Introduction
Influenza is a highly contagious disease that represents one of the most serious health and economic threats to humans and animals worldwide. In order to understand the epidemiology of influenza, it is critical to recognize that influenza A viruses infect a wide variety of species. Moreover, the viruses exhibit only partial restriction of their host range such that viruses from one species can occasionally transmit to infect another species (Webster et al., Reference Webster, Bean, Gorman, Chambers and Kawaoka1992; Webby and Webster, Reference Webby and Webster2001). Historically, only a limited number of subtypes of influenza viruses have been associated with widespread infection of mammals (Webster et al., Reference Webster, Bean, Gorman, Chambers and Kawaoka1992; Alexander and Brown, Reference Alexander and Brown2000). However, viruses of all 16 hemagglutinin (HA) and nine neuraminidase (NA) subtypes have been recovered from wild waterfowl and seabirds (Webster et al., Reference Webster, Bean, Gorman, Chambers and Kawaoka1992; Webby and Webster, Reference Webby and Webster2001). As such, waterfowl provide a vast global reservoir of influenza viruses in nature from which novel viruses can emerge to infect mammalian species (Webster et al., Reference Webster, Bean, Gorman, Chambers and Kawaoka1992; Webby and Webster, Reference Webby and Webster2001). Undoubtedly, the most prominent examples of direct transmission of avian viruses to mammalian species are the recent infections of humans and cats with the highly pathogenic avian H5N1 viruses (de Jong et al., Reference de Jong, Claas, Osterhaus, Webster and Lim1997; Claas et al., Reference Claas, Osterhaus, van Beek, De Jong, Rimmelzwaan, Senne, Krauss, Shortridge and Webster1998b; Kuiken et al., Reference Kuiken, Rimmelzwaan, van Riel, van Amerongen, Baars, Fouchier and Osterhaus2004; Webster et al., Reference Webster, Guan, Poon, Krauss, Webby, Govorkovai and Peiris2005). Yet, while these examples clearly demonstrate that cross-species transmission of viruses can occur, it has long been recognized that barriers exist that limit transmission of influenza viruses among species (Webster et al., Reference Webster, Bean, Gorman, Chambers and Kawaoka1992; Webby and Webster, Reference Webby and Webster2001).
In general, the ability of any given virus to cross from one species to another is dependent on epidemiological factors as well as host and viral factors. For example, some viruses are prevented from entering a new host species simply by the absence of the appropriate receptor (Morse, Reference Morse1997). Other viruses are able to enter the host cell, yet, they are unable to complete their replication cycle (Morse, Reference Morse1997). Many viruses that have demonstrated the ability to transmit between species that contain RNA genomes. As viral RNA polymerases lack proofreading functions, RNA viruses generally demonstrate high mutation rates (for influenza A viruses the mutation rate is estimated at one point mutation/1.5×105 nucleotides) (Buonagurio et al., Reference Buonagurio, Nakada, Parvin, Krystal, Palese and Fitch1986), with consequent potential for rapid evolution. As this mutation rate is sufficiently high to yield one or more point mutations in each progeny viral genome per round of replication, viral stocks often represent a population of genomes, a ‘quasispecies’ (Eigen and Schuster, Reference Eigen and Schuster1977), rather than a homogenous population (Morse, Reference Morse1997). This genetic diversity allows plasticity within the viral population, for example, for adaptation to a new environment such as a new host species. Despite this, many viruses show remarkable genetic stability in their natural hosts. In waterfowl, influenza viruses are generally highly host-adapted, as evidenced by low evolutionary rates (‘evolutionary stasis’) (Gammelin et al., Reference Gammelin, Altmuller, Reinhardt, Mandler, Harley, Hudson, Fitch and Scholtissek1990; Gorman et al., Reference Gorman, Bean and Webster1992; Webster et al., Reference Webster, Bean, Gorman, Chambers and Kawaoka1992; Webby and Webster, Reference Webby and Webster2001).
As a general rule, upon introduction into a new environment (i.e. a new host species), selection of mutants that are most ‘fit’ (i.e. replicate most efficiently in the new environment) will be selected from within the population of virus genomes. The selective pressure may affect regions in the virus genome that convey a replication advantage, control species specificity, or correspond to antigenic sites (Morse, Reference Morse1997). The genetic diversity within a virus population is determined by the balance between the emergence of new mutants and the extinction of circulating variants through competition (Ferguson et al., Reference Ferguson, Galvani and Bush2003). For human influenza viruses, selection by the host immune system is thought to be the driving force in the production of influenza genetic diversity. As protection conferred by influenza-specific immunoglobulins decreases with increasing genetic divergence of the HA, cross-protection tends to decrease as the antigenic divergence between two strains increases (Ferguson et al., Reference Ferguson, Galvani and Bush2003). Theoretically, this should result in the selection of antigenically novel strains and subsequent exponential growth of influenza virus diversity. Yet, at any given time, human influenza virus strains demonstrate a surprisingly limited genetic diversity (Ferguson et al., Reference Ferguson, Galvani and Bush2003). Although recent results indicate that multiple lineages of virus strains are represented in the influenza virus population (Ghedin et al., Reference Ghedin, Sengamalay, Shumway, Zaborsky, Feldblyum, Subbu, Spiro, Sitz, Koo, Bolotov, Dernovoy, Tatusova, Bao, St George, Taylor, Lipman, Fraser, Taubenberger and Salzberg2005), human influenza virus evolution seems to be characterized by the continuous replacement of circulating strains (Webster et al., Reference Webster, Bean, Gorman, Chambers and Kawaoka1992; Webby and Webster, Reference Webby and Webster2001). In fact, phylogenetic analyses suggest that genetic evolution of human influenza viruses follows a multi-strain population dynamic, in which 95% of strains are maintained in the population for less than one year. Only approximately 1% of influenza virus strains will become established in the human population on a global scale (Fitch et al., Reference Fitch, Bush, Bender and Cox1997; Ferguson et al., Reference Ferguson, Galvani and Bush2003). More recent results by Wolf and colleagues (Wolf et al., Reference Wolf, Viboud, Holmes, Koonin and Lipman2006) indicate that evolution of human influenza viruses may not be linear, but rather occurs in periods of rapid fitness change (and displacement of old lineages with new dominant ones), followed by intervals of relative evolutionary stasis of the influenza virus genome. These periods of stasis are characterized by generally neutral sequence substitutions without apparent changes in the antigenic properties of the virus and, thus, only slow extinction of coexisting virus lineages (Wolf et al., Reference Wolf, Viboud, Holmes, Koonin and Lipman2006). Regardless of the selection mechanisms involved, the subsequent step in virus emergence hinges on the virus's ability to maintain itself in the new population. This step requires efficient transmission of the virus among individuals of the new species and is dependent on viral factors (such as replication potential), population factors (such as host density), and host factors (such as immune status and response to the pathogen) (Morse, Reference Morse1997).
Given the plasticity of the virus genome, influenza fulfills the prerequisites of a virus with emerging disease potential (Webster et al., Reference Webster, Wright, Castrucci, Bean and Kawaoka1993). It is highly likely that sometime in the near future a ‘new’ influenza A virus, be it one of the H5N1 viruses currently circulating in the wild bird population in large parts of Asia or a different virus, will be able to emerge from its animal reservoir to cause widespread disease in mammalian species. The impact of influenza in humans and animals, whether measured by morbidity, mortality or economic losses, is substantial. It is, therefore, essential to understand the precise epidemiological and molecular mechanisms that allow these viruses to jump species barriers and establish themselves in new populations. This review focuses on transmission of viruses between species, discussing both direct transmission of viruses from aquatic birds to mammals and virus transmission between mammalian species. This also includes a discussion of the molecular factors that are thought to affect influenza virus species specificity.
Etiology
Influenza viruses are members of the family Orthomyxoviridae and are enveloped viruses with segmented, single-stranded, negative-sense RNA genomes (schematically depicted in Fig. 1). The Orthomyxoviridae comprise five genera; influenza A, B, and C viruses, thogotovirus, and isavirus (Wright and Webster, Reference Wright and Webster2006). Influenza A viruses are distinguished from types B and C based on genetic and antigenic differences in their nucleoprotein (NP) and matrix (M) proteins. In addition, influenza A and B viruses contain eight separate segments of single-stranded RNA, whereas influenza C viruses possess only seven. In contrast to influenza A viruses that infect a wide variety of animals, influenza B viruses are primarily human pathogens. Influenza C viruses have most commonly been isolated from humans, but these viruses can also infect pigs and dogs (Ohwada et al., Reference Ohwada, Kitame, Sugawara, Nishimura, Homma and Nakamura1987; Manuguerra et al., Reference Manuguerra, Hannoun, Simon, Villar and Cabezas1993).

Fig. 1. Schematic diagram of structural components of influenza A virus. Three integral membrane proteins – HA, NA, and the ion channel protein (M2) – are embedded in the lipid envelope of the virion. The matrix protein (M1) underlies the lipid envelope. Associated with the viral RNA is the polymerase complex, consisting of PA, PB1, and PB2. The viral nucleoprotein (NP) encapsidates the viral RNA segments.
Influenza A virions possess a host-cell-derived lipid envelope, are 80–120 nm in diameter, and, if propagated in eggs or cell culture, have a fairly regular spherical appearance. In contrast, on initial isolation from humans or animals, influenza A viruses exhibit pleomorphism (Lamb and Krug, Reference Lamb and Krug2006). Embedded in the lipid envelope are the HA and NA, forming about 500 spikes radiating outward, and the integral M2 protein, which functions as an ion channel (Lamb and Krug, Reference Lamb and Krug2006). The HA serves as the viral receptor-binding protein and mediates fusion of the virus envelope with the host cell membrane (Wharton et al., Reference Wharton, Weis, Skehel and Wiley1989; Skehel and Wiley, Reference Skehel and Wiley2000). Each monomer of the trimeric HA protein consists of a globular head, made up exclusively of HA1, and a stalk, which consists of all of HA2 and parts of HA1 (Lamb and Krug, Reference Lamb and Krug2006). The globular head portion contains the receptor-binding site, which is comprised of an antibody-inaccessible pocket. Thus protected from immunological pressure, the amino acid residues located in the receptor-binding site are largely conserved among subtypes (Wilson et al., Reference Wilson, Skehel and Wiley1981; Wharton et al., Reference Wharton, Weis, Skehel and Wiley1989; Skehel and Wiley, Reference Skehel and Wiley2000). The HA is the major target of the host humoral immune response. There are five antigenic regions that cover much of the surface of the globular head portion of the molecule. Host immune pressure is the driving force in selecting mutant viruses with amino acid substitutions in these antigenic sites, a process also referred to as ‘antigenic drift’ (Fig. 2) (Wharton et al., Reference Wharton, Weis, Skehel and Wiley1989; Lamb and Krug, Reference Lamb and Krug2006).

Fig. 2. Schematic diagram illustrating antigenic drift. The influenza A virus RNA polymerase lacks proofreading function. The mutation rate of the influenza virus genome is sufficiently high to yield one or more point mutations (represented by the ‘X’) in each progeny viral genome per round of replication. Host immune pressure is the driving force in the selection of mutants with amino acid substitutions in antigenic sites on the HA and NA envelope glycoproteins.
