1. Introduction
Granitoids are the major components of upper continental crust and have been central to models of the formation, emergence, evolution and destruction of continental crust, as well as the history of supercontinents (Brown, Reference Brown2013; Spencer et al. Reference Spencer, Roberts and Santosh2017; Roberts & Santosh, Reference Roberts and Santosh2018; Cawood & Hawkesworth, Reference Cawood and Hawkesworth2019; Hawkesworth et al. Reference Hawkesworth, Cawood and Dhuime2019; Wang & Santosh, Reference Wang and Santosh2019). Granitoids are also widely used for petrogenetic modelling to understand deep crustal and crust–mantle interaction processes, including geodynamic and tectonic processes (Zhu et al. Reference Zhu, Lai, Qin and Zhao2018a,b,c).
This study focuses on the granites from the Tengchong Block, which is located in the southwestern part of the Sanjiang Tethys tectonic domain, and corresponds to the southern extension of the Lhasa Block, which is a region that has been the focus of investigations related to the Neo-Tethyan subduction and the India–Asia collision (Fig. 1a) (Zhao et al. Reference Zhao, Lai, Qin, Zhu and Wang2017b; Zhu et al. Reference Zhu, Lai, Santosh, Qin and Zhao2017b, Reference Zhu, Lai, Qin and Zhao2018b). The large granitoids in the Tengchong Block define three N–S-trending belts from the Early Cretaceous eastern Donghe to Late Cretaceous central Guyong and Palaeogene western Binglangjiang granites (Fig. 1b) (Cao et al. Reference Cao, Zou, Zhang, Zhang, Zheng, Zhang, Tang and Pei2016, Reference Cao, Zhang, Santosh, Zhang, Tang, Pei and Yang2018; Fang et al. Reference Fang, Zhang, Zhang, Cao, Zou and Dong2018). There are also abundant synchronous tin deposits, such as the Xiaolonghe and Lailishan in the Tengchong Block (Cao et al. Reference Cao, Zhang, Santosh, Zhang, Tang, Pei and Yang2018). As the largest tin deposit in the Tengchong Block, the Xiaolonghe tin deposit located in the Guyong granite belt has been involved in several recent studies, which were mainly focused on the ore-forming processes and fluid evolution (Chen et al. Reference Chen, Zhao, Zhang and Yang2017, Reference Chen, Zhao, Zhu, Wang and Cui2018; Cui et al. Reference Cui, Wang, Deng, Wu and Shu2019), the geochronology of the deposit and related granites (Chen et al. Reference Chen, Hu, Bi, Li, Lan, Zhao and Zhu2014; Zhang et al. Reference Zhang, Zhang, Cao, Wu, Xiao, Chen and Tang2014; Cao et al. Reference Cao, Zou, Zhang, Zhang, Zheng, Zhang, Tang and Pei2016) and the genetic link between the metallogeny and magmatism (Cao et al. Reference Cao, Zou, Zhang, Zhang, Zheng, Zhang, Tang and Pei2016). Although the Xiaolonghe granites were considered to be related to the tin mineralization based on their geochronological and stable isotopic (C–H–O–S–Pb–He–Ar) features (Cao et al. Reference Cao, Zou, Zhang, Zhang, Zheng, Zhang, Tang and Pei2016; Chen et al. Reference Chen, Zhao, Zhu, Wang and Cui2018), and the geochronology and geochemical evolution of the Guyong porphyritic biotite granites were well studied (Xu et al. Reference Xu, Yang, Lan, Luo, Huang, Shi and Xie2012; Xie et al. Reference Xie, Zhu, Dong, Zhao, Wang and Mo2016; Zhao et al. Reference Zhao, Lai, Qin, Zhu and Wang2017b), a systematic study of the relationship and detailed magmatic process of these different granites in the Guyong granite belt is still lacking. In addition, the question of whether the Xiaolonghe medium-grained granite or Xiaolonghe fine-grained syenogranite is related to the mineralization remains equivocal. Debate also surrounds the tectonic setting in which the Guyong and Xiaolonghe granites formed because of the complex tectonic evolution of the region (Yang et al. Reference Yang, Xu, Huang, Luo and Shi2009; Jiang et al. Reference Jiang, Gong, Zhang and Ma2012; Xu et al. Reference Xu, Yang, Lan, Luo, Huang, Shi and Xie2012; Ma et al. Reference Ma, Deng, Wang, Wang, Zhang and Li2013; Zhang et al. Reference Zhang, Jin, Fan, Zhang, Shen, Gao and Cheng2013; Chen, H. J. et al. Reference Chen, Hu, Bi, Zhong, Lan, Zhao and Zhu2015; Chen, X. C. et al. Reference Chen, Zhang, Cao, Wang, Nie, Zhang and Tang2015; Qi et al. Reference Qi, Zhu, Grimmer and Hu2015; Cao et al. Reference Cao, Zou, Zhang, Zhang, Zheng, Zhang, Tang and Pei2016; Zhao et al. Reference Zhao, Lai, Qin, Zhu and Wang2017b).

Fig. 1. (a) Geotectonic map of the Tengchong Block (Cao et al. Reference Cao, Zhang, Lin, Zheng, Wu and Li2014). (b) The distribution of granitoid rocks in the Tengchong Block (Chen et al. Reference Chen, Hu, Bi, Li, Lan, Zhao and Zhu2014). (c) Geological sketch of the Xiaolonghe tin deposit (Sang et al. Reference Sang, Xia, Zhao and Jiang2015). Abbreviations: JSSZ – Jinsha suture zone; BNSZ – Bangong Nujiang suture zone; IYMSZ – Indus Yarlung Myitkyina suture zone; QTB – Qiangtang Block; NLSB – Northern Lhasa Block; CLSB – Central Lhasa Block; SLSB – Southern Lhasa Block; TCB – Tengchong Block; BSB – Baoshan Block; BNMB – Bangong Nujiang metallogenic belt; GDMB – Gangdese metallogenic belt; YLMB – Yulong metallogenic belt; 1 – glutenite; 2 – metasandstone and slate of Carboniferous Menghong group; 3 – Xiaolonghe fine-grained syenogranite; 4 – Xiaolonghe medium-grained syenogranite; 5 – Guyong porphyritic monzogranite; 6 – cassiterite and greisen; 7 – geological boundary; 8 – granite facies boundary.
