Toshkovdol Fm (see Fig. 7).
The absence of metazoan frame-builders is an important control on maintaining the ramp morphology, and therefore the development of carbonate ramps may be crucially influenced by biological evolution. The delayed post-Permian recovery of carbonate-producing organisms in marine systems until the Middle Triassic epoch (Chen & Benton, Reference Chen and Benton2012) was a consequence of several extrinsic (i.e. physical environment) and/or intrinsic (i.e. ecosystem dynamics) processes (Chen & Benton, Reference Chen and Benton2012; Dineen, Fraiser & Sheehan, Reference Dineen, Fraiser and Sheehan2014; Woods, Reference Woods2014; Wei et al. Reference Wei, Shen, Schoepfer, Krystyn, Richoz and Algeo2015, and references therein). This recovery was characterized by slow reestablishment of metazoan frame-building communities in Anisian time while further diversification and proliferation occurred during the Ladinian age and especially during the Late Triassic epoch (Stanley, Reference Stanley1988; Flügel, Reference Flügel, Kiessling, Flügel and Golonka2002; Bernecker, Reference Bernecker2005; Pruss & Bottjer, Reference Pruss and Bottjer2005; Kiessling, Reference Kiessling2010). Consequently, the global lacking or reduced activity of metazoan reef-builders was a major positive factor for the development of Early Triassic and early Middle Triassic carbonate ramps worldwide (Burchette & Wright, Reference Burchette and Wright1992), and in particular across the Western Tethys shelves (Calvet, Tucker & Helton, Reference Calvet, Tucker, Helton and Tucker1990; Michalík et al. Reference Michalík, Masaryk, Lintnerová, Papšová, Jendrejáková and Reháková1992; Senowbari-Daryan et al. Reference Senowbari-Daryan, Zühlke, Bechstädt and Flügel1993; Rüffer & Zamparelli, Reference Rüffer and Zamparelli1997; Hips, Reference Hips, Wright and Burchette1998; Török, Reference Török, Wright and Burchette1998; Zühlke, Reference Zühlke2000; Rychliński & Szulc, Reference Rychliński and Szulc2005; Feist-Burkhardt et al. Reference Feist-Burkhardt and McCann2008).
It is clear, however, that biological evolution alone cannot explain the absence of frame-builders in this particular case, i.e. from the late Olenekian subage to the early Carnian subage. Thus, unfavourable environmental conditions must also have hampered the development of reef communities and formation of build-ups (cf. Török, Reference Török, Wright and Burchette1998), which was conducive to the maintenance of ramp morphology. The overall paucity or lack of metazoan and other reef builders that reappeared globally during the Anisian age (sponges, bryozoans, corals, Tubiphytes, red algae) was most likely controlled by the abundant production of lime mud (i.e. soft muddy substrate) and high-stress environment (e.g. variable salinity and oxygenation, intense storm activity) that were not favourable for massive colonization and construction of build-ups (Chatalov, Reference Chatalov2013).
4.a.6. Carbonate production
Lime mud was a volumetrically significant component of the ramp depositional system (Fig. 10). Mud production was most abundant in the peritidal environments (tidal flats, lagoons) and mid-ramp to outer ramp areas (Chatalov, Reference Chatalov, Bachmann and Lerche2000b, Reference Chatalov2013; Chatalov & Vangelov, Reference Chatalov and Vangelov2001). Although physicochemical precipitation was a presumably important mechanism at least during the late Olenekian subage (Chatalov, Reference Chatalov2007), other possible sources include mechanical breakdown of skeletal grains, bioerosion, disintegration of algal skeletons, and biologically induced or controlled precipitation (Flügel, Reference Flügel2004, p. 81; Gischler et al. Reference Gischler, Dietrich, Harris, Webster and Ginsburg2013, and references therein). Among these, the contribution of mud producers such as decaying calcareous algae, commonly cited in the literature, must have been quite limited on account of their paucity in the ramp setting (Chatalov, Reference Chatalov2007). Lime mud was a major textural constituent of Tethyan carbonate platforms during the Triassic period (Kiessling, Flügel & Golonka, Reference Kiessling, Flügel and Golonka2003), and in particular of carbonate ramps developed across the Western Tethys and in Peri-Tethyan basins (e.g. Aigner, Reference Aigner1985; Calvet, Tucker & Helton, Reference Calvet, Tucker, Helton and Tucker1990; Michalík et al. Reference Michalík, Masaryk, Lintnerová, Papšová, Jendrejáková and Reháková1992; Rüffer & Zamparelli, Reference Rüffer and Zamparelli1997; Török, Reference Török, Wright and Burchette1998; Borkhataria, Aigner & Pipping, Reference Borkhataria, Aigner and Pipping2006; Pérez-Valera & Pérez-López, Reference Pérez-Valera and Pérez-López2008; Knaust & Costamagna, Reference Knaust and Costamagna2012). According to Pomar & Hallock (Reference Pomar and Hallock2008), the origin of lime mud in the Triassic epicontinental seas could be related to increased alkalinity of seawater induced by enhanced photosynthesis of phytoplankton and photosynthetic bacteria in the neritic zone, the so-called neritic lime-mud factory. The unusual seawater chemistry, e.g. higher levels of calcium carbonate supersaturation, and the decreased role of organisms during the Early Triassic epoch may have been major controls on the massive precipitation of fine-grained carbonate in the water column (Payne et al. Reference Payne, Lehrmann, Jiayong and Knoll2006; Woods, Reference Woods2014).