The NA is a type II integral membrane protein and is the second large glycoprotein embedded in the influenza virus envelope (Varghese et al., Reference Varghese, Laver and Colman1983; Colman et al., Reference Colman, Laver, Varghese, Baker, Tulloch, Air and Webster1987). The NA is responsible for the cleavage of the α-ketosidic linkage between a sialic acid (SA) molecule and an adjacent sugar residue (Gottschalk, Reference Gottschalk1957). Biologically, the protein assists in the release of budding virus particles by removing SA residues from the viral glycoproteins as well as the infected cell (Palese et al., Reference Palese, Tobita, Ueda and Compans1974; Bucher and Palese, Reference Bucher and Palese1975; Air and Laver, Reference Air and Laver1989). More recent data also indicate that the NA plays an essential role in virus invasion of the respiratory tract by catalyzing the cleavage of the α-ketosidic linkage between the terminal SA and the adjacent sugar residue in mucus (Castrucci and Kawaoka, Reference Castrucci and Kawaoka1993; Matrosovich et al., Reference Matrosovich, Matrosovich, Gray, Roberts and Klenk2004b). Like the HA, the NA contains antigenic determinants and undergoes substantial antigenic variation in response to immune pressure (Wright and Webster, Reference Wright and Webster2006).
The M2 protein, the third envelope glycoprotein present in the influenza virion, serves as an ion channel (Pinto et al., Reference Pinto, Holsinger and Lamb1992; Wang et al., Reference Wang, Takeuchi, Pinto and Lamb1993; Holsinger et al., Reference Holsinger, Nichani, Pinto and Lamb1994). The M2 ion channel is activated at low pH and allows hydrogen ions to enter the virion during uncoating. In addition, M2 modulates the pH of the Golgi apparatus, thus preventing premature conformational change of the HA protein prior to virus assembly (Hay, Reference Hay1992; Cleverley et al., Reference Cleverley, Geller and Lenard1997; Liu and Ye, Reference Liu and Ye2002; Wright and Webster, Reference Wright and Webster2006). The M1 protein is the most abundant protein present in the influenza virion. M1 lies beneath the lipid envelope, providing rigidity to the membrane that surrounds the eight ribonucleoprotein (RNP) complexes (Lamb and Krug, Reference Lamb and Krug2006). Each RNP complex consists of a single RNA segment, encapsidated by NP molecules, as well as the three polymerase proteins PA, PB1, and PB2 (Lamb and Krug, Reference Lamb and Krug2006; Noda et al., Reference Noda, Sagara, Yen, Takada, Kida, Cheng and Kawaoka2006). The segmented nature of the influenza virus genome is a key feature of the influenza virus structure. In the event that cells are infected with two (or more) different viruses, exchange of RNA segments between the viruses allows the generation of progeny viruses containing a novel combination of genes (‘genetic reassortment’). In theory, genetic reassortment could potentially lead to the creation of 254 new gene combinations from two parental viruses (Wright and Webster, Reference Wright and Webster2006).
Influenza viruses encode two ‘non-structural’ (NS) proteins NS1 and NS2. While the NS2 or nuclear export protein (NEP) was originally thought to be non-structural, it has since been found to be a part of the influenza virion (Richardson and Akkina, Reference Richardson and Akkina1991; Yasuda et al., Reference Yasuda, Nakada, Kato, Toyoda and Ishihama1993). In contrast, although NS1 is abundantly present in infected cells during virus replication, the protein is not incorporated into progeny virions (Wright and Webster, Reference Wright and Webster2006).
Impact of influenza A virus infections
The incidence of influenza A virus infection in the human population varies significantly from year to year and is dependent on the attack rate, virulence of the circulating strain, and on the degree of immunity of individuals in the population (Alexander and Brown, Reference Alexander and Brown2000). Nevertheless, the impact of yearly human influenza epidemics is substantial, resulting in an average of 114,000 hospitalizations, 36,000 deaths and up to $10 billion in medical costs and lost income in the United States alone (Klimov et al., Reference Klimov, Simonsen, Fukuda and Cox1999; Cox and Subbarao, Reference Cox and Subbarao2000; Bridges et al., Reference Bridges, Fukuda, Uyeki, Cox and Singleton2002a, Reference Bridges, Kuehnert and Hall2003; Thompson et al., Reference Thompson, Shay, Weintraub, Brammer, Cox, Anderson and Fukuda2003). In temperate climates, influenza epidemics typically occur in the winter months. In contrast, in the tropics the disease can occur year round (Cox and Subbarao, Reference Cox and Subbarao2000). Due to antigenic drift, the antigenicity of circulating influenza viruses is constantly changing. This allows the drift variants to infect individuals that were immune to previously circulating influenza strains (Cox and Subbarao, Reference Cox and Subbarao2000; Subbarao et al., Reference Subbarao, Swayne and Olsen2006). Therefore, the influenza viruses included in human vaccines have to be reviewed and potentially updated each year to keep pace with antigenic drift (Cox and Subbarao, Reference Cox and Subbarao2000; Subbarao et al., Reference Subbarao, Swayne and Olsen2006). Influenza surveillance is coordinated by the World Health Organization's (WHO) global influenza surveillance program.
The most dramatic consequences of influenza are associated with the periodic occurrence of influenza pandemics. Influenza pandemics are defined as global outbreaks of disease due to the emergence of viruses that contain envelope glycoproteins to which the human population is immunologically naïve (Horimoto and Kawaoka, Reference Horimoto and Kawaoka2001). In modern times, pandemics occurred in 1918 (‘Spanish flu’, H1N1), 1957 (‘Asian flu’, H2N2), 1968 (‘Hong Kong flu’, H3N2), and on a much more limited scale in 1977 (‘Russian flu’, H1N1) (Webster et al., Reference Webster, Bean, Gorman, Chambers and Kawaoka1992; Cox and Subbarao, Reference Cox and Subbarao2000; Horimoto and Kawaoka, Reference Horimoto and Kawaoka2001; Wright and Webster, Reference Wright and Webster2006). The devastation that influenza pandemics can cause was clearly demonstrated by the 1918 ‘Spanish flu’ pandemic that killed an estimated 40–50 million people worldwide (Crosby, Reference Crosby1989; Taubenberger et al., Reference Taubenberger, Reid and Fanning2000; Potter, Reference Potter2001; Taubenberger, Reference Taubenberger2003). Projections of the impact of the next influenza pandemic in the United States alone include 89,000–207,000 deaths, 314,000–734,000 hospitalizations, and up to $166 billion in direct costs (Meltzer et al., Reference Meltzer, Cox and Fukuda1999; Ferguson, Reference Ferguson2006; Layne, Reference Layne2006; Maldin and Criss, Reference Maldin and Criss2006).
While ‘fowl plague’, the disease caused by highly pathogenic avian influenza (HPAI) viruses in poultry, has been recognized since the late 18th century, the close relationship between the infectious agents causing ‘fowl plague’ and mammalian influenza was not demonstrated until 1955 (Webster et al., Reference Webster, Bean, Gorman, Chambers and Kawaoka1992; Alexander and Brown, Reference Alexander and Brown2000). HPAI viruses are restricted to H5 and H7 subtypes and clinical signs associated with infection in birds vary according to the species, age, virus strain, and environmental factors involved (Webster et al., Reference Webster, Bean, Gorman, Chambers and Kawaoka1992; Alexander and Brown, Reference Alexander and Brown2000; Swayne and Suarez, Reference Swayne and Suarez2000; Mutinelli et al., Reference Mutinelli, Capua, Terregino and Cattoli2003; Jones and Swayne, Reference Jones and Swayne2004; Ramirez et al., Reference Ramirez, Fehervari, Paasch and Calderon2005; Isoda et al., Reference Isoda, Sakoda, Kishida, Bai, Matsuda, Umemura and Kida2006). Typically, HPAI viruses are not maintained in the wild waterfowl population, but are thought to appear by introduction of H5 and H7 low-pathogenicity avian influenza viruses (LPAI) in land-based poultry and subsequent mutation to HPAI in these birds (Rohm et al., Reference Rohm, Horimoto, Kawaoka, Suss and Webster1995; Subbarao et al., Reference Subbarao, Swayne and Olsen2006). Clinical signs associated with HPAI infection may include cessation of egg laying, high fever, subcutaneous and internal hemorrhages, necrosis of the comb and wattles, edema of the head and neck, and cyanosis of the unfeathered skin (Alexander and Brown, Reference Alexander and Brown2000; Swayne and Suarez, Reference Swayne and Suarez2000; Ramirez et al., Reference Ramirez, Fehervari, Paasch and Calderon2005; Isoda et al., Reference Isoda, Sakoda, Kishida, Bai, Matsuda, Umemura and Kida2006). In contrast to LPAI viruses, which cause only mild respiratory disease and minimal to no mortality, HPAI viruses spread systemically and infection often rapidly results in death (Swayne and Suarez, Reference Swayne and Suarez2000). Therefore, outbreaks of HPAI often carry severe consequences for animal health as well as the economy of the region where they occur. For example, the outbreak of HPAI in Pennsylvania in the early 1980s resulted in 17 million culled birds, including chickens, turkeys, chukar partridges, and guinea fowl, and cost more than 60 million dollars to eradicate (Acland et al., Reference Acland, Silverman and Eckroade1984; Subbarao et al., Reference Subbarao, Swayne and Olsen2006). The outbreak of HPAI H5N1 virus in Hong Kong in 1997 resulted in the culling of 1.4 million chickens and other in-contact birds (Subbarao et al., Reference Subbarao, Swayne and Olsen2006). Lastly, hundreds of millions of domestic poultry have died or have been culled to prevent the spread of the avian H5N1 virus and the economic impact the disease has had on affected countries is estimated to exceed 10 billion dollars (Kilpatrick et al., Reference Kilpatrick, Chmura, Gibbons, Fleischer, Marra and Daszak2006). In the past, most outbreaks of HPAI were caused by a single lineage of HPAI virus. Moreover, as a result of extensive eradication programs, the virus was eliminated from the domestic bird population in less than a year (Subbarao et al., Reference Subbarao, Swayne and Olsen2006). The Asian H5N1 outbreaks appear to follow a different pattern and have been characterized by the detection of multiple reassortant viruses in domestic poultry (Guan et al., Reference Guan, Peiris, Poon, Dyrting, Ellis, Sims, Webster and Shortridge2003). Apart from the substantial socioeconomic implications of HPAI infection in poultry, transmission of H5N1 and H7N7 viruses to humans have clearly demonstrated the significant zoonotic threat these viruses pose (de Jong et al., Reference de Jong, Claas, Osterhaus, Webster and Lim1997; Claas et al., Reference Claas, Osterhaus, van Beek, De Jong, Rimmelzwaan, Senne, Krauss, Shortridge and Webster1998b; Fouchier et al., Reference Fouchier, Schneeberger, Rozendaal, Broekman, Kemink, Munster, Kuiken, Rimmelzwaan, Schutten, Van Doornum, Koch, Bosman, Koopmans and Osterhaus2004; Webster et al., Reference Webster, Guan, Poon, Krauss, Webby, Govorkovai and Peiris2005). By February 2007, the re-emergence of H5N1 HPAI in Asia and subsequent spread of the viruses to Europe and Africa, has resulted in 274 human cases, including 167 deaths (www.who.int/csr/disease/avian_influenza/en).