In this study, we perform an integrated petrological, geochemical and isotopic investigation of the Guyong and Xiaolonghe intrusions, including zircon U–Pb ages, Hf–O data and whole-rock major and trace elements, and we determine Sr–Nd isotope composition. In conjunction with information from previous studies, we attempt to (1) identify the ore-forming rocks and understand the genesis of the Xiaolonghe tin deposit; (2) evaluate the petrogenesis and relationship of the Guyong and Xiaolonghe granites, as well as the relative role of mantle, lower crust and upper crust in the generation of the Guyong and Xiaolonghe granites; and (3) provide insights into the evolution of the Neo-Tethys system and the related geodynamic history of the region.
2. Geological setting
2.a. Regional geology
The Sanjiang Tethys belt located in SW China is composed of several micro-continental blocks progressively younging from east to west, such as the Baoshan and Tengchong blocks (Liu et al. Reference Liu, Hu, Gao, Feng, Huang, Lai, Yuan, Liu, Coulson, Feng, Wang and Qi2009; Shi et al. Reference Shi, Wu, Kröner, Fan and Yang2015), which are separated by the Lushui–Luxi–Ruili fault (Zhu et al. Reference Zhu, Lai, Qin and Zhao2015). The Tengchong Block, a northern fragment of the Sibumasu (Wang et al. Reference Wang, Zhang, Cawood, Ma, Fan, Zhang, Zhang and Bi2014), is located in the southeastern part of the Tethyan tectonic zone (Fig. 1a), and it tectonically belongs to the suture zone of the Indian Plate and Eurasia. The subduction of the Meso-Tethys and the formation of the Neo-Tethys was induced by the northward movement of the Lhasa and west Myanmar regions during Late Triassic – Late Jurassic times (Chen et al. Reference Chen, Zhao, Zhu, Wang and Cui2018). The closure of the Meso-Tethys, which formed the Bangong–Nujiang suture (Chen et al. Reference Chen, Zhao, Zhu, Wang and Cui2018), occurred during Late Jurassic and Early Cretaceous times, and the closure of the Neo-Tethys occurred after Late Cretaceous times along the Indus Yarlung Myitkyina suture zone (Xu et al. Reference Xu, Yang, Lan, Luo, Huang, Shi and Xie2012; Shi et al. Reference Shi, Wu, Kröner, Fan and Yang2015; Chen et al. Reference Chen, Zhao, Zhu, Wang and Cui2018; Zhu et al. Reference Zhu, Lai, Santosh, Qin and Zhao2017b; Fang et al. Reference Fang, Zhang, Zhang, Cao, Zou and Dong2018). The timing of the initial collision of the Indo-Asian continents is still uncertain and ranges from 65 Ma to 34 Ma (Chen et al. Reference Chen, Zhao, Zhu, Wang and Cui2018). The successive subduction of the Meso- and Neo-Tethyan oceans resulted in a series of magmatic activities (Fig. 1b) in the Tengchong Block (Fang et al. Reference Fang, Zhang, Zhang, Cao, Zou and Dong2018).
The northward subduction of the Neo-Tethyan oceanic crust beneath the Eurasian continental crust and subsequent collision between the Indian and Eurasian plates resulted in large-scale Mesozoic–Cenozoic magmatism and related mineralization, termed the Tethyan metallogenic belt (Cao et al. Reference Cao, Zhang, Lin, Zheng, Wu and Li2014; Chen et al. Reference Chen, Zhao, Zhu, Wang and Cui2018; Fang et al. Reference Fang, Zhang, Zhang, Cao, Zou and Dong2018). The Sanjiang Tethys tectonic domain defines the junction between Eurasia, the South China Block, India and Sunda. In addition, it is located in the southeastern Tethys tectonic domains (Fig. 1a) and is composed of several continental blocks (Liu et al. Reference Liu, Hu, Gao, Feng, Huang, Lai, Yuan, Liu, Coulson, Feng, Wang and Qi2009). Granites and related ore deposits are widespread in the Sanjiang Tethys tectonic domains and form part of the Tethyan metallogenic domain. As the northernmost extension of the world-class SE Asian tin belt and the most important tin belt of the Sanjiang Tethyan Metallogenic Domain, the Tengchong–Lianghe tin belt hosts abundant granites and spatio-temporally related tin deposits (Fig. 1b; Cao et al. Reference Cao, Zhang, Lin, Zheng, Wu and Li2014; Chen, X. C. et al. Reference Chen, Hu, Bi, Zhong, Lan, Zhao and Zhu2015).
Magmatism in the Tengchong Block can be divided into three stages as follows: (1) The Early Cretaceous Donghe granite belt in the eastern Tengchong Block. These granites are mostly S-type granites with ages between 131 and 114 Ma, and they are enriched in large ion lithophile elements (LILEs) and depleted in high field strength elements (HFSEs) (Deng et al. Reference Deng, Wang, Li, Li and Wang2014; Xie et al. Reference Xie, Zhu, Dong, Zhao, Wang and Mo2016). The zircon εHf(t) isotopic values of these granites reveal the increasing contributions of mantle or juvenile crustal components, and they were induced by subduction of the Meso-Tethys and collision between the Tengchong and Baoshan blocks in the eastern Tengchong Block (Qi et al. Reference Qi, Zhu, Grimmer and Hu2015; Xie et al. Reference Xie, Zhu, Dong, Zhao, Wang and Mo2016). (2) The Late Cretaceous Guyong granite belt in the middle Tengchong Block. These granites are mainly S-type and A-type granites with ages between 76 and 65 Ma, and they have negative zircon εHf(t) isotopic values (Xie et al. Reference Xie, Zhu, Dong, Zhao, Wang and Mo2016) and variable whole-rock εNd(t) isotopic values between −12 and 0.5 (Jiang et al. Reference Jiang, Gong, Zhang and Ma2012; Xu et al. Reference Xu, Yang, Lan, Luo, Huang, Shi and Xie2012; Ma et al. Reference Ma, Deng, Wang, Wang, Zhang and Li2013; Deng et al. Reference Deng, Wang, Li, Li and Wang2014). (3) The Palaeogene Binglangjiang granite belt in the western Tengchong Block. These granites have ages ranging from 66 to 49 Ma, and they are mainly I-type granites with zircon εHf(t) isotopic values between −4 and +6 and S-type granites with zircon εHf(t) isotopic values between −12 and −2 (Xu et al. Reference Xu, Yang, Lan, Luo, Huang, Shi and Xie2012; Deng et al. Reference Deng, Wang, Li, Li and Wang2014; Qi et al. Reference Qi, Zhu, Grimmer and Hu2015; Xie et al. Reference Xie, Zhu, Dong, Zhao, Wang and Mo2016; Sun et al. Reference Sun, Dong, Zhao, Wang and Liu2017). Minor Cambrian–Ordovician granites are located on either side of the Gaoligongshan zone (Cao et al. Reference Cao, Zou, Zhang, Zhang, Zheng, Zhang, Tang and Pei2016) and the Triassic – Early Jurassic granites occur between Tengchong and Lianghe County (Zhu et al. Reference Zhu, Lai, Qin, Zhao and Santosh2018c) with ages of 520–440 Ma and 250–190 Ma, respectively (Cao et al. Reference Cao, Zhang, Pei, Zhang, Tang, Lin and Cai2017b). In addition, there are also minor basaltic and basaltic–andesitic volcanic rocks in the Tengchong Block (Yang et al. Reference Yang, Ye, Ye, Wang, Luzhou, Na and Chang2017) with ages of 5.5–4.0 Ma, 3.9–0.9 Ma and 0.8–0.01 Ma (Zhao et al. Reference Zhao, Lai, Qin and Zhu2016a).