The high-energy inner ramp environments were dominated by formation of ooids and intraclasts, while peloids, aggregate grains and mainly Girvanella oncoids were generated in inner to mid-ramp areas with low to moderate hydrodynamics (Chatalov, Reference Chatalov, Bachmann and Lerche2000 b, Reference Chatalov2002, Reference Chatalov2013). Ooids showing diverse morphology and cortical fabrics were restricted to fringing and barrier shoal facies (Fig. 10; Chatalov, Reference Chatalov2002, Reference Chatalov2005 b), and wave-agitated sectors of the shoal complexes were characterized by intensive precipitation of marine phreatic cements (Chatalov, Reference Chatalov2000 a). Detailed studies on the primary mineralogy of ooids and micrites have outlined an irreversible transition from aragonite-dominated precursors in the late Olenekian – middle Anisian interval to calcite-dominated precursors in the late Anisian – early Carnian interval (Chatalov, Reference Chatalov2005 a, b, Reference Chatalov2007). This temporal shift during the time of a global ‘aragonite sea’ (Sandberg, Reference Sandberg1983; Stanley & Hardie, Reference Stanley and Hardie1998) was probably controlled by the major-ion chemistry of seawater, i.e. progressive decrease of the Mg/Ca ratio during that interval of the Triassic period when the ramp carbonates were formed (e.g. Stanley & Hardie, Reference Stanley and Hardie1998; Demicco et al. Reference Demicco, Lowenstein, Hardie and Spencer2005). It is noteworthy, however, that the recognized mineralogical change of abiotic precipitation did not influence the source, type and spatial distribution of non-skeletal carbonate in the ramp depositional system.
The main living carbonate producers during the ramp evolution comprise bivalves, crinoids, brachiopods, gastropods and foraminifers, and to lesser extent ostracods, cyanobacteria, green algae (dasycladaceans) and echinoids, while red algae (solenoporaceans), ammonoids, bryozoans and scleractinian corals show very limited occcurrence (Tronkov, Reference Tronkov1968 a, Reference Tronkov1969, Reference Tronkov1973, Reference Tronkov1976; Benatov et al. Reference Benatov, Budurov, Trifonova and Petrunova1999; Chatalov, Reference Chatalov, Bachmann and Lerche2000 b, Reference Chatalov2002, Reference Chatalov2010, Reference Chatalov2013; Benatov, Reference Benatov, Brunton, Cocks and Long2001). The vertical distribution of biota in the Triassic ramp succession (Fig. 10) largely resulted from environmental controls (temperature, salinity, light, oxygenation, bathymetry, substrate, water circulation) and was closely related to the deposition of particular facies. For example, the most fossil-rich strata (Babina Fm) were deposited in mid-ramp to outer ramp settings characterized by low to moderate hydrodynamics, dominantly oxygenated bottom regime, well-illuminated water, normal salinity and soft lime mud substrate (Chatalov & Vangelov, Reference Chatalov and Vangelov2001; Chatalov, Reference Chatalov2010, Reference Chatalov2013). Contrariwise, the poor and low-diversity fossil assemblage in the peritidal strata (Svidol Fm, Opletnya Mb of Mogilata Fm, Rusinovdel Fm) reflects unfavourable environmental conditions including increased salinity, elevated temperature and episodic subaerial exposure (Chatalov, Reference Chatalov, Bachmann and Lerche2000 b) as the intertidal/supratidal deposits were exclusively dominated by cyanobacteria, i.e. forming dolomitized microbial laminites. The inner ramp shoal settings (Lakatnik Mb of Mogilata Fm, Milanovo Fm, Toshkovdol Fm) were typified by low amounts of high-diversity biota due to intensive hydrodynamics at the seafloor and the mobile character of the shoals (Chatalov, Reference Chatalov2002, Reference Chatalov2010). In particular, dasycladaceans flourished only locally in protected, near-shoal areas where ooid formation was not favoured (Toshkovdol Fm). However, apart from the regional environmental controls the temporal distribution of fauna and flora in the ramp system was apparently related to global biotic effects. Thus, the vertical occurrence of skeletal components indicates the crucial influence of biological evolution, i.e. different modes of recovery of organism groups after the end-Permian mass extinction (Aguirre & Riding, Reference Aguirre and Riding2005; Chen, Kaiho & George, Reference Chen, Kaiho and George2005; Nützel, Reference Nützel2005; Twitchett & Oji, Reference Twitchett and Oji2005; Posenato, Reference Posenato2008; Payne et al. Reference Payne, Summers, Rego, Altiner, Wei, Yu and Lehrmann2011; Song et al. Reference Song2011; Chen & Benton, Reference Chen and Benton2012; Crasquin & Forel, Reference Crasquin and Forel2013; Dineen, Fraiser & Sheehan, Reference Dineen, Fraiser and Sheehan2014, among many others). For example, representatives of some clades appear in the upper Olenekian strata (bivalves, gastropods, crinoids, foraminifers, ostracods and very rare ammonoids), which is compatible with their progressive Early Triassic recovery, especially during the Spathian subage (Chen & Benton, Reference Chen and Benton2012; Wei et al. Reference Wei, Shen, Schoepfer, Krystyn, Richoz and Algeo2015). Among them bivalves are the most abundant fossils because bivalves were the dominant shelled marine invertebrates in benthic communities during the Early Triassic epoch (Fraiser & Bottjer, Reference Fraiser and Bottjer2007). In comparison, dasycladacean algae reappeared in the Anisian age, reaching highest diversity during the Ladinian age (Aguirre & Riding, Reference Aguirre and Riding2005), and in the studied succession green algae occur exclusively in the near-shoal facies deposited around the Anisian–Ladinian boundary (Chatalov, Reference Chatalov2002). The lack of brachiopod fauna in Lower Triassic strata of the Western Balkandies conforms to the extreme rarity of Early Triassic brachiopods in the Western Tethys realm (e.g. Chen, Kaiho & George, Reference Chen, Kaiho and George2005; Posenato, Holmer & Prinoth, Reference Posenato, Holmer and Prinoth2014) despite their accelerated recovery and global dispersal during the Olenekian age (Chen, Kaiho & George, Reference Chen, Kaiho and George2005; Chen et al. Reference Chen, Tong, Song, Luo, Huang and Xiang2015). Therefore, the abundance of diverse brachiopods in the Middle Triassic ramp deposits (Tronkov, Reference Tronkov1973; Benatov et al. Reference Benatov, Budurov, Trifonova and Petrunova1999; Benatov, Reference Benatov, Brunton, Cocks and Long2001) reflects their global radiation during the Anisian age (Chen, Kaiho & George, Reference Chen, Kaiho and George2005) including the Western Tethys (e.g. Torti & Angiolini, Reference Torti and Angiolini1997; Pálfy, Reference Pálfy and Vörös2003).