Although a recent study demonstrated the potential of low-pathogenic avian influenza virus to cause clinical disease in wild birds (van Gils et al., Reference van Gils, Munster, Radersma, Liefhebber, Fouchier and Klaassen2007), influenza infection in waterfowl typically is thought to result only in subclinical infection in these animals (Webster et al., Reference Webster, Yakhno, Hinshaw, Bean and Murti1978, Reference Webster, Bean, Gorman, Chambers and Kawaoka1992). The viruses preferentially replicate in the duck intestinal tract and are shed in high concentrations in the feces, thereby contaminating the lakes and ponds the birds visit (Halvorson et al., Reference Halvorson, Karunakaran, Senne, Kelleher, Bailey, Abraham, Hinshaw and Newman1983; Webster et al., Reference Webster, Bean, Gorman, Chambers and Kawaoka1992; Laver et al., Reference Laver, Bischofberger and Webster2000). As viruses of all 16 HA and nine NA subtypes are maintained in aquatic birds, particularly in migrating waterfowl, these birds represent a vast global reservoir of influenza (Halvorson et al., Reference Halvorson, Karunakaran, Senne, Kelleher, Bailey, Abraham, Hinshaw and Newman1983; Webster et al., Reference Webster, Bean, Gorman, Chambers and Kawaoka1992; Laver et al., Reference Laver, Bischofberger and Webster2000). Indeed, viruses of avian origin have been the source of outbreaks of influenza in mammals, such as seals, whales, mink, pigs, and horses (Geraci et al., Reference Geraci, St Aubin, Barker, Webster, Hinshaw, Bean, Ruhnke, Prescott, Early, Baker, Madoff and Schooley1982; Hinshaw et al., Reference Hinshaw, Bean, Webster, Rehg, Fiorelli, Early, Geraci and St Aubin1984, Reference Hinshaw, Bean, Geraci, Fiorelli, Early and Webster1986; Klingeborn et al., Reference Klingeborn, Englund, Rott, Juntti and Rockborn1985; Chambers et al., Reference Chambers, Yamnikova, Kawaoka, Lvov and Webster1989; Guo et al., Reference Guo, Wang, Kawaoka, Gorman, Ito, Saito and Webster1992; Callan et al., Reference Callan, Early, Kida and Hinshaw1995; Shortridge et al., Reference Shortridge, Chan and Guan1995; Guan et al., Reference Guan, Shortridge, Krauss, Li, Kawaoka and Webster1996; Brown et al., Reference Brown, Ludwig, Olsen, Hannoun, Scholtissek, Hinshaw, Harris, McCauley, Strong and Alexander1997; Karasin et al., Reference Karasin, Brown, Carman and Olsen2000a).
Swine influenza, first clinically recognized in pigs during the late summer and fall of 1918 (Koen, Reference Koen1919), has remained of substantial importance to the swine industry throughout the world (Webster et al., Reference Webster, Bean, Gorman, Chambers and Kawaoka1992; Alexander and Brown, Reference Alexander and Brown2000; Olsen, Reference Olsen2002). Infection of pigs can pose serious economic consequences because of the prolonged time needed for affected pigs to reach slaughter weight (Janke, Reference Janke1998). Clinical signs of influenza in pigs are similar to those observed in humans, and infections are manifested as outbreaks of acute respiratory disease characterized by fever, inactivity, decreased food intake, coughing, sneezing, and nasal discharge (McQueen et al., Reference McQueen, Steele and Robinson1968; Alexander and Brown, Reference Alexander and Brown2000; Kothalawala et al., Reference Kothalawala, Toussaint and Gruys2006; Subbarao et al., Reference Subbarao, Swayne and Olsen2006). In addition to the epizootic form of disease, influenza viruses are part of the porcine respiratory disease complex, acting in concert with other swine respiratory pathogens such as Mycoplasma hyopneumoniae, porcine reproductive and respiratory syndrome virus (PRRSV), and bacterial agents of pneumonia (Thacker et al., Reference Thacker, Thacker and Janke2001).
Since first diagnosed during an epidemic of respiratory disease in Eastern Europe in 1956 (Sovinova et al., Reference Sovinova, Tumova, Pouska and Nemec1958), outbreaks of equine influenza have occurred regularly throughout most of the world. The clinical signs observed are similar to those seen in pigs and humans and disease severity is dependent on immune status, infecting dose and virus strain (Wilson, Reference Wilson1993; Hannant and Mumford, Reference Hannant and Mumford1996). In vaccinated animals, the disease is rarely fatal, but deaths have been reported during some epidemics, particularly in donkeys (Wilson, Reference Wilson1993; Alexander and Brown, Reference Alexander and Brown2000). Severe epidemics have occurred relatively recently in India (Uppal et al., Reference Uppal, Yadav and Oberoi1989), the People's Republic of China (Guo et al., Reference Guo, Wang, Kawaoka, Gorman, Ito, Saito and Webster1992; Shortridge et al., Reference Shortridge, Chan and Guan1995), and South Africa (Guthrie et al., Reference Guthrie, Stevens and Bosman1999). In developed countries, equine influenza infections can largely be managed by vaccination and by resting of affected animals. In many other parts of the world, however, horses, donkeys and mules remain as principal working animals and influenza virus outbreaks can have severe socioeconomic impacts (Shortridge et al., Reference Shortridge, Chan and Guan1995).
Additional animal species from which influenza A viruses have been isolated include seals, mink, whales, feral and domestic cats, and dogs. In 1979–1980, harbor seals populating the northeastern coast of the United States died of respiratory disease, characterized by severe pulmonary consolidation. Influenza viruses, subtyped as H7N7, were demonstrated in the lungs and brains of the affected animals (Geraci et al., Reference Geraci, St Aubin, Barker, Webster, Hinshaw, Bean, Ruhnke, Prescott, Early, Baker, Madoff and Schooley1982). Additional isolates from seals have included H3N3 and H4N6 influenza viruses (Hinshaw et al., Reference Hinshaw, Bean, Webster, Rehg, Fiorelli, Early, Geraci and St Aubin1984; Callan et al., Reference Callan, Early, Kida and Hinshaw1995). Viruses of H13N2, H13N9, and H1N3 subtypes have been detected in the lungs of whales (Lvov et al., Reference Lvov, Zdanov, Sazonov, Braude, Vladimirtceva, Agafonova, Skljanskaja, Kaverin, Reznik, Pysina, Oserovic, Berzin, Mjasnikova, Podcernjaeva, Klimenko, Andrejev and Yakhno1978; Hinshaw et al., Reference Hinshaw, Bean, Geraci, Fiorelli, Early and Webster1986; Chambers et al., Reference Chambers, Yamnikova, Kawaoka, Lvov and Webster1989) and avian origin H10N4 viruses, causing systemic infection and disease, were isolated from farm-raised mink (Klingeborn et al., Reference Klingeborn, Englund, Rott, Juntti and Rockborn1985). Since 2004, infections of exotic and domestic cats with H5N1 HPAI viruses were documented on multiple occasions in Asia, the Middle East, and Europe (Kuiken et al., Reference Kuiken, Rimmelzwaan, van Riel, van Amerongen, Baars, Fouchier and Osterhaus2004, Reference Kuiken, Fouchier, Rimmelzwaan, Osterhaus and Roeder2006; Rimmelzwaan et al., Reference Rimmelzwaan, van Riel, Baars, Bestebroer, van Amerongen, Fouchier, Osterhaus and Kuiken2006; Songsermn et al., Reference Songsermn, Amonsin, Jam-on, Sae-Heng, Meemak, Pariyothorn, Payungporn, Theamboonlers and Poovorawan2006a; Yingst et al., Reference Yingst, Saad and Felt2006; Leschnik et al., Reference Leschnik, Weikel, Moestl, Revilla-Fernandez, Wodak, Bago, Vanek, Benetka, Hess and Thalhammer2007), of dogs in Thailand (Butler, Reference Butler2006; Songsermn et al., Reference Songsermn, Amonsin, Jam-on, Sae-Heng, Pariyothorn, Payungporn, Theamboonlers, Chutinimitkul, Thanawongnuwech and Poovorawan2006b), and of a stone marten in Germany (www.who.int/csr/con/2006_03_09a/en/index.html). Lastly, in the spring of 2004, an influenza virus was isolated from lung tissues of greyhound dogs that had died from hemorrhagic pneumonia (Crawford et al., Reference Crawford, Dubovi, Castleman, Stephenson, Gibbs, Chen, Smith, Hill, Ferro, Pompey, Bright, Medina, Group, Johnson, Olsen, Cox, Klimov, Katz and Donis2005). Sequence analysis of the viral genome revealed that the canine isolate was closely related to and had evolved from a contemporary equine H3N8 virus (Peek et al., Reference Peek, Landolt, Karasin, Slack, Steinberg, Semrad and Olsen2004; Crawford et al., Reference Crawford, Dubovi, Castleman, Stephenson, Gibbs, Chen, Smith, Hill, Ferro, Pompey, Bright, Medina, Group, Johnson, Olsen, Cox, Klimov, Katz and Donis2005). Since then, canine influenza viruses have spread across large parts of the country and appear to have established themselves in the dog population of the United States (Crawford et al., Reference Crawford, Dubovi, Castleman, Stephenson, Gibbs, Chen, Smith, Hill, Ferro, Pompey, Bright, Medina, Group, Johnson, Olsen, Cox, Klimov, Katz and Donis2005).
Influenza virus ecology
The avian reservoir
Influenza pandemics are precipitated by an ‘antigenic shift’, which describes the replacement of the predominantly circulating influenza virus subtype with a novel HA subtype, to which the human population has not recently been exposed (Fig. 3) (Webster et al., Reference Webster, Bean, Gorman, Chambers and Kawaoka1992; Wright and Webster, Reference Wright and Webster2006). This novel virus can then evade immune surveillance and replicate largely unimpeded in the immunologically naïve population. Historically, only a limited number of subtypes of influenza viruses have been associated with infection of mammals. For example, in humans only viruses of H1, H2, H3, N1, and N2 subtypes have circulated widely in the population (Webster et al., Reference Webster, Bean, Gorman, Chambers and Kawaoka1992; Alexander and Brown, Reference Alexander and Brown2000), in horses influenza infections have been largely restricted to viruses of H7N7 and H3N8 subtypes (Webster, Reference Webster1993; Wilson, Reference Wilson1993; Alexander and Brown, Reference Alexander and Brown2000), and only H1, H3, N1, and N2 subtypes have been consistently isolated from pigs (Webster et al., Reference Webster, Bean, Gorman, Chambers and Kawaoka1992; Olsen, Reference Olsen2002). The most widely accepted theory of how pandemic viruses emerge is that a virus with a novel subtype is introduced into the human population through transfer from the avian reservoir (Fig. 4), with or without genetic reassortment (Webster et al., Reference Webster, Bean, Gorman, Chambers and Kawaoka1992, Reference Webster, Wright, Castrucci, Bean and Kawaoka1993; Horimoto and Kawaoka, Reference Horimoto and Kawaoka2001; Webby and Webster, Reference Webby and Webster2001). This theory is supported by phylogenetic studies demonstrating that the ‘Spanish flu’ pandemic was caused by a virus that derived all its genes from an avian virus (Tumpey et al., Reference Tumpey, Basler, Aguilar, Zeng, Solorzano, Swayne, Cox, Katz, Taubenberger, Palese and Garcia-Sastre2005), as well as the finding that the pandemic strains of 1957 and 1968 arose from genetic reassortment of contemporary human and avian influenza viruses (Gething et al., Reference Gething, Bye, Skehel and Waterfield1980; Fang et al., Reference Fang, Min, Huylebroeck, Devos and Fiers1981; Kawaoka et al., Reference Kawaoka, Krauss and Webster1989). Several other findings highlight the importance of the avian reservoir. For example, there exists ample genetic evidence that viruses from aquatic birds were the ancestral precursors of all contemporary influenza virus lineages present in other species (Gammelin et al., Reference Gammelin, Altmuller, Reinhardt, Mandler, Harley, Hudson, Fitch and Scholtissek1990; Gorman et al., Reference Gorman, Bean, Kawaoka, Donatelli, Guo and Webster1991; Webster et al., Reference Webster, Bean, Gorman, Chambers and Kawaoka1992; Webster, Reference Webster1998). In addition, direct transmission of an avian virus to horses resulted in the severe equine influenza epidemic that occurred in the Jilin and Heilongjiang Provinces in the northeast of the People's Republic of China in 1989 (Guo et al., Reference Guo, Wang, Kawaoka, Gorman, Ito, Saito and Webster1992). Lastly, there have been several well-documented occasions on which direct avian-to-swine transmissions of viruses have occurred (see below).

