Introduction
Over the recent decade, scientists have been interested in searching for clues to the origin and evolution of life on Earth by simulating the primary environment of our planet (Miller Reference Miller1953; Ponnamperuma & Mack Reference Ponnamperuma and Mack1965; Huber & Wächtershäuser Reference Huber and Wächtershäuser1998; Früh-Green et al. Reference Früh-Green, Kelley, Bernasconi, Karson, Ludwig, Butterfield, Boschi and Proskurowski2003; Foustoukos & Seyfried Reference Foustoukos and Seyfried2004). The synthesis of prebiotic organic compounds (Shock Reference Shock1990, Reference Shock1992; Simoneit Reference Simoneit1992) and amino acids (Shock Reference Shock1990; Islam et al. Reference Islam, Kaneko and Kobayashi2003) under conditions mimicking the primitive environment have attracted special attention. In 1953, Miller's milestone experiment reported an abiotic synthesis of amino acids from a mixture of moist reducing gases (CH4, NH3 and H2) using electric discharge under simulated primitive atmosphere conditions (Miller Reference Miller1953). Since then, numerous studies have been reported to generate amino acids by Strecker (Hennet et al. Reference Hennet, Holm and Engel1992; Cody et al. Reference Cody, Boctor, Hazen, Brandes, Morowitz and Yoder2001), gas-phase reactions (Miller Reference Miller1953; Plankensteiner et al. Reference Plankensteiner, Reiner, Schranz and Rode2004) using simple organic (CH4, HCHO , CH3CHO and HCN) and inorganic (CO, CO2, NH3, H2 and H2O) molecules which may have existed on our planet a long time ago. However, no experimental evidence indicates that amino acids can be synthesized directly from carboxylic acid and ammonia under submarine hydrothermal conditions. In our previous studies, we were able to employ ammonia to synthesize glycine and alanine from acetic acid and propionic acid, respectively. Here, we report the first abiotic synthesis of amino acids performed under hydrothermal conditions, which highly mimic hypothesized conditions of the ocean floor in primitive Earth environment.
Since the discovery of submarine hydrothermal springs by Corliss et al. in the late 1970s (Corliss et al. Reference Corliss1979), it has been proposed that deep-sea hydrothermal systems were plausible sites for the origin and evolution of life (Corliss et al. Reference Corliss, Baross and Hoffman1981). The primordial sea-floor vents encompass a wide range of closely linked gradients in physical and chemical conditions, including temperature (2–450 °C), pressure (0.1–25 MPa), pH, redox potential and metal/non-metal environments (Baross & Hoffman Reference Baross and Hoffman1985). These gradients and associated mineralization provided necessary multiple pathways for the origin of life, from the abiotic synthesis of chemical precursors to the formation of simple bio-molecules such as amino acids and proteinoids, even to the evolution of free-living organisms. A general concern about submarine hydrothermal systems as possible geochemical environments for non-biological synthesis lies in the stability of amino acids, peptides and proteins at high temperatures (Bernhardt et al. Reference Bernhardt, Lüdemann and Jaenicke1984; White Reference White1984; Andersson & Holm Reference Andersson and Holm2000). Amino acids are predicted to be thermodynamically stable in the cool regions of submarine hydrothermal systems, however, unstable at the centre of hydrothermal vents with a high temperature of about 350 °C (Shock Reference Shock1992). The hypothesis is that the reactions associated with chemical precursors to organic compounds of biological importance occurred at the centre of hydrothermal vent. Later, these organic compounds were delivered to lower-temperature areas by circulating fluids across temperature gradients, which were conducive to the formation of amino acids. Therefore, when succinic acid was formed in high-temperature zones, it flowed into cooler zones to form amino acids.