The widespread global occurrence of so-called ‘anachronistic facies’ in Lower Triassic carbonate strata (e.g. Deng et al. Reference Deng, Wang, Woods, Li and Liao2015) is generally explained by specific environmental conditions and biotic controls after the end-Permian mass extinction as well as uniformitarian sedimentologic factors (Woods, Reference Woods2014). Such deposits occur in the Olenekian part of Mogilata Fm (Fig. 10) including vermicular limestones, microbially coated grains (see Woods, Reference Woods2013), subtidal mud-chip facies (sensu Pruss, Corsetti & Bottjer, Reference Pruss, Corsetti and Bottjer2005) and flat-pebble conglomerates (Fig. 11a, b, c). According to Woods (Reference Woods2013, Reference Woods2014), the unusual Early Triassic seawater chemistry, enhanced primary productivity, warm climate and limited development of benthic communities favoured the abiotic and microbial precipitation of CaCO3, rapid lithification on the seafloor, and decrease in bioturbation depth and intensity.

Figure 11. ‘Anachronistic facies’ in the upper Olenekian strata of Mogilata Fm (Opletnya Mb). (a) Vermicular limestone. Section 5. (b) Concentric and homogeneous micrite ooids interpreted to have formed from microbially mediated precipitation of fine-grained CaCO3 (see Woods, Reference Woods2013). Section 17. Scale bar is 0.3 mm across. (c) Subtidal flat-pebble conglomerate (fpc) grading into mud-chip facies (mcf). Outcrop between sections 16 and 17.
4.b. Local controls
The Triassic carbonate ramp system was also influenced by local factors (Fig. 5), i.e. manifested in some areas of the ramp during different stages of its evolution. Thus, locally elevated palaeorelief affected the ramp initialization while autogenic processes largely controlled the formation of peritidal cyclicity during the early stage of ramp retrogradation. Moreover, syndepositional tectonics was an important control on a local scale during the late stage of ramp progradation.
4.b.1. Antecedent topography
The basal carbonate deposits in exposures from the northwesternmost part of the study area, i.e. Belogradchik region (Fig. 1), are lithostratigraphically referred to as Edivetar Fm (Fig. 6). This unit has relatively small thickness (~18 m) and consists of predominant sandy bioclastic limestones and subordinate calcareous sandstones having early Anisian age (Tronkov, Reference Tronkov1973, Reference Tronkov1976; Muttoni et al. Reference Muttoni, Gaetani, Budurov, Zagorchev, Trifonova, Ivanova, Petrounova and Lowrie2000). As mentioned above, the younger age of these lowermost marine strata in the Triassic succession can be explained by advancing Tethyan transgression from the east/southeast (Section 4.a.4 above). During the Early Triassic epoch the Belogradchik region was a dryland (Tronkov, Reference Tronkov1968 b) characterized by fluvial gravel-dominated deposition in arid to semi-arid climate (Mader & Čatalov, Reference Mader, Čatalov and Mader1992 b). Several lines of evidence indicate additional local control of the antecedent topography at the time of ramp initialization. For example, the direct development of shallow subtidal setting with normal marine salinity and predominantly high-energy conditions (Chatalov, Reference Chatalov2010) is in contrast to the rest ramp areas where an extensive, low-energy carbonate tidal flat with elevated salinity of seawater (Chatalov, Reference Chatalov, Bachmann and Lerche2000 b) was formed diachronously. The accumulation of relatively thin inner ramp deposits, i.e. Edivetar Fm, and the lack of marginal-marine facies both suggest rapid transgression across an uneven palaeorelief. The local influence of a remnant dryland, and hence, the existence of rugged antecedent topography, is likewise inferred from the intensive supply of immature siliciclastics concurrently with the initial carbonate deposition (Chatalov, Reference Chatalov2010). Also, great variations in the thicknesses (30–300 m) and the grain size (sandstones v. breccia-conglomerates) of the Lower Triassic strata, i.e. Petrohan Terrigenous Group, forming the pre-ramp basement in close localities of the Belogradchik region (sections 1 and 2 in Fig. 6), imply lack of advanced peneplenation at the onset of the Middle Triassic epoch.
4.b.2. Autocyclic processes
The aforementioned elementary cycles distinguished by Ajdanlijski, Strasser & Tronkov (Reference Ajdanlijski, Strasser, Tronkov and Sinnyovsky2004) approximately correspond to the peritidal cycles described by Chatalov (Reference Chatalov2000 b). These metre-scale asymmetric cycles in the Olenekian part of Opletnya Mb (Fig. 10) show an invariably shallowing-upward trend, i.e. transition from subtidal to intertidal (Fig. 12a) and locally supratidal facies (Chatalov, Reference Chatalov, Bachmann and Lerche2000 b). The subtidal facies include decimetre-thick, commonly cross-bedded basal lags of intraclastic, ooidal or bioclastic grainstones, packstones or rudstones, and overlying metre-thick bedsets of mostly laminated calcimudstones and bioclastic wackestones. The intertidal/supratidal facies is represented by massive or laminated dolomudstones and microbial dolobindstones with desiccation cracks, tepee structures, bird's-eye fenestrae and spar-filled nodules (Fig. 12b). Dolomitic flat-pebble conglomerates (dolorudstones) occur in the topmost part of only a few cycles and probably indicate formation in a supratidal setting. The transgressive basal lags have an erosional lower bed surface and commonly contain intraclasts derived from penecontemporaneously dolomitized intertidal/supratidal facies of the underlying cycle (Fig. 12c). The cycle boundaries correspond to subplanar discontinuity surfaces produced by erosion related to marine flooding (i.e. Bádenas, Aurell & Bosence, Reference Bádenas, Aurell and Bosence2010). Thicknesses of the individual peritidal cycles vary widely from 0.90 m to 8.30 m.