The metamorphic basement of the Tengchong Block is composed of the Palaeo-Neoproterozoic Gaoligongshan Group, which is mainly distributed in the eastern and western parts of the Tengchong Block (Yang et al. Reference Yang, Ye, Ye, Wang, Luzhou, Na and Chang2017). The lower segment of the Gaoligongshan Group experienced widespread migmatization and is mainly composed of gneiss, granulite and amphibole. In contrast, the upper segment of the Gaoligongshan Group is mainly composed of schists and granulites in the absence of amphibolites, with weak migmatization compared with the lower segment (Yunnan BGMR, 1990). The zircon U–Pb ages of the paragneiss and orthogneiss from this group are 1053–635 and 490–470 Ma, respectively (Song et al. Reference Song, Niu, Wei, Ji and Su2010). The basement is covered by an upper Palaeozoic – Mesozoic formation, and lower Palaeozoic sediments are absent in the Tengchong Block (Zhao et al. Reference Zhao, Lai, Qin and Zhu2016b). The sediments in the Tengchong Block are composed of carbonate and clastic rocks from the upper Palaeozoic to lower Mesozoic. The Carboniferous, Permian and Triassic formations are composed of marbles, dolomites, limestones, quartz sandstone and siltstones. The Devonian strata are dominated by siltstones, marbles and limestones. Jurassic and Cretaceous sediments are absent (Cao et al. Reference Cao, Zhang, Pei, Zhang, Tang, Lin and Cai2017b).
2.b. Deposit geology
As the largest tin deposit in the Tengchong–Lianghe tin belt, the Xiaolonghe tin deposit hosts more than 65,000 tonnes of tin (Liu et al. Reference Liu, Qin and Fan2005) and is composed of four orebodies: the Xiaolonghe, Wandanshan, Dasongpo and Huangjiashan. Abundant granitoids exist in the Xiaolonghe tin ore district, including the Guyong coarse- to medium-grained porphyritic monzogranite (GPM), the Xiaolonghe medium-grained syenogranite (XMS) and the Xiaolonghe fine-grained syenogranite (XFS), which are intrusions in the Menghong Formation (Fig. 1c). Following regional metamorphism and hydrothermal alteration, the Menghong Formation within the ore field is mainly composed of hornfels with minor marbles and quartzite (Cao et al. Reference Cao, Zou, Zhang, Zhang, Zheng, Zhang, Tang and Pei2016).
The orebodies from the Xiaolonghe tin deposit mainly occur at the top and sides of the XMS and XFS and at the inner contact zone between the syenogranite (XMS and XFS) and the Menghong Formation (Fig. 1c; Cao et al. Reference Cao, Zou, Zhang, Zhang, Zheng, Zhang, Tang and Pei2016; Chen et al. Reference Chen, Zhao, Zhu, Wang and Cui2018). These orebodies are nearly N–S distributed, which is controlled by the N–S-trending faults. Greisen vein-type mineralization mainly occurs in the deposit, which is mainly manifested as greisen veins with abundant quartz, muscovite and cassiterite, and as topaz and fluorite within the XMS and XFS (Chen et al. Reference Chen, Hu, Bi, Li, Lan, Zhao and Zhu2014, Reference Chen, Zhao, Zhu, Wang and Cui2018; Cao et al. Reference Cao, Zou, Zhang, Zhang, Zheng, Zhang, Tang and Pei2016). The hydrothermal alteration of the Xiaolonghe tin deposit is diverse and mainly includes greisenization, pyritization, silicification and carbonatization, and tin mineralization is mainly associated with greisenization (Cao et al. Reference Cao, Zou, Zhang, Zhang, Zheng, Zhang, Tang and Pei2016; Chen et al. Reference Chen, Zhao, Zhu, Wang and Cui2018; Cui et al. Reference Cui, Wang, Deng, Wu and Shu2019).
2.c. Petrography and sampling
The Guyong granite belt in the central Tengchong Block is a long and narrow wedge-shaped region that is relatively wide in the north and narrow in the south. Our study area is located in the relatively wide northern part of the Guyong granite belt (Fig. 1b). The GPM, XMS and XFS compose the major intrusions in this area (Fig. 1c). Both the Guyong intrusion (GPM) and the Xiaolonghe intrusions (XMS and XFS) show direct contact with the Menghong Formation (Chen, H. J. et al. Reference Chen, Zhang, Cao, Wang, Nie, Zhang and Tang2015) (Fig. 1c). The GPM in the study area is the dominant rock type, which occupies 70 % of the batholith and shows a gradational contact with the XMS (Fig. 1b). The XFS is distributed in limited areas and surrounded by the XMS, and the contact between them is gradational (Fig. 1c). In this study, we collected five representative GPM samples, six XMS samples and two XFS samples. The locations of these samples are shown in Figure 1b. The GPM samples were collected across the central part of the Guyong pluton, two of which were near the western border, and the other three were near the eastern border of the Guyong pluton. One XMS sample was collected in the Dasongpo area, and the rest of the XMS samples were collected in the Xiaolonghe area. All of the XFS samples were collected in the Xiaolonghe area (Fig. 1c). Most of those samples are fresh and some are slightly altered.