Among these investigations, extensive attention has been focused on the possibility of catalytic activity of nature minerals containing transition metal Fe-bearing minerals (Fe–O, Fe–S–O and Fe–Si–O), which may exert catalytic activity and specificity for prebiotic synthesis of organic compounds and amino acids (Huebner Reference Huebner and Ulmer1971; Chou Reference Chou, Ulmer and Barnes1987; Fein et al. Reference Fein, Hemley, D'Angelo, Komninou and Sverjensky1992). Therefore, it is reasonable that magnetite can potentially act as an effective mineral catalyst for abiotic formation of amino acids from succinic acid and ammonia in hydrothermal systems.
It has been suggested that thermal energy sources were readily available for prebiotic synthesis of amino acids and complex organic compounds as evidenced by the widespread occurrence of regions of elevated temperatures on Earth (Fox & Windsor Reference Fox and Windsor1970; Lawless & Boynton Reference Lawless and Boynton1973). In this study, microwave-assisted heating was used as a more effective heating method, which would shorten exhaustive long reaction from hours to a few minutes (Kremsner & Kappe Reference Kremsner and Kappe2005).
Succinic acid, which could readily exist in submarine hot springs (Ruiz-Bermejo et al. Reference Ruiz-Bermejo, Menor-Salván, Osuna-Esteban and Veintemillas-Verdaguer2007), was employed as the starting material to construct carbon backbone. The amine group was installed by direct reaction of succinic acid and ammonia under the experimental conditions described above (Scheme 1). Then, a possible route for origin of amino acids is further proposed and discussed based on characterization and analysis of amino acid products.
Scheme 1. Succinic acid reacted with ammonia yielding aspartic acid and glycine in the presence of magnetite catalysts and microwave irradiation.
Materials and methods
All chemical reagents were of analytical grade. All aqueous solutions were prepared with ultrapure water (18 MΩ cm) produced in a water purification system. Ammonia and succinic acid were purchased from the Fifth Reagent Company of Shengyang. Magnetite was obtained from Tianjin Jinfeng Chemical Co., Ltd.
In a vial, 3 mL aqueous solution of reactants (succinic acid 0.002 M, ammonia 0.002 M) was prepared. After adding Fe3O4 as the catalytic transition metal, the solution was purged by bubbling high-purity nitrogen gas for 5 minutes. The vial was capped and then heated in a microwave reactor at 90–180 °CFootnote 1 for 10, 20 or 30 minutes. The solution was cooled down to room temperature and collected for further analysis.
Pre-column derivatization methods were employed to determine the yields of products by reverse-phase HPLC on an Agilent 1100 series HPLC system with UV detection. For amino acid analysis, in a 1 mL vial, 300 μL of 0.5M NaHCO3 (pH 9) and 50 μL of 5% 2, 4-dinitrofluorobenzene/acetonitrile were successively added to 50 μL of a sample solution. After being mixed adequately, the specimen was heated in darkness to 60 °C for 30 minutes. When the sample cooled down to room temperature, 400 μL of 0.1 M KH2PO4 buffer (pH 7) was added to terminate the derivatization reaction. Identification and quantification of amino acids were performed by comparing the retention times of pure reference substances in a series of concentrations. Gradient elution was performed by using the following eluents. Solvent A consisted of acetonitrile and ultrapure water (CH3CN:H2O=55 : 45). Solvent B was a solution of KH2PO4 buffered at pH 7.2. The samples (2 μl) were injected into an Agilent ODS column (5 μm, 150 mm×4.6 μm). Column temperature was maintained at 30 °C. The flow rate was 1 ml minute−1. Amino acids eluting from the column were detected with a UV detector at 360 nm.
Results
Small amounts of aspartic acid and glycine were found in HPLC analysis (Fig. 1b). The analytical uncertainties were within±0.5%. UV spectra of the products were compared with those of the 15 amino acid standards (Fig. 1a). The reaction at 180 °C, 10 minutes (Fig. 1b) shows peaks at retention times of 15.22 and 7.77 minutes corresponding to glycine (41.12 μmol L−1) and aspartic acid (25.25 μmol L−1), respectively. The simplest α-amino acid glycine was probably a decomposition product from the more complex amino acid, aspartic acid. It should be noted that 13 other possible amino acids with retention times from 7.7 to 19.6 minutes were not detected.