Figure 12. Peritidal cyclicity in the Opletnya Mb of Mogilata Fm. (a) Shallowing-upward cycle (reverse triangle) consisting of cross-bedded transgressive basal lag (bl), laminated subtidal calcimudstones (cm) and thick intertidal/supratidal dolomite cap (dc). Elements of the underlying and overlying cycles are also visible. Cycle boundaries represent subplanar discontinuity surfaces produced by erosion related to marine flooding. Section 17. (b) Intertidal/supratidal facies including massive dolomudstone (mdm), thin layer of flat-pebble dolorudstone (fpd), and laminated microbial dolobindstone (ldb) with desiccation cracks (short arrows) and tepee structure (long arrow). Section 17. (c) Fresh rock surface of rudstone basal lag with pebble-sized intraclasts (pale yellow) derived from penecontemporaneously dolomitized intertidal/supratidal facies of the underlying cycle. Scale: coin diameter is 20 mm. Section 15. (d) Peritidal cycles showing great variability in their thicknesses, disordered stacking pattern, and variable thickness ratio between the subtidal limestone facies (grey) and the intertidal/supratidal dolomitic facies (pale yellow). Scale: height of the outcrop is 15 m. Section 16.
The formation of small-scale shallowing-upward sequences in peritidal settings can result from allocyclic (allogenic) and/or autocyclic (autogenic) processes (Strasser, Reference Strasser, Einsele, Ricken and Seilacher1991; Tresch & Strasser, Reference Tresch and Strasser2011). Allocyclic controls are independent of the depositional environment and include orbitally driven eustatic sea-level fluctuations (Goldhammer, Dunn & Hardie, Reference Goldhammer, Dunn and Hardie1987; Strasser et al. Reference Strasser, Pittet, Hillgärtner and Pasquier1999; Fischer et al. Reference Fischer, D'Argenio, Silva, Weissert, Ferreri, D'Argenio, Fischer, Silva, Weiser and Ferreri2004), or repeated synsedimentary fault movements (Cisne, Reference Cisne1986; Bosence et al. Reference Bosence2009; De Benedictis, Bosence & Waltham, Reference De Benedictis, Bosence and Waltham2007), that generate changes in the accommodation space for the cycles to form. Autocyclic processes operating within the sedimentary basin involve progradation and aggradation of sedimentary bodies (tidal flats, shoals, delta lobes), or lateral migration of tidal channels (Ginsburg, Reference Ginsburg1971; Satterley, Reference Satterley1996; Burgess, Reference Burgess2006; Pratt, Reference Pratt, James and Dalrymple2010), without being related to extrinsic factors such as tectonics and eustatic sea-level changes. The relative influence of allocyclic and autocyclic processes is difficult to estimate as they commonly interact (Strasser, Reference Strasser, Einsele, Ricken and Seilacher1991; Lehrmann & Goldhammer, Reference Lehrmann, Goldhammer, Harris, Saller and Simo1999; Burgess, Reference Burgess2006; Bosence et al. Reference Bosence2009; Tucker & Garland, Reference Tucker and Garland2010; Tresch & Strasser, Reference Tresch and Strasser2011; Hill et al. Reference Hill, Wood, Curtis and Tetzlaff2012; Laya, Tucker & Perez-Huerta, Reference Laya, Tucker and Perez-Huerta2013).
The origin of the metre-scale cyclicity can best be studied in the southern part of the Western Balkanides (Fig. 1) where the well-exposed Olenekian–Anisian peritidal succession shows maximum and persistent thickness (Figs 3, 4, 6). Field data collected from eight sections of Opletnya Mb allow tracing of the cycle numbers, boundaries, thicknesses and stacking patterns as well as the intracycle facies distributions and lateral facies heterogeneity (Fig. 13). A tectonic origin of the peritidal cycles should be considered unlikely given the regional setting of the passive continental margin, the lack of evidence for repeated fault movements, the relatively large area with cyclic peritidal sedimentation, and the absence of cycle-type variability and great thickness variations of the studied sections (e.g. Bosence et al. Reference Bosence2009; Bádenas, Aurell & Bosence, Reference Bádenas, Aurell and Bosence2010; Spalluto, Reference Spalluto2012; Amodio, Ferreri & D'Argenio, Reference Amodio, Ferreri and D'Argenio2013; Olivier et al. Reference Olivier, Brayard, Vennin, Escarguel, Fara, Bylund, Jenks, Caravaca and Stephen2015; Zhang et al. Reference Zhang, Chen, Zhou, Guo, Wei and Mutti2015). Although the influence of low-amplitude, high-frequency eustatic oscillations during the Olenekian cannot be ruled out (see Lehrmann et al. Reference Lehrmann, Yang, Wei, Yu and Xiao2001), there are clear implications for dominant autocyclic control on the formation of the peritidal cycles (assuming constant subsidence rate). Most important is the lack of evidence for subaerial exposure, erosion or vadose diagenesis in the subtidal facies (i.e. reflecting sea-level fall), which suggests that eustatic sea-level fluctuations played a minor role in the development of peritidal cyclicity (Strasser, Reference Strasser, Einsele, Ricken and Seilacher1991; Lehrmann & Goldhammer, Reference Lehrmann, Goldhammer, Harris, Saller and Simo1999; Burgess, Reference Burgess2006). In contrast, short-lived subaerial exposure is indicated only by the intertidal and supratidal facies forming the cycle tops which are bounded by the flooding surface of the next cycle. Moreover, the lack of supratidal facies directly superimposed on subtidal facies, i.e. indicative of abrupt shallowing, or muddy lagoonal deposits overlying directly intertidal/supratidal facies, i.e. indicative of abrupt deepening, is not consistent with the assumption of overriding eustatic control (Pomoni-Papaioannou, Reference Pomoni-Papaioannou2008; Spalluto, Reference Spalluto2012). Other features of the studied sections that likewise imply a dominant autocyclic mechanism include the different numbers of cycles and great variability in the cycle thicknesses (Fig. 12d). Also, common lateral facies heterogeneity is revealed by the highly variable thickness ratio between the subtidal and intertidal/supratidal facies as well as between the transgressive basal lags and lagoonal mudstones/wackestones (Fig. 13). Taken together, these characteristics result in generally very poor correlation of the peritidal cycles even over short distances of several kilometres. Other evidence against a prevailing eustatic control is the absence of ordered hierarchical organization of the cycles, such as distinctive upward trends of decreasing or increasing cycle thicknesses, and thinning or thickening intracycle bed thicknesses (Section 4.a.4 above). During greenhouse times such as the Triassic period, autocyclic processes would be expected to override the reduced forcing potential of high-frequency low-amplitude eustasy, resulting in relatively high levels of randomness in the facies occurrence and in the cycle stacking patterns (Lehrmann & Goldhammer, Reference Lehrmann, Goldhammer, Harris, Saller and Simo1999). However, the simple operation of autocyclic processes (i.e. shoreline progradation) to produce a disordered stacking pattern (Lehrmann & Goldhammer, Reference Lehrmann, Goldhammer, Harris, Saller and Simo1999) was challenged by Burgess (Reference Burgess2006) who demonstrated through forward modelling that changes in stochastic processes such as carbonate production rate and sediment transport direction are necessary to generate random stratal patterns. Therefore, other factors inherent to the depositional system, for example, carbonate productivity, accumulation rate, depositional topography and hydrodynamic conditions (Strasser et al. Reference Strasser, Pittet, Hillgärtner and Pasquier1999; Burgess, Reference Burgess2006; Bádenas, Aurell & Bosence, Reference Bádenas, Aurell and Bosence2010; Yang & Lehrmann, Reference Yang and Lehrmann2014), may also have contributed to the variable cycle thicknesses, lateral facies heterogeneity and disordered stacking pattern in the studied peritidal succession.