The GPM is porphyritic with 15–25 vol. % phenocrysts. The phenocrysts are dominated by white plagioclase and stumpy K-feldspar (mainly orthoclase with minor perthite and microcline) with sizes up to 4.5 × 4.5 cm (Fig. 2a, b). The matrix is composed of plagioclase (∼30 vol. %), K-feldspar (∼35 vol. %), quartz (∼25 vol. %), biotite (∼8 vol. %) and minor amphiboles (Fig. 3a, b, c). The accessory minerals are represented by zircon, titanite, needle-like apatite, monazite, allanite and magnetite. Titanite exhibits typical rhombic and tabular shapes (Fig. 3b). A poikilitic texture is common in monzogranite, with biotite, titanite and quartz occurring within K-feldspar (Fig. 3d). The monzogranite exhibits slight sericitization, chloritization, argillic alteration and muscovitization, showing replacement of biotite by chlorite or muscovite and plagioclase by sericite or kaolinite.
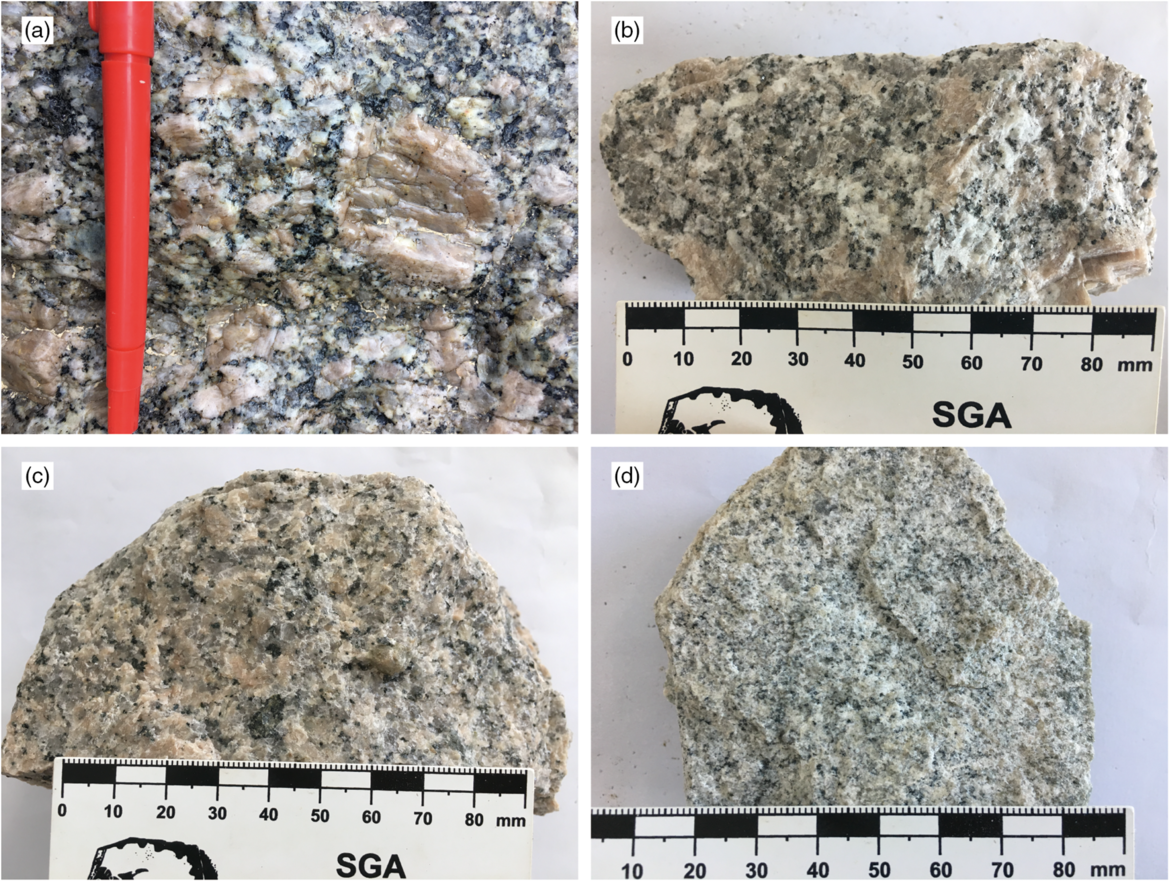
Fig. 2. Field photographs and hand specimens of the Guyong and Xiaolonghe plutons showing mineral composition and texture of (a, b) the Guyong porphyritic biotite monzogranite; (c) the Xiaolonghe medium-grained biotite syenogranite; and (d) the Xiaolonghe fine-grained syenogranite.

Fig. 3. Photomicrographs under cross-polarized light showing mineral composition and texture of (a) the Guyong porphyritic biotite monzogranite showing porphyritic texture with K-feldspar phenocryst; (b) the rhombic titanite and (c) amphibole in the monzogranite; (d) monzogranite showing poikilitic texture with biotite, titanite and quartz incorporated by K-feldspar; (e) the Xiaolonghe medium-grained syenogranite; (f) muscovite formed by the alteration of biotite and sericite from the alteration of plagioclase in the medium-grained syenogranite; (g) the Xiaolonghe fine-grained syenogranite; (h) biotite partly replaced by muscovite in the fine-grained syenogranite. Abbreviations: Qz – quartz; Pl – plagioclase; Kf – K-feldspar; Bt – biotite; Ttn – titanite; Amp – amphibole; Ser – sericite; Ms – muscovite; Mi – microcline.
The XMS is composed of K-feldspar (mainly orthoclase and microcline; ∼35 vol. %), plagioclase (∼30 vol. %), quartz (∼30 vol. %) and biotite (∼3 vol. %) (Figs 2c, 3e). The accessory minerals are zircon, needle-like apatite, monazite, allanite and magnetite, the contents of which are markedly less than those of the GPM. Biotite is partly replaced by muscovite, sericite and chlorite, whereas plagioclase is altered to kaolinite and sericite (Fig. 3f). Compared to the XMS, the XFS shows similar mineral assemblages with more quartz content (∼35 vol. %) and less biotite content (Figs 2d, 3g). Some biotite was altered to muscovite, and the plagioclase core was replaced by muscovite (Fig. 3h).