Fig. 3. Schematic diagram illustrating genetic reassortment. In the event that cells are infected with two (or more) distinct influenza viruses, the exchange of RNA gene segments between viruses allows the generation of progeny viruses containing novel combinations of genes. If such a reassortment event results in the introduction of a novel HA subtype, the new virus can escape neutralizing antibodies (‘antigenic shift’).
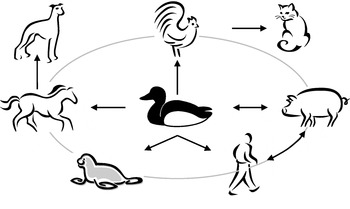
Fig. 4. Schematic representation of influenza A virus cross-species transmission. Research indicates that wild aquatic birds are the ancestral source of all influenza A viruses present in birds and mammals.
Yet, despite these examples, evidence also supports the existence of barriers that limit the transmission of influenza viruses from birds to mammals. For instance, prior to 1997 there were only three reports of human infections with avian influenza viruses (Campbell et al., Reference Campbell, Webster and Breese1970; Taylor and Turner, Reference Taylor and Turner1977; Webster et al., Reference Webster, Geraci, Petursson and Skirnisson1981). Although direct avian-to-human transmission of H5N1, H9N2, and H7N7 viruses have been described since then (de Jong et al., Reference de Jong, Claas, Osterhaus, Webster and Lim1997; Claas et al., Reference Claas, de Jong, van Beek, Rimmelzwaan and Osterhaus1998a; Lin et al., Reference Lin, Shaw, Gregory, Cameron, Lim, Klimov, Subbarao, Guan, Krauss, Shortridge, Webster, Cox and Hay2000; Bridges et al., Reference Bridges, Lim, Hu-Primmer, Sims, Fukuda, Mak, Rowe, Thompson, Conn, Lu, Cox and Katz2002b; Hatta and Kawaoka, Reference Hatta and Kawaoka2002; Uyeki et al., Reference Uyeki, Chong, Katz, Lim, Ho, Wang, Tsang, Au, Chan, Rowe, Hu-Primmer, Bell, Thompson, Bridges, Cox, Mak and Fukuda2002; Katz, Reference Katz2003; Fouchier et al., Reference Fouchier, Schneeberger, Rozendaal, Broekman, Kemink, Munster, Kuiken, Rimmelzwaan, Schutten, Van Doornum, Koch, Bosman, Koopmans and Osterhaus2004; Webster et al., Reference Webster, Guan, Poon, Krauss, Webby, Govorkovai and Peiris2005), these avian viruses still appear not to have developed the ability to transmit efficiently from person to person [examples of suspected, limited human-to-human spread of H5N1 virus notwithstanding (Gilsdorf et al., Reference Gilsdorf, Boxall, Gasimov, Agayev, Mammadzade, Ursu, Gasimov, Brown, Mardel, Jankovic, Pimentel, Ayoub, Elassal, Salvi, Legros, Pessoa da, Hay, Andraghetti, Rodier and Ganter2006; Kandun et al., Reference Kandun, Wibisono, Sedyaningsih, Yusharmen, Purba, Santoso, Septiawati, Tresnaningsih, Heriyanto, Yuwono, Harun, Soeroso, Giriputra, Blair, Jeremijenko, Kosasih, Putnam, Samaan, Silitonga, Chan, Poon, Lim, Klimov, Lindstrom, Guan, Donis, Katz, Cox, Peiris and Uyeki2006)]. Yet, the ability to transmit efficiently among humans is considered to be one of the chief prerequisites for pandemic emergence of an influenza virus (de Jong et al., Reference de Jong, Claas, Osterhaus, Webster and Lim1997; Cox and Subbarao, Reference Cox and Subbarao2000; Taubenberger and Morens, Reference Taubenberger and Morens2006).
In general, avian influenza viruses do not replicate well in humans and non-human primates, and vice versa, human viruses typically do not replicate well in birds (Hinshaw et al., Reference Hinshaw, Bean, Webster and Easterday1978, Reference Hinshaw, Webster, Naeve and Murphy1983; Webster et al., Reference Webster, Yakhno, Hinshaw, Bean and Murti1978; Murphy et al., Reference Murphy, Hinshaw, Sly, London, Hosier, Wood, Webster and Chanock1982; Snyder et al., Reference Snyder, Buckler-White, London, Tierney and Murphy1987; Beare and Webster, Reference Beare and Webster1991). Given this limited capacity for direct avian-to-human transmission, it is debatable whether the creation of pandemic viruses rests solely in either species. Rather, it is hypothesized that the emergence of an avian virus with pandemic potential requires prior adaptation in an intermediate host. Viral adaptation at the molecular level would then result in an avian-lineage virus with the ability to spread efficiently among humans (Scholtissek et al., Reference Scholtissek, Burger, Bachmann and Hannoun1983; Scholtissek and Naylor, Reference Scholtissek and Naylor1988; Scholtissek, Reference Scholtissek1990; Webster et al., Reference Webster, Bean, Gorman, Chambers and Kawaoka1992; Brown, Reference Brown2000b; Ito, Reference Ito2000).
The role of intermediate hosts in the creation of pandemic viruses
Pigs have been suggested to support two processes that can lead to the development of influenza viruses with pandemic potential: adaptation and genetic reassortment. As avian viruses of virtually all HA subtypes (H1–H13) were able to infect and replicate in pigs under experimental conditions, these animals have been postulated as the logical intermediate host in which adaptation of avian viruses may occur (Kida et al., Reference Kida, Ito, Yasuda, Shimizu, Itakura, Shortridge, Kawaoka and Webster1994). In support of these experimental results are several well-documented examples of direct avian-to-pig transmission of influenza viruses that have occurred under natural conditions. For example, in 1979 a wholly avian H1N1 influenza virus crossed the species barrier to infect pigs in Europe (Pensaert et al., Reference Pensaert, Ottis, Vandeputte, Kaplan and Bachmann1981). These avian-lineage viruses subsequently became established in the pig population throughout much of Europe (Pensaert et al., Reference Pensaert, Ottis, Vandeputte, Kaplan and Bachmann1981; Scholtissek et al., Reference Scholtissek, Burger, Bachmann and Hannoun1983; Donatelli et al., Reference Donatelli, Campitelli, Castrucci, Ruggieri, Sidoli and Oxford1991; Schultz et al., Reference Schultz, Fitch, Ludwig, Mandler and Scholtissek1991; Brown et al., Reference Brown, Ludwig, Olsen, Hannoun, Scholtissek, Hinshaw, Harris, McCauley, Strong and Alexander1997; Webby and Webster, Reference Webby and Webster2001). Other examples of in toto transmission of avian viruses to pigs include the transmission of H1N1, H3N2, H5N1, and H9N2 viruses to pigs in China and Hong Kong (Kida et al., Reference Kida, Shortridge and Webster1988; Guan et al., Reference Guan, Shortridge, Krauss, Li, Kawaoka and Webster1996; Peiris et al., Reference Peiris, Guan, Markwell, Ghose, Webster and Shortridge2001; Xu et al., Reference Xu, Fan, Wei and Zhao2004; Choi et al., Reference Choi, Nguyen, Ozaki, Webby, Puthavathana, Buranathal, Chaisingh, Auewarakul, Hanh, Ma, Hui, Guan, Peiris and Webster2005), as well as infections of pigs with H4N6, H1N1, and H3N3 viruses in Canada (Karasin et al., Reference Karasin, Brown, Carman and Olsen2000a, Reference Karasin, West, Carman and Olsen2004).
Binding of influenza virus to its cellular receptor is determined by two properties: the SA species [e.g. N-acetylneuraminic (NeuAc) or N-glycolylneuraminic (NeuGc) acid] involved, as well as the NeuAc or NeuGc linkage to galactose residues (e.g. α2,6Gal or α2,3Gal) on the cell membrane. While avian viruses prefer binding to SA with α2,3Gal linkages in NeuGc or NeuAc forms, human lineage viruses preferentially bind to NeuAcα2,6Gal-linked receptors (Suzuki, Reference Suzuki1994; Ito, Reference Ito2000; Ito and Kawaoka, Reference Ito and Kawaoka2000; Suzuki et al., Reference Suzuki, Ito, Suzuki, Holland, Chambers, Kiso, Ishida and Kawaoka2000; Varki, Reference Varki2001). This is consistent with the fact that avian intestinal cells primarily express α2,3-linked receptors, whereas human tracheal epithelial cells predominantly express α2,6-linked receptors (Rogers and Paulson, Reference Rogers and Paulson1983; Suzuki, Reference Suzuki1994; Gambaryan et al., Reference Gambaryan, Tuzikov, Piskarev, Yamnikova, Lvov, Robertson, Bovin and Matrosovich1997; Matrosovich et al., Reference Matrosovich, Gambaryan, Teneberg, Piskarev, Yamnikova, Lvov, Robertson and Karlsson1997; Vines et al., Reference Vines, Wells, Matrosovich, Castrucci, Ito and Kawaoka1998; Ito, Reference Ito2000). Yet, a study by Gambaryan and colleagues (Gambaryan et al., Reference Gambaryan, Webster and Matrosovich2002) demonstrated that SAs expressed in chickens are not exclusively of α2,3 linkage, supporting the notion that these birds could act as a potential intermediate host for the transmission of influenza viruses from aquatic birds to humans. Moreover, Wan and Perez (Reference Wan and Perez2006) found that SAs expressed in the trachea of Japanese quail are of both α2,3 and α2,6 forms. Vice versa, the SAs expressed in the human airway are also not exclusively of α2,6 linkage. While the α2,6-linked sialyloligosaccharides are the predominant receptor type expressed on the respiratory epithelial cells of the human nasal passages and trachea, a recent study by Matrosovich and colleagues (Matrosovich et al., Reference Matrosovich, Matrosovich, Gray, Roberts and Klenk2004a) indicated that a subset of human tracheal respiratory epithelial cells also express the α2,3Gal-linked SAs. Moreover, Shinya and coworkers (Shinya et al., Reference Shinya, Ebina, Yamada, Ono, Kasai and Kawaoka2006) found that non-ciliated cuboidal bronchiolar cells, as well as a substantial proportion of cells lining the alveolar walls (most likely alveolar type II cells) of the human lungs, also expressed SAα2,3Gal. The presence of SAα2,3Gal-bearing cells deep in the human respiratory tract supports the findings that viruses that have retained the avian-type receptor specificity can infect humans and cause lethal disease (Matrosovich et al., Reference Matrosovich, Zhou, Kawaoka and Webster1999).