Figure 13. Shallowing-upward peritidal cycles in eight sections of Mogilata Fm (Opletnya Mb) from the southern part of the Western Balkanides. The different numbers of cycles, great variability in the cycle thicknesses, absence of ordered hierarchical organization, lateral facies heterogeneity, and very poor correlation of the cycles even over short distances (see also Fig. 1) plus some other characteristics (see text) suggest dominant autogenic control on the formation of the metre-scale cyclicity. The absence of any trend upsection and boundary surfaces indicated by long-term subaerial exposure rules out grouping of the cycles into third-order depositional sequences as suggested by Ajdanlijski, Strasser & Tronkov (Reference Ajdanlijski, Strasser, Tronkov and Sinnyovsky2004).
The autocyclic mechanism is considered herein as having been effective on a local scale because the occurrence of peritidal cyclicity is not known from Olenekian shallow marine carbonate successions outcropping in other regions of Bulgaria, e.g. exposures and boreholes from the adjacent Moesian Platform, Central Balkanides, and western Srednogorie Zone (Fig. 1, inset), even within strata of the same lithostratigraphic unit (Mogilata Fm). Also, the absence of such documented cyclicity on a broader regional scale, i.e. in platform carbonates deposited around the Olenekian–Anisian boundary across the NW Tethys shelf area, further supports the hypothesis for dominant autogenic controls on the formation of peritidal cycles during the early stage of ramp retrogradation.
4.b.3. Syndepositional tectonics
A recent study of the Anisian mid-ramp to outer ramp deposits (Babina Fm) from the southern part of the Western Balkanides suggested close correspondence to homoclinal morphology of the Triassic carbonate ramp (Chatalov, Reference Chatalov2013). However, new field data reveal that the upper Zgorigrad Mb of the same formation (Fig. 3) exposed in the Vratsa region (Fig. 1) shows significant differences in terms of total thickness, stratification pattern, sedimentary structures, lithology and depositional textures compared to the rest studied sections (Table 1; Fig. 14). For example, the Zgorigrad Mb is much thicker, being characterized by variable bed thicknesses (Fig. 15a) and dominant lithofacies of calcimudstones and bioclastic wackestones (cf. Chatalov, Reference Chatalov2013). The most distinctive lithological feature is abundance of clay-sized siliciclastics forming marly rock matrix and intrabed layers or laminae that are dolomitized to various degrees. Typical sedimentary structures include nodular bedding, i.e. limestone nodules in marly matrix, and heterolithic bedding, i.e. alternating limestone and marly layers, while slumps (Fig. 15b) and slides (Fig. 15c) are only locally observed. The interpretation of gravity slides v. erosional channels is based on the similar lithology, structure and microfabric of the carbonate beds (nodular calcimudstones) on both sides of the slide surface (Pedley, Cugno & Grasso, Reference Pedley, Cugno and Grasso1992; Knaust, Reference Knaust, Bachmann and Lerche2000; Sherman, Narbonne & James, Reference Sherman, Narbonne and James2001; Zonneveld et al. Reference Zonneveld, Beatty, Williford, Orchard and McRoberts2010). The uppermost part of Zgorigrad Mb consists of dolomitic ribbon rocks, i.e. laminar arrangement of calcimudstones and dolomitized marlstones, showing abrupt boundary with the overlying thick-bedded shoal facies of Milanovo Fm. In many nodular beds the carbonate nodules ‘float’ within the marly matrix, having sharp contacts, smaller size, higher degree of roundness and sphericity, and better sorting (Fig. 15d), compared to adjacent sectors of the rock which display compact arrangement and common coalescence of nodules (Fig. 15e). Elsewhere, poorly sorted limestone intraclasts also ‘float’ within marly matrix, showing pebble size, flat to irregular shape, rounded edges, subparallel orientation of their long axes to the bedding planes, and monomict composition (Fig. 15f). All intrabed sectors with such intraclasts grade from underlying heterolithic limestone–marl layers that have undergone in situ brecciation resulting in the formation of closely spaced limestone flat pebbles. Similarly, most dolomitic laminae in the ribbon rocks contain variously rounded limestone intraclasts with diverse shapes and sizes while the limestone laminae are locally cracked and/or brecciated (Fig. 15g).
Table 1. Comparative stratigraphic and sedimentological characteristics of the Zgorigrad Mb from two regions in the Western Balkanides (data on the southern exposures are from Chatalov, Reference Chatalov2013).


Figure 14. Stratigraphic–lithologic logs of the Zgorigrad Mb in section 8 from the Vratsa region (left) and section 17 from the southern area (right). The former contains explicit evidence for local distal steepening of the carbonate ramp as a result of syndepositional tectonism (see Table 1).