Fig. 1. Chromatograms of amino acids formed in experiment. (a) High performance liquid chromatography (HPLC) chromatogram showing 15 amino acid standards namely aspartic acid (Asp), glutamine (Glu), serine (Ser), histidine (His), arginine (Arg), glycine (Gly), threonine (Thr), proline (Pro), alanine (Ala), valine (Val), cystine (Cys), methionine (Met), isoleucine (Ile), leucine (Leu) and phenylalanine (Phe). (b). HPLC chromatogram showing the amino acids glycine and aspartic acid in experimental fluid after reaction at 180 °C, 10 minutes.
Discussion
The HPLC data of retention time of reactions after 30 minute heating at different temperatures were compared in Table 1. No obvious product was observed with heating up to 90 °C. Nevertheless, a trace amount of aspartic acid was observed when the reaction temperature was raised to 120 °C. As expected, the amount of aspartic acid increased with the temperature above 120 °C, and reached a maximum at 180 °C (upper limit of the instrument) eventually. Glycine showed a similar temperature trend to aspartic acid.
Table 1. Amino acids obtained by succinic acid-ammonia-type synthesis when the reactants were heated for 30 minutes
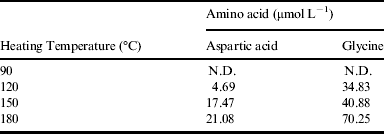
N.D.: not detected.
Time dependence of the aspartic acid reaction was studied at different temperatures (Table 2). The yield of aspartic acid increases upon a longer reaction time at relatively low temperatures (120 °C and 150 °C). A possible explanation is that the direct formation of aspartic acid from ammonia and succinic acid is highly energy demanding. Relatively long time is required for producing an appreciable amount of products at a relatively low reaction rate. At high temperature (180 °C), a large amount of aspartic acid could be formed within only 10 minutes, which can be attributed to the high reaction rate at 180 °C. However, the amount of aspartic acid starts to decline at reaction time of 30 minutes or longer. This may be explained by possible further decomposition reactions. Aspartic acid, a hydrophilic amino acid, could easily decompose under harsh conditions. Heating at high temperatures for long time without protection makes amino acids as structurally complicated as aspartic acid vulnerable for decomposition or oligomerization. Similar side reactions have been observed in other amino acid syntheses at about 200 °C (Yanagawa & Kojima Reference Yanagawa and Kojima1985; Marshall Reference Marshall1994). It is known that different amino acids have different decomposition temperatures and the decomposition temperatures of structurally complex amino acids are usually lower than those of simpler ones. Therefore, for simple amino acid glycine, the decomposition temperature is higher than that of aspartic acid. This explains why no obvious decomposition reaction was observed for glycine at 180 °C. The yield of glycine only increases with longer reaction time (Table 3).
Table 2. Yields of aspartic acid (μmol L−1) in the reaction with different reaction times at 120, 150 and 180 °C

Table 3. Yields of glycine (μmol L−1) in the reaction with different reaction times at 120, 150 and 180 °C

Further studies of the yield of glycine and aspartic acid at 180 °C at different times indicate different formation trends of aspartic acid and glycine. Namely, the yield of aspartic acid increases, while the yield of glycine decreases (Table 4). This further supports our hypothesis that the structural complexity of amino acid undermines the yield in a high-temperature reaction. It also suggests that submarine hot spring, as a complicated geographical environment with various temperature gradients, may play a crucial role in the origin of life by providing a unique reaction environment for amino acid evolution in various stages. First, the environment immediately around the submarine hot springs had the highest temperature, which encouraged the formation of simple organic compounds from simple inorganic compounds. Spreading with water flow, these basic organic compounds were delivered to low-temperature environment to form amino acids and other biological macromolecules like proteins. The time that amino acids stayed on each temperature gradient was varied by seawater flowing speed. Therefore, multifarious submarine hot springs could have ‘designed’ specific environments with different reaction temperatures and time for every individual amino acid, which ensured high diversity of amino acids, and further diversity of species in a larger picture.