3. Analytical methods
Preparation of sample powders for whole-rock major and trace elemental analysis, as well as for whole-rock Sr–Nd isotopic analysis, were performed at the XinHang Surveying and Mapping Institute of Hebei Province in China. Prior to crushing, fresh samples of the rocks were chipped and cleaned in 5 % HCl solution. These were then ultrasonically washed in Milli-Q water and dried in a clean environment. The dried rocks were crushed into powder (200 mesh) using an agate mill. Zircon grains for U–Pb and Hf–O isotopic studies were also separated at this Institute by conventional heavy liquid and magnetic techniques. After extraction, the samples were carefully selected after being examined under a binocular microscope. Approximately 150 representative zircon grains for each sample were mounted in epoxy resin discs that were polished and cleaned. All zircon grains were photographed under transmitted and reflected light, and then images were obtained by the cathodoluminescence (CL) technique using a JEOL JXA-8900RL microprobe before the U–Pb isotope analyses. The photomicrographs and CL images of the zircons were taken to locate the analytical spots.
3.a. Whole-rock major and trace elements
The analyses of the major elemental compositions were carried out at the State Key Laboratory of Geological Processes and Mineral Resources, China University of Geosciences, Beijing. After adding 1:5 lithium metaborate, the samples were melted at 1000 °C in a muffle furnace for 15 minutes and subsequently cooled. They were extracted with 3 % HNO3 and diluted 2000 times to a constant volume. The major oxides were analysed by X-ray fluorescence (XRF) spectrometry (SHIMADZU XRF-1800), and the analytical precision and accuracy were better than 5 %. The analytical procedures followed those described by Cao et al. (Reference Cao, Pei, Zhang, Zhang, Tang, Lin and Zheng2017a). Trace elements were analysed using an Agilent 7700e ICP-MS at the Wuhan Sample Solution Analytical Technology Co., Ltd. The analytical procedures are the same as those described by Liu et al. (Reference Liu, Jiang, Zhang, Xia, Algeo and Li2018). An international standard was also analysed for every ten samples, and one blank sample was analysed for all samples.
3.b. Zircon U–Pb ages and Hf–O isotope analysis
Zircon U–Pb geochronology was performed using laser ablation inductively coupled plasma mass spectrometry (LA-ICP-MS) at the State Key Laboratory of Geological Processes and Mineral Resources, China University of Geosciences, Beijing. The analytical methods are the same as those described by Cao et al. (Reference Cao, Zhang, Pei, Zhang, Tang, Lin and Cai2017b). The beam diameter was 35 μm with a laser frequency of 8 Hz. Zircon standard 91500 was used as the external standard and run twice for every five analytical sites, while the silicate glass standard NIST610 was used to optimise the instrument. Common Pb was corrected by the method of Andersen (Reference Andersen2002). The off-line raw data selection, integration of background and analyte signals, and time-drift correction and quantitative calibration were conducted using ICP-MS DataCal (Liu et al. Reference Liu, Gao, Hu, Gao, Zong and Wang2010). Age calculations and Concordia plots were carried out using Isoplot (version 4.0) (Ludwig, Reference Ludwig2003).
In situ zircon Lu–Hf isotopic analyses were conducted using a Neptune multi-collector ICP-MS equipped with a 193 nm laser at the Tianjin Institute of Geology and Mineral Resources. During Hf isotopic analyses, a laser frequency of 10 Hz was used, and the spot size was 50 μm. Every eight-sample analysis was followed by two analyses of zircon standard GJ-1. Raw count rates for 172Yb, 173Yb, 175Lu, 176(Hf + Yb + Lu), 177Hf, 178Hf, 179Hf, 180Hf and 182W were collected, and the isobaric interference corrections for 176Lu and 176Yb on 176Hf were determined precisely. The detailed analytical technique is similar to that of Cao et al. (Reference Cao, Pei, Zhang, Zhang, Tang, Lin and Zheng2017a). The zircon standard GJ-1 yielded a 176Hf/177Hf ratio of 0.281986 ± 0.000021 (2r, N = 88) during the data acquisition, which is consistent with the recommended 176Hf/177Hf ratio of 0.282015 ± 19 (Elhlou et al. Reference Elhlou, Belousova, Griffin, Pearson and O’Reilly2006).
Zircon oxygen isotopes (18O/16O) were analysed by SHRIMP II and SHRIMP SI (Stable Isotope) ion microprobes at the Research School of Earth Sciences, The Australian National University, Canberra. The details of the analytical method are similar to those described by Fu et al. (Reference Fu, Broecker, Ireland, Holden and Kinsley2015).
3.c. Whole-rock Sr–Nd isotopes
The Sr–Nd isotopes were analysed at the State Key Laboratory of Geological Processes and Mineral Resources, School of Earth Sciences and Mineral Resources, China University of Geosciences, Beijing. The sample powder (50–100 mg) was dissolved with HF–HNO3–HCl completely. After purification by resin and HCl, the Sr and Nd samples were evaporated, and their medium was changed by using 0.1 ml HNO3. Then, they were dried again and dissolved in 3% HNO3 as preparation for isotope analysis on the MC-ICP-MS. The Sr and Nd isotopic ratios were corrected by 86Sr/88Sr = 0.1194 and 146Nd/144Nd = 0.7219, respectively. The geological standards of BCR-2 for 87Sr/86Sr and 143Nd/144Nd were 0.705029 ± 9 (2σ) and 0.512616 ± 11 (2σ) for this study, respectively. The reference standard of SRM987 for the Sr isotope shows 87Sr/86Sr = 0.710274 ± 21 (2σ, N = 61) and the Alfa Nd (an ultrapure single elemental standard solution from the Alfa Aesar A Johnson Mattey Company of the USA) for the Nd isotope shows Alfa Nd = 0.512425 ± 21.
4. Results
4.a. Zircon U–Pb ages
Three samples (16GY30, 16XLH04 and 16XLH36) from the GPM, XMS and XFS were used for LA-ICP-MS zircon U–Pb dating, and the analytical data are provided in online Supplementary Material Table S1. Most of the zircon grains are colourless and transparent. They are euhedral to subhedral and 50–300 μm in length and 1:1 to 3:1 in aspect ratio. Concordia plots (Fig. 4a, b, c) and cathodoluminescence images (Fig. 4d) of representative zircons display obvious oscillatory zoning, which is a typical feature of magmatic origins. Thus, the U–Pb age is interpreted to represent the emplacement age of those granites.

Fig. 4. (a–c) Zircon U–Pb Concordia diagrams, average ages and (d) cathodoluminescence images of representative zircon grains for the Guyong monzogranite and Xiaolonghe medium- and fine-grained syenogranite. For (d), the yellow circles represent U–Pb age spots; the dashed red circles represent Lu–Hf isotope spots; and the green circles represent O isotope spots.