Figure 15. Macroscopic characteristics of Zgorigrad Mb in the Vratsa region (section 8) suggesting distal steepening of the ramp profile and gravity-driven transport. (a) Outcrop of the unit showing variable bed thicknesses (MlFm – Milanovo Fm; ZmMb – Zimevitsa Mb). (b) Slump in dolomitic ribbon rocks. Scale: hammer is 31 cm long. (c) Slide developed between two beds of nodular limestones (slide surface shown by hammer). (d) Redeposited limestone nodules ‘floating’ in marly matrix. (e) Nodular bedding with compact arrangement and common coalescence of in situ grown limestone nodules. (f) Intrabed transition between autobrecciated limestone layers (arrows) alternating with marly layers and pebble-sized limestone intraclasts ‘floating’ in marly matrix. The elongated intraclasts show subparallel orientation to the bedding direction (indicated by double-ended arrow). (g) Ribbon rocks with intraclasts (arrows) derived from the calcimudstone laminae and embedded in the dolomitic laminae.
The described strata of Zgorigrad Mb in the Vratsa region are interpreted as mid-outer ramp deposits on the basis of the following characteristics: abundance of lime mud and fine-grained siliciclastics, dominant nodular bedding, scarce bioturbation, low amount of benthic fauna with absence of phototrophic organisms, presence of ammonites (Tronkov, Reference Tronkov1976), prevailing mud-supported fabrics, and lack of depositional structures and textures indicating high-energy conditions or tidal influence. Nodular limestones appear to be particularly common in deeper-marine and slope settings (Flügel, Reference Flügel2004, p. 200) and are a widespread deep-water facies in the Triassic successions of the Western Tethys realm (Preto et al. Reference Preto, Spötl, Mietto, Gianolla, Riva and Manfrin2005). Although heterolithic carbonates are formed in various depositional environments, i.e. from peritidal to outer ramp, in this case low-energy deep subtidal setting (cf. Markello & Read, Reference Markello and Read1981; Sherman, Narbonne & James, Reference Sherman, Narbonne and James2001; Chen et al. Reference Chen, Chough, Chun and Han2009; Dilliard et al. Reference Dilliard, Pope, Coniglio, Hasiotis and Lieberman2010; Kim et al. Reference Kim, Rhee, Woo and Park2014; Bayet-Goll et al. Reference Bayet-Goll, Chen, Moussavi-Harami and Mahboubi2015; Gomez & Astini, Reference Gomez and Astini2015) is suggested by the dominant mudstone fabrics, high clay content, rare skeletal remains, and lack of evidence for wave reworking, current activity or subaerial exposure. Moreover, the dolomitic ribbon rocks are associated with slumps making deposition in shallow water less probable (cf. Sherman, Narbonne & James, Reference Sherman, Narbonne and James2001; Knight & Boyce, Reference Knight and Boyce2009; Turner, Reference Turner2009; Gomez & Astini, Reference Gomez and Astini2015). The mid-outer ramp environment was characterized by fair-weather sedimentation as is indicated by the absence of storm-related features (e.g. graded sheets, coquina beds, gutter casts, hummocky cross-lamination, sharp erosional bases) in the rocks of Zgorigrad Mb from all studied sections in the Western Balkanides (Chatalov, Reference Chatalov2013). Low hydrodynamics prevailed on the seafloor as deposition took place mainly from suspension settling of lime mud and clay-sized siliciclastics below fair-weather wave base. Reduced sedimentation rates are suggested in particular by the formation of limestone nodules (Flügel, Reference Flügel2004, p. 201).
The Anisian mid-outer ramp facies outcropping in the Vratsa region contain macroscopic evidence for distal steepening of the ramp profile, for example, the occurrence of slumps and slides such as those described from slope deposits of ancient distally steepened ramps (Gawthorpe, Reference Gawthorpe1986; Pedley, Cugno & Grasso, Reference Pedley, Cugno and Grasso1992; Elrick, Reference Elrick1996; Hips, Reference Hips, Wright and Burchette1998; Sherman, Narbonne & James, Reference Sherman, Narbonne and James2001; Drzewiecki & Simó, Reference Drzewiecki and Simó2002; Cozzi, Grotzinger & Allen, Reference Cozzi, Grotzinger and Allen2004; Decarlis & Lualdi, Reference Decarlis and Lualdi2009; Dilliard et al. Reference Dilliard, Pope, Coniglio, Hasiotis and Lieberman2010; Zonneveld et al. Reference Zonneveld, Beatty, Williford, Orchard and McRoberts2010; Martin-Rojas et al. Reference Martin-Rojas, Somma, Delgado, Estévez, Iannace and Zamparelli2012; Delpomdor, Kant & Préat, Reference Delpomdor, Kant and Préat2014). Also, the intrabed gradation between isolated limestone nodules in marly matrix and compact nodular structure implies reworking of early lithified nodules that may be due to oversteepened slope. Although redeposition of carbonate nodules has been attributed to wave reworking, storm-induced and other currents (Mount & Kidder, Reference Mount and Kidder1993; Kim & Lee, Reference Kim and Lee1996; Myrow et al. Reference Myrow, Tice, Archuleta, Clark, Taylor and Ripperdan2004; Bertok et al. Reference Bertok, Martire, Perotti, d'Atri and Piana2011), transport by sediment gravity flows has been documented by several authors (Föhlisch & Voigt, Reference Föhlisch, Voigt, McCaffrey, Kneller and Peakall2001; Preto et al. Reference Preto, Spötl, Mietto, Gianolla, Riva and Manfrin2005; Whalen & Beatty, Reference Whalen, Beatty, Reifenstuhl and Decker2008; Dilliard et al. Reference Dilliard, Pope, Coniglio, Hasiotis and Lieberman2010; Ferry et al. Reference Ferry, Grosheny, Backert and Atrops2015). The rounded limestone pebbles ‘floating’ in marly matrix likewise suggest downslope movement of semi-consolidated sediments as a result of slope failure. This conclusion is supported by the observed upward transition from undisturbed and/or