Nevertheless, efficient transmission of avian viruses among human beings requires the HA protein to adapt to preferentially bind to the human-type SAα2,6Gal receptors (Neumann and Kawaoka, Reference Neumann and Kawaoka2006). As the respiratory epithelial cells of the porcine tracheal epithelium possess both SAα2,3Gal (NeuAc and NeuGc) as well as SAα2,6Gal receptors, pigs may serve as adaptation hosts in which the switch from avian-type to human-type receptor preference could occur (Couceiro et al., Reference Couceiro, Paulson and Baum1993; Suzuki et al., Reference Suzuki, Horiike, Yamazaki, Kawabe, Masuda, Miyamoto, Matsuda, Nishimura, Yamagata, Ito, Kida, Kawaoka and Suzuki1997; Ito et al., Reference Ito, Couceiro, Kelm, Baum, Krauss, Castrucci, Donatelli, Kida, Paulson, Webster and Kawaoka1998; Ito, Reference Ito2000). For instance, an avian virus may initially infect pigs by employing SAα2,3Gal receptors. With continued replication in pigs, the virus may then adapt its receptor specificity to NeuAcα2,6Gal, thus providing a potential link from birds to humans (Ito et al., Reference Ito, Couceiro, Kelm, Baum, Krauss, Castrucci, Donatelli, Kida, Paulson, Webster and Kawaoka1998; Ito, Reference Ito2000). This scenario is particularly attractive in light of the fact that adaptation of the HA receptor-binding preference was observed after introduction of the avian H1N1 influenza virus into pigs in Europe in 1979 (Rogers and D'Souza, Reference Rogers and D'Souza1989; Ito, Reference Ito2000). Also, the subsequent recovery of these viruses from human patients in The Netherlands (Rimmelzwaan et al., Reference Rimmelzwaan, de Jong, Bestebroer, van Loon, Claas, Fouchier and Osterhaus2001) confirmed that these viruses had also become infectious for human beings.
Secondly, since pigs are also susceptible to infection with human lineage viruses (Kundin, Reference Kundin1970; Shortridge et al., Reference Shortridge, Webster, Butterfield and Campbell1977; Hinshaw et al., Reference Hinshaw, Bean, Webster and Easterday1978; Nakajima et al., Reference Nakajima, Nakajima, Shortridge and Kendal1982; Ottis et al., Reference Ottis, Sidoli, Bachmann, Webster and Kaplan1982; Mancini et al., Reference Mancini, Donatelli, Rozera, Arangio and Butto1985; Bean et al., Reference Bean, Schell, Katz, Kawaoka, Naeve, Gorman and Webster1992; Shu et al., Reference Shu, Lin, Wright, Shortridge and Webster1994; Bikour et al., Reference Bikour, Frost, Deslandes, Talbot, Weber and Elazhary1995; Brown et al., Reference Brown, Harris and Alexander1995; Nerome et al., Reference Nerome, Kanegae, Shortridge, Sugita and Ishida1995; Karasin et al., Reference Karasin, Schutten, Cooper, Smith, Subbarao, Anderson, Carman and Olsen2000c; Song et al., Reference Song, Lee, Oh, Lyoo, Yoon, Park and Park2003), these animals may serve as ‘mixing vessel’ hosts for genetic reassortment between human and avian viruses. According to this hypothesis, if two or more distinct influenza viruses co-infect a pig, the viruses can exchange RNA segments during replication, which can lead to the creation of new virus variants (Scholtissek, Reference Scholtissek1990). While there is no direct evidence that the reassortment events leading to the 1957 or 1968 pandemic viruses occurred in pigs, genetic reassortment between human-like H3N2 and avian-like H1N1 viruses has happened more recently among pigs in Europe (Castrucci et al., Reference Castrucci, Donatelli, Sidoli, Barigazzi, Kawaoka and Webster1993). The resulting reassortant viruses contained mammalian HA and NA surface glycoproteins, while maintaining the avian internal genes (Castrucci et al., Reference Castrucci, Donatelli, Sidoli, Barigazzi, Kawaoka and Webster1993; Brown et al., Reference Brown, Harris, McCauley and Alexander1998). And importantly, reassortant H3N2 and H1N2 viruses were subsequently also isolated from humans in Europe and Hong Kong (Claas et al., Reference Claas, Kawaoka, de Jong, Masurel and Webster1994; Gregory et al., Reference Gregory, Lim, Cameron, Bennett, Marozin, Klimov, Hall, Cox, Hay and Lin2001, Reference Gregory, Bennett, Orkhan, Al Hajjar, Varsano, Mendelson, Zambon, Ellis, Hay and Lin2002). Additional support for the ‘mixing vessel’ theory comes from the demonstration of 2-way (human/swine) and 3-way (avian/human/swine) reassortant viruses of H3N2, H1N2, H1N1, and H3N1 subtypes that have emerged in pigs since 1998 (Zhou et al., Reference Zhou, Senne, Landgraf, Swenson, Erickson, Rossow, Liu, Yoon, Krauss and Webster1999a; Karasin et al., Reference Karasin, Olsen and Anderson2000b, Reference Karasin, Schutten, Cooper, Smith, Subbarao, Anderson, Carman and Olsenc, Reference Karasin, Landgraf, Swenson, Erickson, Goyal, Woodruff, Scherba, Anderson and Olsen2002, Reference Karasin, Carman and Olsen2006; Webby et al., Reference Webby, Swenson, Krauss, Gerrish, Goyal and Webster2000, Reference Webby, Rossow, Erickson, Sims and Webster2004; Choi et al., Reference Choi, Goyal, Farnham and Joo2002; Song et al., Reference Song, Lee, Oh, Lyoo, Yoon, Park and Park2003; Lekcharoensuk et al., Reference Lekcharoensuk, Lager, Vemulapalli, Woodruff, Vincent and Richt2006; Ma et al., Reference Ma, Gramer, Rossow and Yoon2006; Olsen et al., Reference Olsen, Karasin, Carman, Li, Bastien, Ojkic, Alves, Charbonneau, Henning, Low, Burton and Broukhanski2006). In the United States, these reassortant viruses have subsequently spread widely within the country's swine population. However, equally important to note is the fact that persistent circulation of human influenza viruses in swine populations, though likely to facilitate the development of a pandemic virus in pigs through genetic reassortment, has occurred relatively rarely (Hinshaw et al., Reference Hinshaw, Bean, Webster and Easterday1978; Easterday, Reference Easterday1980; Ito, Reference Ito2000). Consequently, it has been suggested that, as with maintenance of avian influenza viruses in the swine population, efficient infection of pigs with human influenza viruses may require mutational adaptation of the virus to the new swine host (Brown, Reference Brown2000b; Lipatov et al., Reference Lipatov, Govorkova, Webby, Ozaki, Peiris, Guan, Poon and Webster2004). In support of this notion are recent data demonstrating limited infectivity of a human virus following experimental infection of pigs (Landolt et al., Reference Landolt, Karasin, Phillips and Olsen2003, Reference Landolt, Karasin, Schutten and Olsen2006).
For many years pigs have been considered the leading candidate for the intermediate host for avian-to-mammalian influenza virus adaptation; however, recent data suggest that terrestrial poultry, such as quail, chickens, and turkeys, may also play a central role in the emergence of viruses with pandemic potential. Surveillance in live bird markets in China, as well as experimental infection studies, demonstrated that land-based birds support replication of a variety of subtypes of avian influenza viruses (Liu et al., Reference Liu, Guan, Peiris, He, Webby, Perez and Webster2003a, Reference Liu, He, Walker, Zhou, Perez, Mo, Li, Huang, Webster and Webbyb; Perez et al., Reference Perez, Webby, Hoffmann and Webster2003). More importantly, H5N1 and H9N2 viruses isolated from land-based poultry were found to have lower affinity for SAα2,3Gal than their respective counterparts isolated from aquatic birds (Matrosovich et al., Reference Matrosovich, Zhou, Kawaoka and Webster1999, Reference Matrosovich, Krauss and Webster2001; Saito et al., Reference Saito, Lim, Suzuki, Suzuki, Kida, Nishimura and Tashiro2001), suggesting that land-based poultry may serve as adaptation hosts for the conversion of SAα2,3Gal to SAα2,6Gal receptor preference (Matrosovich et al., Reference Matrosovich, Krauss and Webster2001; Perez et al., Reference Perez, Webby, Hoffmann and Webster2003; Li et al., Reference Li, Guan, Wang, Smith, Xu, Duan, Rahardjo, Puthavathana, Buranathai, Nguyen, Estoepangestie, Chaisingh, Auewarakul, Long, Hanh, Webby, Poon, Chen, Shortridge, Yuen, Webster and Peiris2004). This is consistent with the fact that both SAα2,3Gal and SAα2,6Gal receptors are expressed in trachea of these birds (Gambaryan et al., Reference Gambaryan, Webster and Matrosovich2002; Wan and Perez, Reference Wan and Perez2006). The potential significance of terrestrial poultry as intermediate hosts is further highlighted by the finding that H7N3 viruses circulating since 2002 in the turkey population of Northern Italy were closely related to H7N3 strains isolated from wild ducks in 2001 (Capua et al., Reference Capua, Marangon, Cordioli, Bonfanti and Santucci2002; Abe et al., Reference Abe, Takashita, Sugawara, Matsuzaki, Muraki and Hongo2004; Campitelli et al., Reference Campitelli, Mogavero, De Marco, Delogu, Puzelli, Frezza, Facchini, Chiapponi, Foni, Cordioli, Webby, Barigazzi, Webster and Donatelli2004). Serological studies conducted in human beings with close contact with infected turkeys indicated that zoonotic transmission of the H7N3 viruses had also occurred (Puzelli et al., Reference Puzelli, Di Trani, Fabiani, Campitelli, De Marco, Capua, Aguilera, Zambon and Donatelli2005). Yet, despite these examples, recent data demonstrated that human and swine lineage influenza viruses were unable to replicate efficiently in terrestrial birds (Makarova et al., Reference Makarova, Ozaki, Kida, Webster and Perez2003). Therefore, it appears that pigs remain the most likely domestic animal species in which genetic reassortment between avian and human viruses may occur.