Sample 16GY30 of the GPM was collected from the western part of the Guyong pluton. Twenty-three analyses yielded a weighted mean 206Pb–238U age of 75.9 ± 0.3 Ma (MSWD = 1.1) (Fig. 4a). One inherited zircon grain yielded a 206Pb–238U age of 929 Ma (Fig. 4d) and is considered to be derived from the protolith of the source materials. Sample 16XLH04 of the XMS was collected from the Xiaolonghe ore block. Twenty-four zircon grains from this rock yielded a weighted mean 206Pb–238U age of 75.6 ± 0.5 Ma (MSWD = 0.4) (Fig. 4c). Sample 16XLH36 of the XFS was collected from the Xiaolonghe ore block. Twenty-four zircon grains from this rock yielded a weighted mean 206Pb–238U age of 75.7 ± 0.3 Ma (MSWD = 0.8) (Fig. 4b). All ages above are identical within error limits.
4.b. Zircon Hf–O isotopes
In situ zircon Hf–O isotopic analysis was conducted on the same three samples (16GY30, 16XLH04 and 16XLH36) from which zircon U–Pb ages were gathered, and on the same spots on the grains (online Supplementary Material Table S2). The zircon εHf(t) isotopic values and two-stage model ages were calculated based on the emplacement age. Most of the 176Lu/177Hf ratios of the zircons are less than 0.003, which excludes any major radioactive Hf accumulation. The zircon εHf(t) values of samples 16GY30, 16XLH04 and 16XLH36 are −10.04 to −5.22, −10.63 to −3.48, and −9.53 to −3.04, with average values of −7.26, −6.39 and −6.86, and correspond to two-stage model ages of 1478 to 1784 Ma, 1364 to 1820 Ma, and 1339 to 1750 Ma, respectively. The zircon δ18O values for the three samples 16GY30, 16XLH04 and 16XLH36 are 6.69 to 8.58 ‰, 6.54 to 8.69 ‰, and 6.60 to 8.02 ‰, with average values of 7.46, 7.65 and 7.24, respectively.
4.c. Major and trace elemental compositions
All GPM, XMS and XFS samples plot in the field of subalkalic rocks in the TAS diagram (Fig. 5a). The GPM has a limited SiO2 content ranging from 71.34 to 73.29 wt %, and the XMS has a narrow but higher SiO2 content ranging from 73.65 to 76.40 wt % (online Supplementary Material Table S3). The XFS has the highest SiO2 content ranging from 76.35 to 77.33 wt %, which all belong to the field of granite (Fig. 5a). In the Q′-ANOR classification diagram, the GPM is classified as monzogranite, and the XMS and XFS belong to the field of syenogranite (Fig. 5b). The GPM is calc-alkaline and metaluminous (A/CNK = 0.95–0.98, 0.97 on average), the XMS is calc-alkaline to alkali-calcic and ranges from metaluminous to weakly peraluminous (A/CNK = 0.97–1.02, 0.99 on average), and the XFS shows a calc-alkaline affinity with weakly peraluminous features (A/CNK = 1.03–1.07, 1.05 on average) (Fig. 5c, d). The K2O/Na2O ratios of the GPM, XMS and XFS are 1.28–1.69, 1.54–1.75 and 1.12–1.16, and the total alkali contents (ALK = K2O + Na2O) are 7.87–8.48, 7.86–9.04 and 7.72–8.23 wt %, respectively. The XFS has a lower K2O content (4.14–4.34 wt %) compared to the GPM (4.41–5.33 wt %) and the XMS (4.91–5.66 wt %), largely because of alteration. The differentiation index values for the GPM, XMS and XFS are 86.05–87.75 (86.85 on average), 91.06–94.35 (92.92 on average) and 93.58–95.04 (94.31 on average), respectively. The major contents (MgO, Fe2O3T, P2O5 and TiO2) clearly decrease with the increase in SiO2 (Fig. 6) from the GPM to the XMS and XFS.

Fig. 5. (a) Total alkalis (K2O + Na2O) versus SiO2 (TAS) diagram (Middlemost, Reference Middlemost1994). (b) Q′–ANOR classification diagram (Streckeisen & Le Maitre, Reference Streckeisen and Le Maitre1979); ANOR = An/(Or + An) × 100; Q′ = Q/(Q + Or + Ab + An) × 100. (c) (Na2O + K2O − CaO) versus SiO2 diagram (Frost et al. Reference Frost, Barnes, Collins, Arculus, Ellis and Frost2001). (d) A/NK versus A/CNK diagram (Maniar & Piccoli, Reference Maniar and Piccoli1989). For (a): 1 – peridotgabbro; 2 – gabbro; 3 – gabbroic diorite; 4 – diorite; 5 – granodiorite; 6 – granite; 7 – quartzolite; 8 – monzogabbro; 9 – monzodiorite; 10 – monzonite; 11 – quartz monzonite; 12 – syenite; 13 – foid gabbro; 14 – foid monzodiorite; 15 – foid monzosyenite; 16 – foid syenite; 17 – foidolite; 18 – tawite/urtite/italite. For (b): 2 – alkalic feldspar granite; 3a – syenogranite/granite; 3b – monzogranite; 4 – granodiorite; 5 – tonalite; 6* – quartz alkali feldspar syenite; 7* – quartz monzonite; 8* – quartz monzodiorite; 9* – quartz monzodiorite/quartz monzogabbro; 10* – quartz diorite/quartz gabbro/quartz anorthosite; 6 – alkali feldspar syenite; 7 – syenite; 8 – monzonite; 9 – monzodiorite/monzogabbro; 10 – diorite/gabbro/anorthosite. Data sources: Jiang et al. (Reference Jiang, Gong, Zhang and Ma2012); Zhang et al. (Reference Zhang, Jin, Fan, Zhang, Shen, Gao and Cheng2013); Cao (Reference Cao2015); Chen, X. C. et al. (Reference Chen, Hu, Bi, Zhong, Lan, Zhao and Zhu2015); Qi et al. (Reference Qi, Zhu, Grimmer and Hu2015); Cao et al. (Reference Cao, Zou, Zhang, Zhang, Zheng, Zhang, Tang and Pei2016); Dong (Reference Dong2016); Yu (Reference Yu2016); Zhao et al. (Reference Zhao, Lai, Qin, Zhu and Wang2017b).