brecciated heterolithic layers. Apparently, the gravity-driven transport was preceded by early diagenetic autobrecciation or autoconglomeration, i.e. in situ fragmentation of carbonate layers in the limestone–marl couplets (or limestone–dolomite couplets in the ribbon rocks) and subsequent rounding of the generated intraclasts (Kwon et al. Reference Kwon, Chough, Choi and Lee2002; Chen et al. Reference Chen, Chough, Chun and Han2009). Similar intraformational breccias/conglomerates commonly associated with ribbon carbonates and/or slumps and slides have been reported from slope facies of many ancient distally steepened ramps (Gawthorpe, Reference Gawthorpe1986; Elrick, Reference Elrick1996; Sherman, Narbonne & James, Reference Sherman, Narbonne and James2001; Drzewiecki & Simó, Reference Drzewiecki and Simó2002; Mukhopadhyay & Chaudhuri, Reference Mukhopadhyay and Chaudhuri2003; Cozzi, Grotzinger & Allen, Reference Cozzi, Grotzinger and Allen2004; Dilliard et al. Reference Dilliard, Pope, Coniglio, Hasiotis and Lieberman2010; Zonneveld et al. Reference Zonneveld, Beatty, Williford, Orchard and McRoberts2010; Martin, Montañez & Bishop, Reference Martin, Montañez and Bishop2012; Martin-Rojas et al. Reference Martin-Rojas, Somma, Delgado, Estévez, Iannace and Zamparelli2012; Delpomdor, Kant & Préat, Reference Delpomdor, Kant and Préat2014; Thomson, Rainbird & Dix, Reference Thomson, Rainbird and Dix2014). To sum up, the lack of any evidence for tidal, wave or storm reworking in the strata of Zgorigrad Mb reinforces the interpretation of gravity-influenced transport as a result of slope instability, i.e. distal steepening of the ramp profile. Because the slope angle was dependent on the sediment fabric (Kenter, Reference Kenter1990), the predominantly mud-rich, cohesive deposits maintained low angles of repose, i.e. with values below 15° for mud-supported fabrics and below 5° for pure muds. It should be noted that the initial deformation of heterolithic and nodular layers and the formation of slumps and slides may have been triggered by an external process, for example, seismic shocks (Montenat et al. Reference Montenat, Barrier, Ott d'Estevou and Hibsch2007) predating the gravity-driven mass movement (Pedley, Cugno & Grasso, Reference Pedley, Cugno and Grasso1992; Föhlisch & Voigt, Reference Föhlisch, Voigt, McCaffrey, Kneller and Peakall2001; Kullberg et al. Reference Kullberg, Oloriz, Marques, Caetano and Rocha2001; Drzewiecki & Simó, Reference Drzewiecki and Simó2002; Dilliard et al. Reference Dilliard, Pope, Coniglio, Hasiotis and Lieberman2010; Bertok et al. Reference Bertok, Martire, Perotti, d'Atri and Piana2011). However, this assumption is difficult to prove because seismites have no specific morphological features and proposed diagnostic criteria in the literature for recognition of a seismic trigger seem unreliable due to a number of limitations (Owen, Moretti & Alfaro, Reference Owen, Moretti and Alfaro2011; Moretti & van Loon, Reference Moretti and Van Loon2014).
Distal steepening of carbonate ramps may be caused by antecedent topography, syndepositional tectonics (differential subsidence, extensional faulting), or different sedimentation rates between shallow-water and deep-water areas (Read, Reference Read1985; Burchette & Wright, Reference Burchette and Wright1992; Pomar, Reference Pomar2001 b; Pomar & Kendall, Reference Pomar, Kendall, Lukasik and Simo2008). In this case, the first possibility should be ruled out in view of the low-gradient relief developed at the time of ramp initialization and the persistent thickness of carbonate strata below Zgorigrad Mb across the whole southern part of the study area (Table 1; Figs 6, 14). In the second place, there is no explicit evidence for higher sedimentation rate in the shallow ramp (e.g. reef-dominated shelf edge), and moreover, such potential control on oversteepening would have been manifested on a basin-wide scale. Therefore, the local initialization of a ramp slope must have been caused by synsedimentary tectonics similarly to other known examples from the geological record (Gawthorpe, Reference Gawthorpe1986; Gómez-Pérez, Fernández-Mendiola & García-Mondéjar, Reference Gómez-Pérez, Fernández-Mendiola, García-Mondéjar, Wright and Burchette1998; Rosales, Reference Rosales1999; Drzewiecki & Simó, Reference Drzewiecki and Simó2002; Playton & Kerans, Reference Playton and Kerans2002; Cozzi, Grotzinger & Allen, Reference Cozzi, Grotzinger and Allen2004; Bover-Arnal et al. Reference Bover-Arnal, Moreno-Bedmar, Salas, Skelton, Bitzer and Gili2010; Dilliard et al. Reference Dilliard, Pope, Coniglio, Hasiotis and Lieberman2010; Martin-Rojas et al. Reference Martin-Rojas, Somma, Delgado, Estévez, Iannace and Zamparelli2012; Delpomdor, Kant & Préat, Reference Delpomdor, Kant and Préat2014). The great lateral thickness variations and lithofacies change in the Zgorigrad Mb between the two regions considered (Table 1; Fig. 14) suggests that syndepositional tectonics created differential accommodation space across the ramp during a time of relative sea-level fall (Fig. 3). Enhanced subsidence rate that was probably fault-controlled may, in particular, explain the increased accommodation space in the Vratsa region and the local configuration of distally steepened ramp morphology (Fig. 16). Thus, in terms of genetic classification on the basis of basinal setting and tectonic control (Bosence, Reference Bosence2005), the Triassic carbonate ramp of the Western Balkanides is clearly defined as a subsiding margin-type platform with homoclinal geometry but shows, on a local scale, the characteristics of a fault-block-type platform with steeper-margined profile (cf. Martin-Rojas et al. Reference Martin-Rojas, Somma, Delgado, Estévez, Iannace and Zamparelli2012).