Direct transmission between mammalian species
While the previous examples clearly illustrate the importance of the avian reservoir as a source of novel virus strains, influenza viruses of different genotypes and subtypes occasionally also can transmit between two mammalian species. The appearance of the ‘Spanish flu’ virus in 1918 might have involved this mechanism. Sequence data of the 1918 strain (Belshe, Reference Belshe2005; Tumpey et al., Reference Tumpey, Basler, Aguilar, Zeng, Solorzano, Swayne, Cox, Katz, Taubenberger, Palese and Garcia-Sastre2005), as well as seroepidemiological studies of survivors of the 1918 pandemic, demonstrate that the pandemic 1918 H1N1 virus and the earliest swine H1N1 viruses were very closely related (Taubenberger et al., Reference Taubenberger, Reid and Fanning2000; Taubenberger and Morens, Reference Taubenberger and Morens2006). More recently, direct swine-to-human zoonotic transmission of influenza viruses has been documented on several occasions (Alexander and Brown, Reference Alexander and Brown2000; Myers et al., Reference Myers, Olsen, Setterquist, Capuano, Donham, Thacker, Merchant and Gray2006), including in North America (Hinshaw et al., Reference Hinshaw, Bean, Webster and Easterday1978; Dacso et al., Reference Dacso, Couch, Six, Young, Quarles and Kasel1984; Patriarca et al., Reference Patriarca, Kendal, Zakowski, Cox, Trautman, Cherry, Auerbach, McCusker, Belliveau and Kappus1984; Rota et al., Reference Rota, Rocha, Harmon, Hinshaw, Sheerar, Kawaoka, Cox and Smith1989; Wentworth et al., Reference Wentworth, Thompson, Xu, Regnery, Cooley, McGregor, Cox and Hinshaw1994, Reference Wentworth, McGregor, Macklin, Neumann and Hinshaw1997; Kimura et al., Reference Kimura, Adlakha and Simon1998; Gaydos et al., Reference Gaydos, Top, Hodder and Russell2006; Olsen et al., Reference Olsen, Karasin, Carman, Li, Bastien, Ojkic, Alves, Charbonneau, Henning, Low, Burton and Broukhanski2006), Europe (Claas et al., Reference Claas, Kawaoka, de Jong, Masurel and Webster1994; Rimmelzwaan et al., Reference Rimmelzwaan, de Jong, Bestebroer, van Loon, Claas, Fouchier and Osterhaus2001; Gregory et al., Reference Gregory, Bennett, Thomas, Kaiser, Wunderli, Matter, Hay and Lin2003), and Asia (Gregory et al., Reference Gregory, Lim, Cameron, Bennett, Marozin, Klimov, Hall, Cox, Hay and Lin2001). Furthermore, serologic evidence collected from pig farm workers indicates that zoonotic infection may occur more often than the number of virus isolation reports suggests (Schnurrenberger et al., Reference Schnurrenberger, Woods and Martin1970; Campitelli et al., Reference Campitelli, Donatelli, Foni, Castrucci, Fabiani, Kawaoka, Krauss and Webster1997; Olsen et al., Reference Olsen, Brammer, Easterday, Arden, Belay, Baker and Cox2002; Ayora-Talavera et al., Reference Ayora-Talavera, Cadavieco-Burgos and Canul-Armas2005).
Apart from swine-to-human transmission of viruses, there exist only a handful of reports documenting zoonotic transmission of viruses involving other mammalian species. For instance, experimental infection of human volunteers with H3 equine-lineage viruses produced influenza-like illnesses associated with virus shedding and subsequent seroconversion (Couch et al., Reference Couch, Douglas, Giggs, Knight and Kasel1969; Kasel and Couch, Reference Kasel and Couch1969). Conversely, occasional human-to-equine transmission of H1N1, H2N2, and H3N2 viruses has been reported (Tumova, Reference Tumova1980; Heilman and La Montagne, Reference Heilman and La Montagne1990) and experimental infection of horses with human H3N2 viruses demonstrated their susceptibility to infection with human viruses (Kasel and Couch, Reference Kasel and Couch1969). However, there is no evidence that horse-to-human or human-to-horse transmission routinely occurs under natural conditions. Lastly, data from serosurveillance and experimental challenge studies indicate that human-lineage viruses occasionally cross the species barrier to infect dogs (Ado and Titova, Reference Ado and Titova1959; Nikitin et al., Reference Nikitin, Cohen, Todd and Lief1972; Paniker and Nair, Reference Paniker and Nair1972; Bibrack, Reference Bibrack1975; Bibrack et al., Reference Bibrack, Ackermann and Benary1975; Chang et al., Reference Chang, New, Taylor and Chiang1976; Houser and Heuschele, Reference Houser and Heuschele1980). However, while these results indicate that dogs are susceptible to infection with human influenza viruses, infection did not result in clinical disease (Todd and Cohen, Reference Todd and Cohen1968; Bibrack, Reference Bibrack1975; Bibrack et al., Reference Bibrack, Ackermann and Benary1975; Chang et al., Reference Chang, New, Taylor and Chiang1976; Houser and Heuschele, Reference Houser and Heuschele1980) and these viruses did not spread efficiently among dogs (Nikitin et al., Reference Nikitin, Cohen, Todd and Lief1972). In contrast, recent infections of dogs with an equine H3N8 virus have been associated with clinical signs of respiratory illness (including fatal hemorrhagic pneumonias), and recovery of virus from dogs from across the country, as well as serological evidence, indicate the spread and apparent maintenance of the virus within the canine population of the United States (Crawford et al., Reference Crawford, Dubovi, Castleman, Stephenson, Gibbs, Chen, Smith, Hill, Ferro, Pompey, Bright, Medina, Group, Johnson, Olsen, Cox, Klimov, Katz and Donis2005).
Molecular determinants of species specificity
As the preceding paragraphs demonstrate, cross-species transmissions of influenza A viruses occur relatively frequently. Yet, in many instances, these transmission events tend to be self-limiting and the newly introduced viruses are only rarely maintained in the new host species (Webster et al., Reference Webster, Bean, Gorman, Chambers and Kawaoka1992). It has long been recognized that influenza A viruses exhibit partial restriction of their host range. Moreover, a number of subtypes of influenza viruses are rarely detected in animals other than their typical host (Webster et al., Reference Webster, Bean, Gorman, Chambers and Kawaoka1992; Webby and Webster, Reference Webby and Webster2001), suggesting that specific subtypes differ in their ability to cross the species barrier (Brown, Reference Brown2000a). While the viral and host factors that determine influenza virus host range are only incompletely understood, evidence has accumulated over the years indicating potential contributions by all eight gene segments (Scholtissek et al., Reference Scholtissek, Burger, Kistner and Shortridge1985; Tian et al., Reference Tian, Buckler-White, London, Reck, Chanock and Murphy1985; Snyder et al., Reference Snyder, Buckler-White, London, Tierney and Murphy1987, Reference Snyder, London, Maassab, Chanock and Murphy1990; Murphy et al., Reference Murphy, Buckler-White, London and Snyder1989; Webster et al., Reference Webster, Bean, Gorman, Chambers and Kawaoka1992; Castrucci and Kawaoka, Reference Castrucci and Kawaoka1993; Subbarao et al., Reference Subbarao, London and Murphy1993; Horimoto and Kawaoka, Reference Horimoto and Kawaoka2001; Hatta et al., Reference Hatta, Halfmann, Wells and Kawaoka2002; Li et al., Reference Li, Chen, Jiao, Deng, Tian, Li, Hoffmann, Webster, Matsuoka and Yu2005; Dalton et al., Reference Dalton, Mullin, Amorim, Medcalf, Tiley and Digard2006; Neumann and Kawaoka, Reference Neumann and Kawaoka2006). Examination of the contributions of individual viral proteins to host range restriction is complicated by a number of factors. For one, mutations often appear not in just one, but in multiple gene segments during the process of virus adaptation to a new host species. For example, sequence analysis of six human H5N1 isolates revealed that the viruses had acquired a variety of amino acid substitutions, affecting not only their HA, but also the internal proteins (PB2, PB1, PA, NP, M, and NS) (Suarez et al., Reference Suarez, Perdue, Cox, Rowe, Bender, Huang and Swayne1998; Bender et al., Reference Bender, Hall, Huang, Klimov, Cox, Hay, Gregory, Cameron, Lim and Subbarao1999; Hiromoto et al., Reference Hiromoto, Yamazaki, Fukushima, Saito, Lindstrom, Omoe, Nerome, Lim, Sugita and Nerome2000). As some of the substitutions in NP, PB2, and M2 occurred in sites previously defined as potential species-specific signatures of human versus avian H5N1 isolates, these mutations may indeed reflect adaptation of the virus to the new host species (Zhou et al., Reference Zhou, Shortridge, Claas, Krauss and Webster1999b; Hiromoto et al., Reference Hiromoto, Yamazaki, Fukushima, Saito, Lindstrom, Omoe, Nerome, Lim, Sugita and Nerome2000). However, some of the other substitutions could also have been introduced in response to host immunological pressure or they may simply represent spurious mutations (Zhou et al., Reference Zhou, Shortridge, Claas, Krauss and Webster1999b; Hiromoto et al., Reference Hiromoto, Yamazaki, Fukushima, Saito, Lindstrom, Omoe, Nerome, Lim, Sugita and Nerome2000). To complicate matters further, specific constellations of gene segments may be involved in controlling influenza virus species specificity. This notion is supported by the finding that during the reassortment events that led to the creation of the 1957 and the 1968 pandemic strains, both viruses acquired the avian HA and PB1 genes (as well as the avian NA in 1957) (Kawaoka et al., Reference Kawaoka, Krauss and Webster1989). Yet, despite such complexities, several genes appear to play dominant roles in controlling influenza host range, and the following paragraphs will review the current knowledge of the molecular determinants of influenza host range.
HA
Due to its role as the viral receptor-binding protein, many investigators have focused their attention on the HA as the primary determinant of host range, and over the years a large body of evidence has accumulated indicating that the HA is, in fact, a key player in influenza virus species specificity (Chambers et al., Reference Chambers, Yamnikova, Kawaoka, Lvov and Webster1989; Aytay and Schulze, Reference Aytay and Schulze1991; Inkster et al., Reference Inkster, Hinshaw and Schulze1993; Vines et al., Reference Vines, Wells, Matrosovich, Castrucci, Ito and Kawaoka1998; Bender et al., Reference Bender, Hall, Huang, Klimov, Cox, Hay, Gregory, Cameron, Lim and Subbarao1999; Ito et al., Reference Ito, Kawaoka, Nomura and Otsuki1999; Suzuki et al., Reference Suzuki, Ito, Suzuki, Holland, Chambers, Kiso, Ishida and Kawaoka2000; Hatta et al., Reference Hatta, Halfmann, Wells and Kawaoka2002; Romanova et al., Reference Romanova, Katinger, Ferko, Voglauer, Mochalova, Bovin, Lim, Katinger and Egorov2003; Medeiros et al., Reference Medeiros, Naffakh, Manuguerra and van der Werf2004). As previously discussed, avian influenza viruses bind preferentially to SAα2,3Gal, while human lineage influenza viruses prefer α2,6-linked SA receptors. Analysis of the three-dimensional structure of the H3 HA from human influenza viruses has revealed that the binding site that accommodates the SA receptor is a shallow pocket, formed by amino acid residues that are fairly highly conserved among virtually all subtypes and strains of influenza A viruses (Wright and Webster, Reference Wright and Webster2006). Research has demonstrated that subtle differences in the amino acid residues that form the binding site can result in alterations of the receptor-binding properties of the HA molecule. For example, analysis of the HA gene of the 1968 pandemic strain by Bean and coworkers (Bean et al., Reference Bean, Schell, Katz, Kawaoka, Naeve, Gorman and Webster1992) revealed that fewer than six amino acid residues in the HA of the 1968 Hong Kong pandemic virus were altered in the process of avian-to-human transmission. All of the mutations occurred in the globular head portion of the HA and included amino acid substitutions at positions 62, 144, 193, and 226 (Bean et al., Reference Bean, Schell, Katz, Kawaoka, Naeve, Gorman and Webster1992). The mutation affecting residue 226 was found to be of particular interest in regard to receptor-binding specificity. Leucine (Leu)-226 was found to confer SAα2,6Gal specificity in human H2 and H3, but not H1, viruses (Matrosovich et al., Reference Matrosovich, Tuzikov, Bovin, Gambaryan, Klimov, Castrucci, Donatelli and Kawaoka2000; Skehel and Wiley, Reference Skehel and Wiley2000), whereas glutamine (Gln) at position 226 correlates with SAα2,3Gal preference in avian and equine H3 viruses (Naeve et al., Reference Naeve, Hinshaw and Webster1984; Vines et al., Reference Vines, Wells, Matrosovich, Castrucci, Ito and Kawaoka1998). With the exception of a few early isolates, human viruses with Leu at position 226 typically contain serine (Ser) at residue 228, while glycine (Gly)-228 is associated with Gln-226 in avian viruses (Naeve et al., Reference Naeve, Hinshaw and Webster1984; Vines et al., Reference Vines, Wells, Matrosovich, Castrucci, Ito and Kawaoka1998). While there exists less evidence for its role in receptor specificity compared to residue 226, in most human H3 viruses, Ser at residue 193 is associated with SAα2,6Gal specificity, while asparagine (Asn) or lysine (Lys)-193 is associated with SAα2,3Gal specificity in avian and equine H3 viruses (Medeiros et al., Reference Medeiros, Naffakh, Manuguerra and van der Werf2004). Aspartic acid (Asp)-190 was found to determine SAα2,6Gal specificity in human and swine H1 isolates, whereas glutamic acid (Glu)-190 correlates with the avian-type receptor-binding preference (Gammelin et al., Reference Gammelin, Altmuller, Reinhardt, Mandler, Harley, Hudson, Fitch and Scholtissek1990; Kobasa et al., Reference Kobasa, Takada, Shinya, Hatta, Halfmann, Theriault, Suzuki, Nishimura, Mitamura, Sugaya, Usui, Murata, Maeda, Watanabe, Suresh, Suzuki, Suzuki, Feldmann and Kawaoka2004; Stevens et al., Reference Stevens, Corper, Basler, Taubenberger, Palese and Wilson2004). Finally, both single and combined mutations of the amino acid residues at position 182 (Asn to Lys) or position 192 (Gln to arginine) converted the receptor-binding specificity of avian H5N1 viruses to the human-type SAα2,6Gal receptor specificity (Yamada et al., Reference Yamada, Suzuki, Suzuki, Le, Nidom, Sakai-Tagawa, Muramoto, Ito, Kiso, Horimoto, Shinya, Sawada, Kiso, Usui, Murata, Lin, Hay, Haire, Stevens, Russell, Gamblin, Skehel and Kawaoka2006).