Table 4. Amino acids obtained by succinic acid-ammonia-type synthesis when the reactants were heated at 180 °C for 10, 20 and 30 minutes

In many previous studies, high starting concentrations of reactants were employed to synthesize amino acids (Schulte & Shock Reference Schulte and Shock1995; Horita & Berndt Reference Horita and Berndt1999). Miller et al. suggested that the synthesis of amino acids under simulated natural hydrothermal conditions should use diluted solution of reducing compounds such as CH3CHO, NH3 and HCN from theoretical calculation (Miller & Van Trump Reference Miller, Van Trump and Wolman1981). Our study shows that even very dilute starting materials, i.d. 0.002 M succinic acid and 0.002 M ammonia, can still generate amino acids. When higher concentrations of starting materials were used in our study, the percent yield based on carbon was almost of the same magnitude.
Many attempts have been made to investigate the catalysed reaction of inorganic forms of carbon to form simple organic molecules on mineral surfaces (Cody et al. Reference Cody, Boctor, Hazen, Brandes, Morowitz and Yoder2001). The inorganic mineral catalyst magnetite was collected, and then characterized by XRD analysis. The result shows that magnetite have been changed to be haematite which is also existing in submarine systems (Fig. 2). This shift fits coincidently with the process of ancient sedimentary transformation from magnetite to haematite. It is suggested that the abiotic synthesis of amino acids on magnetite surfaces might be a catalytic process involving adsorption, exchange and electron transfer. Magnetite is abundant on Earth and a major component of all the planetary bodies in the inner solar system. It would be oxidized to haematite in primordial sea floor vent systems. Catalysts consisting of magnetite were effective in hydrocarbon synthesis. The oxidation of magnetite may actually increase its activity (Szatmari Reference Szatmari1989). Alternatively, the reactions generating amino acids take place on the corroded surface of magnetite particles, where the magnetite acts as a redox buffer catalyzing C–H amination of carboxylic acid substrates to give corresponding amino acids (Scheme 2). Interchange between Fe3O4 and Fe2O3 effectively activates the α-C–H bond in succinic acid, which is then inserted by ammonia to give glycine and aspartic acid. In another scenario, the catalytic cycle could be mediated by the electrochemical redox couple on the surface of the magnetite particles. Fe2O3–Fe3O4 surface adsorb the ionic forms of ammonia and succinic acids (NH4+ and C3H7COO−), resulting in an increment of local concentration, such that electrons transfer could happen on the corroded magnetite surface effectively. Ammonia, therefore, could carry out a nucleophilic attack on activated α-hydrogen of succinic acid to form simple amino acids, aspartic acid and glycine.

Fig. 2. XRD pattern of solid sample obtained from reaction at 180 °C, 10 minutes.
Scheme 2. Buffer reaction of magnetite and haematite assemblages.
Conclusion
For the first time, aspartic acid, representing a four carbon backbone-amino acid, has been investigated in the reaction of carboxylic acid and ammonia. Aspartic acid and glycine were synthesized from succinic acid and ammonia under hydrothermal conditions in the presence of magnetite. Among the parameters investigated, time and temperature significantly affected the yield of aspartic acid and glycine. Reaction time has opposite effects on the yield of aspartic acid and glycine due to their difference in complexities. Rather than a limitation, temperature dependency should be seen as a possible cause for diversity of amino acids and furthermore diversity of species. This proves again the important role that hot submarine springs played in the origin of life.
Acknowledgements
This work was supported by the National Sciences Foundation of China (No. 21271082) and the Key International Science and Technology Foundation of Hainan Province (No. 2012-GH004).
Disclosure Statement
The authors have nothing to disclose. No competing financial interests exist.