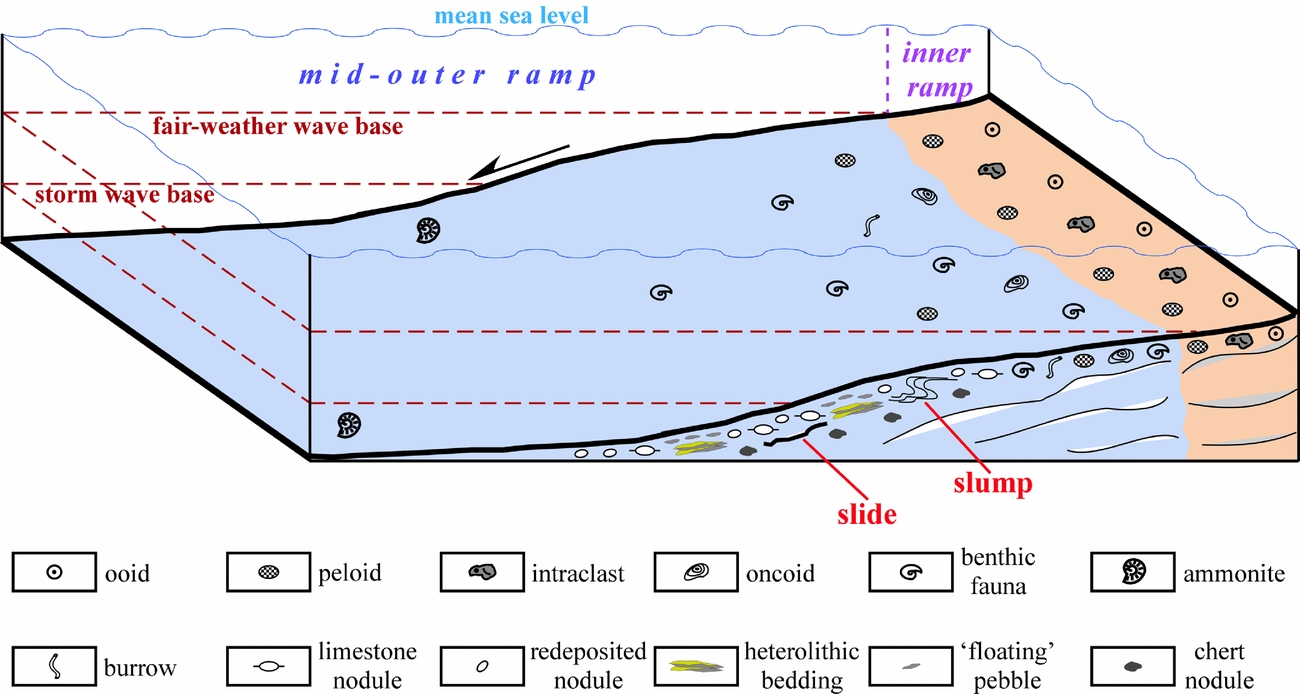
Figure 16. Depositional model for the Zgorigrad Mb in section 8 showing locally developed distal steepening of the ramp profile as an effect of synsedimentary tectonics.
5. Summary and conclusions
Various global and regional factors controlled the Early to Late Triassic development of a carbonate ramp on the subtropical shelf area of the NW Tethys. Geotectonic setting, antecedent topography, climate and oceanographic regime, relative sea-level changes, lack of frame-builders and carbonate production acted as independent or superimposed controls. The late Olenekian initialization and subsequent growth of the ramp system were favoured by slow uniform subsidence on a passive continental margin, gentle relief inherited from extensive fluvial and marginal-marine deposition during the Early Triassic epoch, stable warm and dry climatic conditions with significant influence by subtropical storms, west/northwestward Tethyan transgression along with eustatic sea-level rise, and absence of metazoan reef-builders. The interplay of these extrinsic and intrinsic controls promoted long-term maintenance of a homoclinal ramp morphology and accumulation of relatively thick carbonate strata (~500 m). The sedimentation was characterized by abundant lime mud production, intensive formation of coated grains, intraclasts and peloids, and precipitation of marine phreatic cements. The development and distribution of diverse carbonate-secreting organisms was controlled by environmental conditions and global biotic effects after the end-Permian mass extinction. A specific feature was the deposition of Early Triassic ‘anachronistic facies’ as a consequence of several interacting factors. From the Pelsonian subage onwards, a relative sea-level fall led to progressive shallowing and final demise of the ramp system during the Julian subage. After a period of subaerial exposure and minor karstification the deposition of continental first-cycle quartz arenites suggests possible impact of the Carnian Pluvial Episode.
The carbonate ramp system was also influenced by local factors such as remnant uplifted relief which prevented the basin-wide development of tidal flat environment at the time of ramp initialization. Autocyclic processes and presumably other factors inherent to the depositional system largely controlled the formation of peritidal cycles with disordered stacking pattern during the Olenekian age, although the concomitant influence of high-frequency eustatic oscillations cannot be ruled out. Distal steepening of the ramp profile on a local scale was caused by syndepositional tectonics in Pelsonian–Illyrian time. The configuration of a ramp slope resulted from probably fault-driven differential subsidence which created additional accommodation space, thus overriding the relative sea-level fall.
The upper Olenekian to lower Carnian marine succession of the Western Balkanides more or less resembles other ramp carbonates deposited along a broad belt on the NW Tethys shelf area during the Triassic period. Some comparable characteristics include sediment thickness, stacking pattern, lithofacies types, sedimentary structures and fossil content, thus suggesting great similarities with respect to the ramp model, general evolution of the ramp system, sedimentation rate, depositional settings, environmental constraints, source of carbonate, and biota development. These similarities can be explained by the close palaeogeographic position of the carbonate ramps but also by analogous global and regional controlling factors.
This study has presented a comprehensive and systematic analysis of the complex array of different mechanisms and processes that may control the birth, growth and demise of carbonate ramps. It shows that only the interplay of various allogenic and autogenic factors can exercise control over the origin and development of ramp systems by regulating their geometry, size, sedimentation style and other depositional characteristics. Because the studied Triassic carbonate ramp evolved in a semi-arid to arid subtropical climate it may be regarded as a typical example of greenhouse storm-influenced ramps. The results obtained demonstrate that factors intrinsic to the depositional system may have dominant control over the formation of shallowing-upward peritidal cycles on carbonate ramps during greenhouse periods.
Acknowledgements
The author is grateful to Y. Stefanov (Faculty of Geology and Geography, Sofia University ‘St Kliment Ohridski’) for taking part in several field trips and for useful discussions on some problems of this study. The critical remarks and suggestions of two anonymous reviewers are gratefully acknowledged.
Declaration of interest
The author declares no conflict of interest related to this article.