Fig. 6. Variation diagrams of major elements versus SiO2. (a) MgO; (b) Fe2O3T; (c) P2O5; (d) TiO2.
The GPM, XMS and XFS have total rare earth element (ΣREE) contents of 268.74–309.00 ppm (average 296.23 ppm), 286.54–396.82 ppm (average 341.52 ppm) and 243.93–252.78 ppm (average 248.35 ppm), respectively. The light REE/heavy REE (LREE/HREE) ratios for the GPM, XMS and XFS are in the range of 8.74–10.47 (average 9.49), 4.96–6.58 (average 5.65) and 1.50–1.95 (average 1.73), respectively; the (La/Yb) N ratios are 7.84–10.77 (average 9.45), 4.54–6.32 (average 5.25) and 0.70–0.92 (average 0.81), respectively; and the δEu values are in the range of 0.28–0.37, 0.058–0.079 and 0.008–0.012, respectively. In the chondrite-normalized REE diagram, the GPM shows rightward slope patterns and slight negative Eu anomalies. Compared with the GPM, the XMS shows higher HREE contents and obvious negative Eu anomalies, but the LREE contents do not show much variation (Fig. 7a). The XFS shows the lowest LREE and highest HREE contents, with marked negative Eu anomalies compared with the former two granites, which exhibit a relatively flat ‘seagull’ pattern. An apparent tetrad effect is clearly observed in the M-shaped REE patterns of the XFS, with four convex curves defined by La–Ce–Pr–Nd, (Pm)–Sm–Eu–Gd, Gd–Tb–Dy–Ho and Er–Tm–Yb–Lu. The most abundant elements for each group of convex curves previously mentioned are Ce, Sm, Dy and Yb, respectively. All samples of the granitoids are depleted in Ba, Sr, P and Ti, and the degree of depletion increases from the GPM to the XMS and XFS, as seen in the primitive mantle-normalized trace-element spider diagram. The LILEs (e.g. Rb and U) and Ta show enrichment, whereas Zr shows a decrease from the GPM to the XMS and XFS (Fig. 7b).

Fig. 7. (a) Chondrite-normalized rare earth element (REE) patterns and (b) primitive mantle-normalized multi-element patterns for the Guyong monzogranite and Xiaolonghe medium- and fine-grained syenogranite. Symbols are the same as Figure 5. Chondrite-normalized and primitive mantle-normalized data are from Sun & McDonough (Reference Sun, McDonough, Saunders and Norry1989). The data for the crust are from Rudnick & Gao (Reference Rudnick, Gao, Holland and Turekian2003); the data for the SMG are from Jiang et al. (Reference Jiang, Li, Jiang, Wang and Wei2017) and Li et al. (Reference Li, Myint, Yonezu, Watanabe, Algeo and Wu2018); the other data sources are the same as those in Figure 5.
4.d. Sr–Nd isotopes
The whole-rock Sr–Nd isotopic compositions of three samples for the GPM are listed in Table 1. The initial 87Sr/86Sr ratios (I Sr) and whole-rock εNd(t) isotopic values were calculated based on the emplacement age of 76 Ma. The GPM has relatively higher I Sr ratios (0.7098–0.7099), with whole-rock εNd(t) isotopic values in the range of −9.7 to −10.1, and single-stage Nd model ages of 1.56–1.63 Ga.
Table 1. Whole-rock Sr–Nd isotopic data for the Guyong monzogranite.

5. Discussion
5.a. Petrogenesis of monzogranite
The compositional variations in granitoids are mainly controlled by partial melting and fractional crystallization (Gao et al. Reference Gao, Zheng and Zhao2016; Zhang et al. Reference Zhang, Wang, Zhu, Li, Zhao, Zhang, Chen, Liu, Zheng, Wang and Liao2019). All samples from the GPM, XMS and XFS have high Rb/Sr ratios and low Sr contents, which cannot be generated by equilibrium partial melting, and they require fractional crystallization (Halliday et al. Reference Halliday, Davidson, Hildreth and Holden1991). Moreover, the high differentiation index values of the XMS (91.06–94.35) and XFS (93.58–95.04) also indicate that they have experienced fractional crystallization. The U–Pb ages of the GPM, XMS and XFS are consistent (75.9 Ma, 75.6 Ma and 75.7 Ma, respectively), which suggests that they were derived from the same batch of magma (Zhang, Q. W. et al. Reference Zhang, Wang, Li and Cui2018). Therefore, we consider these intrusions to be cogenetic, and the XMS and XFS represent the fractionated products of the GPM.
Granites cannot be generated by direct melting of the mantle, and the major mechanism that produces granitic magmas is partial melting of crustal rocks (Brown, Reference Brown2013; Gao et al. Reference Gao, Zheng and Zhao2016; Scaillet et al. Reference Scaillet, Holtz and Pichavant2016). The GPM is most close to the initial melt composition compared to the XMS and XFS because of crystal fractionation (Sawyer et al. Reference Sawyer, Cesare and Brown2011). From Figure 7a, it is seen that the GPM is more enriched than the upper crust, and its enrichment in LREEs and depletion in Eu possibly results from anatexis of the lower crust (Sawyer et al. Reference Sawyer, Cesare and Brown2011; Brown, Reference Brown2013; Wu et al. Reference Wu, Liu, Ji, Wang and Yang2017). To further constrain the source region of the GPM, we evaluated the zircon Hf–O for all of the samples and the whole-rock Sr–Nd isotopes for the GPM due to the XMS. It was found that the XFS has high Rb/Sr ratios and shows unreasonably high initial 87Sr/86Sr ratios with a wide range (Chen, X. C. et al. Reference Chen, Hu, Bi, Zhong, Lan, Zhao and Zhu2015). The GPM has similar Sr–Nd isotope characteristics as the Early Cretaceous granites (Fig. 8) derived from ancient lower crust in the east Tengchong Block and Gaoligong belt (Yang et al. Reference Yang, Xu, Huang and Luo2006; Zhu et al. Reference Zhu, Lai, Qin and Zhao2015, Reference Zhu, Lai, Qin, Zhao and Wang2017a, Reference Zhu, Lai, Qin and Zhao2018a,b; Zhao et al. Reference Zhao, Lai, Qin and Zhu2016b, Reference Zhao, Lai, Qin, Zhu and Gan2017a; Zhu, Reference Zhu2017; Wan, Reference Wan2018; Wan et al. Reference Wan, Yang, Li and Chen2018; Zhang, J. Y. et al. Reference Zhang, Peng, Fan, Zhao, Dong, Gao, Peng, Wei, Xia, Chen and Liang2018). The GPM is different from the Eocene granitic rocks in the west Tengchong Block with diverse Sr–Nd isotope values (Fig. 8), which were derived from ancient crust (Wu, Reference Wu2014; Zhao et al. Reference Zhao, Lai, Qin and Zhu2016a, Reference Zhao, Lai, Pei, Qin, Zhu, Tao and Gao2019) or mixed source regions composed of both ancient and juvenile crustal rocks (Ma et al. Reference Ma, Wang, Fan, Geng, Cai, Zhong, Liu and Xing2014). Thus, the high initial 87Sr/86Sr ratios (from 0.7098 to 0.7099) of the GPM (Fig. 8) together with the negative whole-rock εNd(t) isotopic values (from −9.7 to −10.1) indicate that they were generated by partial melting of ancient lower crust.