In addition to the amino acid sequence of the HA molecule, receptor-binding specificity is also influenced by the number and position of N-linked oligosaccharides at or around the receptor-binding site (Deom et al., Reference Deom, Caton and Schulze1986; Aytay and Schulze, Reference Aytay and Schulze1991; Gunther et al., Reference Gunther, Glatthaar, Doller and Garten1993; Inkster et al., Reference Inkster, Hinshaw and Schulze1993; Matrosovich et al., Reference Matrosovich, Gambaryan, Teneberg, Piskarev, Yamnikova, Lvov, Robertson and Karlsson1997; Gambaryan et al., Reference Gambaryan, Marinina, Tuzikov, Bovin, Rudneva, Sinitsyn, Shilov and Matrosovich1998; Baigent and McCauley, Reference Baigent and McCauley2001; Banks and Plowright, Reference Banks and Plowright2003; Abe et al., Reference Abe, Takashita, Sugawara, Matsuzaki, Muraki and Hongo2004). Variation of glycosylation around the receptor-binding site can often be observed following adaptation of a virus to growth in a new host species or cell line. For example, adaptation of influenza viruses to growth in eggs (Robertson et al., Reference Robertson, Nicolson, Major, Robertson and Wood1993; Banks and Plowright, Reference Banks and Plowright2003; Romanova et al., Reference Romanova, Katinger, Ferko, Voglauer, Mochalova, Bovin, Lim, Katinger and Egorov2003), in mice (Gitelman et al., Reference Gitelman, Kaverin, Kharitonenkov, Rudneva, Sklyanskaya and Zhdanov1986), or in mammalian cell lines (Crecelius et al., Reference Crecelius, Deom and Schulze1984; Gunther et al., Reference Gunther, Glatthaar, Doller and Garten1993; Robertson et al., Reference Robertson, Cook, Attwell and Williams1995; Romanova et al., Reference Romanova, Katinger, Ferko, Voglauer, Mochalova, Bovin, Lim, Katinger and Egorov2003), resulted in alterations in glycosylation patterns. Furthermore, glycosylation patterns of the HA may be directly associated with species specificity. This is illustrated by the fact that a glycosylation site at position 63, commonly found in H3 viruses of human origin, is absent in avian-lineage H3 viruses (Kida et al., Reference Kida, Shortridge and Webster1988). Moreover, research demonstrated that adaptation of the H1N1 human strain A/USSR/90/77 to mice resulted in the loss of glycosylation sites at either position 131 (Asn to Asp) alone or in combination with position 94 (Thr to Ala). Growth of the virus in a mammalian cell line [Madin Darby canine kidney (MDCK) cells] led to the selection of variants with a single mutation at position 131 (Asn to Asp), indicating that the carbohydrate group attached to Asn-131 may affect host range (Gitelman et al., Reference Gitelman, Kaverin, Kharitonenkov, Rudneva, Sklyanskaya and Zhdanov1986; Gambaryan et al., Reference Gambaryan, Marinina, Tuzikov, Bovin, Rudneva, Sinitsyn, Shilov and Matrosovich1998).
Competitive inhibitors, such as soluble receptor analogs that are present in the serum of many species, may also play a role in influenza virus host range restriction (Ryan-Poirier and Kawaoka, Reference Ryan-Poirier and Kawaoka1991). For example, α2-macroglobulins present in horse and guinea pig serum were shown to strongly inhibit hemagglutination as well as infection of MDCK cells by human H3 viruses with SAα2,6Gal receptor specificity, but did not affect equine and avian H3 virus infection (Rogers et al., Reference Rogers, Pritchett, Lane and Paulson1983). Similarly, recent studies indicate that SA residues on porcine surfactant protein D (pSP-D) may also influence host range, possibly by acting as natural inhibitors of influenza virus binding to cell-surface SA receptors (Hartshorn et al., Reference Hartshorn, White, Voelker, Coburn, Zaner and Crouch2000; van Eijk et al., Reference van Eijk, van de Lest, Batenburg, Vaandrager, Meschi, Hartshorn, van Golde and Haagsman2002; Hawgood et al., Reference Hawgood, Brown, Edmondson, Stumbaugh, Allen, Goerke, Clark and Poulain2004). While it remains unclear if and to what extent pSP-D contributes to host range, it has been speculated that pSP-D interference may play a particularly important role in human-to-swine transmission of influenza viruses (van Eijk et al., Reference van Eijk, van de Lest, Batenburg, Vaandrager, Meschi, Hartshorn, van Golde and Haagsman2002).
NA
Like HA, the NA also contributes to influenza virus species specificity. Since efficient growth of influenza virus is dependent on balanced action between HA receptor-binding affinity and NA receptor-destroying activity (Baum and Paulson, Reference Baum and Paulson1991; Rudneva et al., Reference Rudneva, Kovaleva, Varich, Farashyan, Gubareva, Yamnikova, Popova, Presnova and Kaverin1993; Gubareva et al., Reference Gubareva, Bethell, Hart, Murti, Penn and Webster1996, Reference Gubareva, Nedyalkova, Novikov, Murti, Hoffmann and Hayden2002; McKimm-Breschkin et al., Reference McKimm-Breschkin, Blick, Sahasrabudhe, Tiong, Marshall, Hart, Bethell and Penn1996; Kaverin et al., Reference Kaverin, Gambaryan, Bovin, Rudneva, Shilov, Khodova, Varich, Sinitsin, Makarova and Kropotkina1998, Reference Kaverin, Matrosovich, Gambaryan, Rudneva, Shilov, Varich, Makarova, Kropotkina and Sinitsin2000; Baigent et al., Reference Baigent, Bethell and McCauley1999; Kaverin and Klenk, Reference Kaverin and Klenk1999; Hughes et al., Reference Hughes, Matrosovich, Rodgers, McGregor and Kawaoka2000, Reference Hughes, McGregor, Suzuki, Suzuki and Kawaoka2001; Mitnaul et al., Reference Mitnaul, Matrosovich, Castrucci, Tuzikov, Bovin, Kobasa and Kawaoka2000; Wagner et al., Reference Wagner, Wolff, Herwig, Pleschka and Klenk2000; Hatta et al., Reference Hatta, Gao, Halfmann and Kawaoka2001; Abed et al., Reference Abed, Bourgault, Fenton, Morley, Gower, Owens, Tisdale and Boivin2002), alterations in HA receptor-binding preference is often associated with changes in the NA's SA substrate specificity (i.e. cleavage activity of SAα2,3Gal versus SAα2,6Gal) and cleavage activity (Baum and Paulson, Reference Baum and Paulson1991; Kaverin et al., Reference Kaverin, Gambaryan, Bovin, Rudneva, Shilov, Khodova, Varich, Sinitsin, Makarova and Kropotkina1998, Reference Kaverin, Matrosovich, Gambaryan, Rudneva, Shilov, Varich, Makarova, Kropotkina and Sinitsin2000; Mitnaul et al., Reference Mitnaul, Matrosovich, Castrucci, Tuzikov, Bovin, Kobasa and Kawaoka2000; Wagner et al., Reference Wagner, Wolff, Herwig, Pleschka and Klenk2000). This conclusion is supported by the finding that after introduction of the 1957 pandemic strain into the human population, the SAα2,6Gal cleavage activity of the avian NA increased (Baum and Paulson, Reference Baum and Paulson1991; Kobasa et al., Reference Kobasa, Kodihalli, Luo, Castrucci, Donatelli, Suzuki, Suzuki and Kawaoka1999), which suggests that the NA had adapted to the SAα2,6Gal preference of the virus's HA (Neumann and Kawaoka, Reference Neumann and Kawaoka2006). Interestingly, at this point the virus also appeared to lose its ability to efficiently grow in ducks (Kobasa et al., Reference Kobasa, Wells and Kawaoka2001).
The NA molecule is comprised of the enzymatically active head domain and a stalk region that is inserted into the viral envelope (Lamb and Krug, Reference Lamb and Krug2006). The length and amino acid sequence of the stalk region vary considerably among different viruses (Blok and Air, Reference Blok and Air1982) and stalk length has been shown to affect growth characteristics of viruses in embryonated chicken eggs (Castrucci and Kawaoka, Reference Castrucci and Kawaoka1993), cell culture (Luo et al., Reference Luo, Chung and Palese1993), and mice (Castrucci and Kawaoka, Reference Castrucci and Kawaoka1993). Viruses with long stalks grew to higher titers in embryonated chicken eggs than those with shorter NA stalks (Castrucci and Kawaoka, Reference Castrucci and Kawaoka1993). Mechanistically, this may be explained by the finding that viruses with a shortened NA stalk typically are released less efficiently from the cell since the enzymatic site in the head domain cannot effectively reach its substrate (Castrucci and Kawaoka, Reference Castrucci and Kawaoka1993; Luo et al., Reference Luo, Chung and Palese1993; Baigent et al., Reference Baigent, Bethell and McCauley1999; Giannecchini et al., Reference Giannecchini, Campitelli, Calzoletti, De Marco, Azzi and Donatelli2006). Furthermore, a deletion in the NA stalk is commonly associated with adaptation of duck viruses to land-based poultry such as turkeys and chickens (Castrucci and Kawaoka, Reference Castrucci and Kawaoka1993; Matrosovich et al., Reference Matrosovich, Zhou, Kawaoka and Webster1999; Wagner et al., Reference Wagner, Wolff, Herwig, Pleschka and Klenk2000; Gambaryan et al., Reference Gambaryan, Webster and Matrosovich2002) and is thought to occur in order to counterbalance changes in the receptor-binding properties of the HA (Baigent and McCauley, Reference Baigent and McCauley2001). Nevertheless, viruses with short stalks can maintain their virulence in humans and poultry. For example, the HPAI H5N1 viruses isolated from land-based poultry in Asia contained a shortened stalk (Li et al., Reference Li, Guan, Wang, Smith, Xu, Duan, Rahardjo, Puthavathana, Buranathai, Nguyen, Estoepangestie, Chaisingh, Auewarakul, Long, Hanh, Webby, Poon, Chen, Shortridge, Yuen, Webster and Peiris2004). Similarly, H5N1 viruses isolated from human patients in Hong Kong in 1997 possessed short NA stalks (Matrosovich et al., Reference Matrosovich, Zhou, Kawaoka and Webster1999).
Polymerase complex
The largest body of evidence regarding the effects of the proteins in the polymerase complex on species specificity has implicated the PB2 gene. For example, Clements and coworkers (Clements et al., Reference Clements, Subbarao, Fries, Karron, London and Murphy1992) reported that a human–avian reassortant virus that contained only the avian PB2 gene replicated efficiently in avian cells, but inefficiently in mammalian cells and in the respiratory tract of squirrel monkeys and human volunteers. Subsequently, research revealed that the switch in host range hinged on a single amino acid residue at position 627 (Glu in avian isolates; Lys in human isolates) (Subbarao et al., Reference Subbarao, London and Murphy1993). Penn and coworkers identified additional amino acid residues in PB2 as potential contributors to host range (Penn, Reference Penn1989). The influenza polymerase complex constitutes a multifunctional enzyme and the outcome of its interaction with the viral RNA is modulated by whether the 3′ and 5′ termini of the viral template are in a base-paired or single-stranded conformation (Lee et al., Reference Lee, Klumpp, Digard and Tiley2003). Thus, it has long been hypothesized that temperature at the site of replication could impact the function of the polymerase complex and thereby determine the host tropism of influenza virus (McCauley and Penn, Reference McCauley and Penn1990; Baigent and McCauley, Reference Baigent and McCauley2003). This scenario is particularly appealing in light of the finding that viral polymerase complexes derived from avian viruses, but not human viruses, exhibited cold sensitivity in mammalian cells, a characteristic that was mostly controlled by residue 627 of PB2 (Massin et al., Reference Massin, van der Werf and Naffakh2001). The importance of residue 627 in the control of influenza host range is further highlighted by the findings that an H7N7 HPAI isolated from a patient in The Netherlands contained a Lys substitution at position 627 (Fouchier et al., Reference Fouchier, Schneeberger, Rozendaal, Broekman, Kemink, Munster, Kuiken, Rimmelzwaan, Schutten, Van Doornum, Koch, Bosman, Koopmans and Osterhaus2004) and several of the H5N1 strains isolated from humans in Asia were also characterized by Lys at residue 627 (Puthavathana et al., Reference Puthavathana, Auewarakul, Charoenying, Sangsiriwut, Pooruk, Boonnak, Khanyok, Thawachsupa, Kijphati and Sawanpanyalert2005). In addition, a recent study by Mase and colleagues revealed that viruses isolated from mice infected with H5N1 (without prior adaptation to mice) all acquired the Glu-to-Lys substitutions at position 627 of the PB2 (Mase et al., Reference Mase, Tanimura, Imada, Okamatsu, Tsukamoto and Yamaguchi2006). Finally, H5N1 isolates recovered from six tigers in 2004 also contained Lys at residue 627 (Amonsin et al., Reference Amonsin, Payungporn, Theamboonlers, Thanawongnuwech, Suradhat, Pariyothorn, Tantilertcharoen, Damrongwantanapokin, Buranathai, Chaisingh, Songserm and Poovorawan2006). Taken together, these observations suggest that the mutation at residue 627 may be a key determinant of influenza host range. Interestingly, studies by Hatta and coworkers indicated that the same Gly-to-Lys substitution at position 627 in the PB2 protein also influenced pathogenicity as well as cell tropism of a highly pathogenic H5N1 virus isolated in Hong Kong in 1997 (Hatta et al., Reference Hatta, Gao, Halfmann and Kawaoka2001).
Effects on host range have also been identified for the remaining two members of the polymerase complex: PA and PB1. For instance, the introduction of an avian PB1 into a human virus resulted in a decrease in replication efficiency in MDCK cells, as well as in squirrel monkeys, but did not affect virus replication in chicken kidney cells (Snyder et al., Reference Snyder, Buckler-White, London, Tierney and Murphy1987).
Apart from temperature sensitivity, amino acid substitutions in the polymerase proteins may influence host range by altering interactions of the polymerase complex with host cell factors. Host factors that were found to modulate viral RNA synthesis in vitro include RNA polymerase activating factors (RAF1 and 2), polymerase release factor (PRF), and RNA polymerase inhibiting factor 1 (RIF1) (Honda and Ishihama, Reference Honda and Ishihama1997).
NP, M, and NS proteins
During virus replication, the influenza NP is modified by host-cell-derived phosphokinases (Lamb and Krug, Reference Lamb and Krug2006). As the phosphorylation pattern of the NP protein appears to determine the extent to which a particular cell line supports virus growth (Kistner et al., Reference Kistner, Muller, Becht and Scholtissek1985), it has been hypothesized that NP is a significant determinant of species specificity (Scholtissek et al., Reference Scholtissek, Burger, Kistner and Shortridge1985; Tian et al., Reference Tian, Buckler-White, London, Reck, Chanock and Murphy1985; Snyder et al., Reference Snyder, Buckler-White, London, Tierney and Murphy1987; Abe et al., Reference Abe, Takashita, Sugawara, Matsuzaki, Muraki and Hongo2004). Experiments using temperature-sensitive mutants of a HPAI H7N1 virus (A/FPV/Rostock/1/34) demonstrated that, with the exception of NP and HA, all remaining gene segments of the fowl plague virus could be replaced by corresponding genes of any strain, irrespective of the lineage of the rescuing virus (Scholtissek et al., Reference Scholtissek, Koennecke and Rott1978, Reference Scholtissek, Burger, Kistner and Shortridge1985). Furthermore, sequence analyses suggest that the NP gene has evolved into five distinct, host-specific lineages: two equine lineages, one pig and human lineage, one aquatic bird lineage, and one lineage in other avian species (Webster, Reference Webster1997).
The influenza M1 protein plays an essential role in viral assembly and has a variety of functions, including association with influenza virus RNP complexes. The M2 is an integral membrane protein and functions as an ion channel (Pinto et al., Reference Pinto, Holsinger and Lamb1992; Holsinger et al., Reference Holsinger, Nichani, Pinto and Lamb1994; Lamb and Krug, Reference Lamb and Krug2006). Employing human–avian reassortant viruses containing the avian M and NP genes, both gene segments were found to be associated with reduced replication of the reassortants in the respiratory tract of squirrel monkeys (Tian et al., Reference Tian, Buckler-White, London, Reck, Chanock and Murphy1985). Further evidence for the M gene's contribution to influenza host range stems from co-infection experiments selecting for reassortant viruses with the human virus M gene and the HA gene derived from the avian virus. While the M segment of an earlier human virus was found to support efficient growth of the reassortants, M genes derived from more recent human strains did not cooperate with the avian HA (Scholtissek et al., Reference Scholtissek, Stech, Krauss and Webster2002).
Like the polymerase complex, the internal proteins may exert their effects on host range through interactions with cytoplasmic and nuclear host cell components (Brown, Reference Brown2000a). For example, during infection, the influenza NP binds to actin, as well as karyopherins 1 and 2. Similarly, NS1 interacts with a number of cellular factors involved in mRNA processing [i.e. Cpsf, poly(A)-binding protein II and PKR] (Lamb and Krug, Reference Lamb and Krug2006), as well as other host factors with undefined function (NS1-BP and NS1-1) (Wolff et al., Reference Wolff, O'Neill and Palese1996, Reference Wolff, O'Neill and Palese1998). Thus, it is conceivable that the quality of these protein-to-protein interactions may have an impact on influenza species specificity. However, to what extent such protein–protein interactions determine host range has yet to be determined.
Concluding remarks
In the past decade, a number of viruses have emerged from animal populations. These include HIV (Levy et al., Reference Levy, Hoffman, Kramer, Landis, Shimabukuro and Oshiro1984; Wain-Hobson et al., Reference Wain-Hobson, Vartanian, Henry, Chenciner, Cheynier, Delassus, Martins, Sala, Nugeyre, Guetard, Klatzmann, Gluckman, Rozenbaum, Barré-Sinoussi and Montagnier1991), hendravirus (Murray et al., Reference Murray, Selleck, Hooper, Hyatt, Gleeson, Westbury, Hiley, Selvey, Rodwell and Ketterer1995), and the coronavirus causing severe acute pulmonary syndrome (SARS) (Kuiken et al., Reference Kuiken, Fouchier, Schutten, Rimmelzwaan, van Amerongen, van Riel, Laman, de Jong, van Doornum, Lim, Ling, Chan, Tam, Zambon, Gopal, Drosten, van der Werf, Escriou, Manuguerra, Stohr, Peiris and Osterhaus2003). In addition to these newly recognized viruses, re-emerging viruses are burdening the human population at a seem-ingly increasing frequency (Morse, Reference Morse1997). One of our most familiar viruses, influenza A virus, also falls into this category. The recent resurgence of H5N1 influenza A viruses in poultry, wild waterfowl, cats and people throughout large parts of Asia, the Middle East, Europe, and Africa further highlights the possibility that viruses originating in an animal species could spark a new influenza pandemic (de Jong et al., Reference de Jong, Claas, Osterhaus, Webster and Lim1997; Cox and Subbarao, Reference Cox and Subbarao2000; Laver et al., Reference Laver, Bischofberger and Webster2000; Horimoto and Kawaoka, Reference Horimoto and Kawaoka2001; Hatta and Kawaoka, Reference Hatta and Kawaoka2002; Katz, Reference Katz2003; Belshe, Reference Belshe2005; Webster et al., Reference Webster, Guan, Poon, Krauss, Webby, Govorkovai and Peiris2005; Ferguson, Reference Ferguson2006; Kenny, Reference Kenny2006; Maldin and Criss, Reference Maldin and Criss2006). An important reason why these viruses have not yet caused a full-blown pandemic is their apparent inability to spread efficiently from person to person (Hatta and Kawaoka, Reference Hatta and Kawaoka2002; Katz, Reference Katz2003; Capua and Alexander, Reference Capua and Alexander2004; Webster et al., Reference Webster, Guan, Poon, Krauss, Webby, Govorkovai and Peiris2005; Kandun et al., Reference Kandun, Wibisono, Sedyaningsih, Yusharmen, Purba, Santoso, Septiawati, Tresnaningsih, Heriyanto, Yuwono, Harun, Soeroso, Giriputra, Blair, Jeremijenko, Kosasih, Putnam, Samaan, Silitonga, Chan, Poon, Lim, Klimov, Lindstrom, Guan, Donis, Katz, Cox, Peiris and Uyeki2006; Shinya et al., Reference Shinya, Ebina, Yamada, Ono, Kasai and Kawaoka2006). While there has been an explosion of data on the molecular determinants of influenza virus adaptation to a new host species within the last decade, much remains to be learned. In light of the importance of animal reservoirs in the ecology of influenza as well as the potential roles of pigs and terrestrial poultry as adaptation or ‘mixing vessel’ hosts, virus surveillance at the human–animal interface and genetic analysis of animal influenza viruses must remain a priority. The data collected will continue to provide important insights regarding the genetic basis of host adaptation and advance our understanding of the extent and impact of animal reservoirs of influenza A viruses.