Fig. 8. Whole-rock εNd(t) values versus initial 87Sr/86Sr values for the Guyong monzogranite from this study (GPM; circles with black border) and intrusive rocks varying from mafic to felsic in the Tengchong Block from the literature (Ji et al. Reference Ji, Zhong and Chen2000; Yang et al. Reference Yang, Xu, Huang and Luo2006, Reference Yang, Xu, Huang, Luo and Shi2009; Xu et al. Reference Xu, Lan, Yang, Huang and Qiu2008; Ma et al. Reference Ma, Deng, Wang, Wang, Zhang and Li2013, Reference Ma, Wang, Fan, Geng, Cai, Zhong, Liu and Xing2014; Wang et al. Reference Wang, Zhang, Cawood, Ma, Fan, Zhang, Zhang and Bi2014, Reference Wang, Li, Ma, Fan, Cai, Zhang and Zhang2015; Wu, Reference Wu2014; Zhu et al. Reference Zhu, Lai, Qin and Zhao2015, Reference Zhu, Lai, Qin, Zhao and Wang2017a; Reference Zhu, Lai, Qin and Zhao2018a,b; Zhao et al. Reference Zhao, Lai, Qin and Zhu2016a,b, Reference Zhu, Lai, Qin, Zhao and Wang2017a,b, Reference Zhao, Lai, Pei, Qin, Zhu, Tao and Gao2019; Zhu, Reference Zhu2017; Wan, Reference Wan2018; Wan et al. Reference Wan, Yang, Li and Chen2018; Zhang, J. Y. et al. Reference Zhang, Peng, Fan, Zhao, Dong, Gao, Peng, Wei, Xia, Chen and Liang2018) (shapes with red border, all the data are recalculated for the age of 76 Ma). Abbreviations: WT – West Tengchong; CT – Central Tengchong; ET – East Tengchong; GLG – Gaoligong.
All zircon εHf(t) isotopic values of the GPM, XMS and XFS are negative and below the value of CHUR (chondritic uniform reservoir) (Fig. 9a), and they show similarity with the Southern Myanmar Sn-bearing granites (SMG) that were derived from partial melting of the Palaeoproterozoic continental crust (Jiang et al. Reference Jiang, Li, Jiang, Wang and Wei2017). This similarity suggests that the magma was derived from an ancient crustal source. As all the 147Sm/144Nd ratios are between 0.13 and 0.10, the single-stage Nd model ages (∼1.6 Ga) that are consistent with the two-stage Hf model ages (from 1.5 to 1.8 Ga for GPM, 1.4 to 1.8 Ga for XMS, and 1.3 to 1.8 Ga for XFS) can represent the age of the source region (Li, Reference Li1996). Therefore, the parental magma of the GPM was generated by partial melting of ancient crust separated from depleted mantle during Mesoproterozoic times (Yang et al. Reference Yang, Wu, Wilde, Xie, Yang and Liu2007; Qi et al. Reference Qi, Zhu, Grimmer and Hu2015). According to Li et al. (Reference Li, Chen, Hou and Luo2016), the Gaoligong metamorphic complex shows four age peaks in the Neoarchaean (∼2.5 Ga), Mesoproterozoic (∼1.6 Ga), Grenvillian (∼1.17 Ga and 0.95 Ga) and Late Neoproterozoic – Cambrian (0.65–0.5 Ga). In summary, together with the presence of an inherited zircon of 929 Ma from the GPM, we consider that the magma that formed the GPM was generated by partial melting of the Proterozoic Gaoligong metamorphic complex.

Fig. 9. (a) Zircon εHf(t) values versus U–Pb ages (Ma); (b) zircon δ18O values versus εHf(t) values for the Guyong monzogranite and Xiaolonghe medium- and fine-grained syenogranite. Fields in (b) are from Kemp et al. (Reference Kemp, Hawkesworth, Paterson and Kinny2006) and Kemp et al. (Reference Kemp, Hawkesworth, Foster, Paterson, Woodhead, Hergt, Gray and Whitehouse2007). The data for the SMG are from Jiang et al. (Reference Jiang, Li, Jiang, Wang and Wei2017).
The GPM has brown biotite, rare amphibole and inherited zircon with accessory minerals of titanite, which show the characteristics of I-type granites (Brown, Reference Brown2013; Gao et al. Reference Gao, Zheng and Zhao2016; Jagoutz & Klein, Reference Jagoutz and Klein2018; Jeon & Williams, Reference Jeon and Williams2018). The similar δ18O values of the GPM, XMS and XFS (between 6.5 ‰ and 9 ‰; Fig. 9b) and the wide range in zircon εHf(t) isotopic values (between −10.04 and −3.04) suggest that they are connected and their source region incorporates metasedimentary and meta-igneous rocks (Kemp et al. Reference Kemp, Hawkesworth, Paterson and Kinny2006; Gardiner et al. Reference Gardiner, Hawkesworth, Robb, Whitehouse, Roberts, Kirkland and Evans2017; Yang et al. Reference Yang, Ye, Ye, Wang, Luzhou, Na and Chang2017). Therefore, the GPM was generated by the partial melting of the metasedimentary and meta-igneous rocks from the Gaoligong crystalline basement.
5.b. Fractional crystallization
From analysis of the change in the geochemical compositions, we can infer the detailed processes of fractional crystallization. Although the contents of Rb, U and Ta increase and those of Ba, Sr, P, Zr, Ti and Eu decrease from the GPM to the XMS and XFS (Fig. 7b), the trace-element ratios of Rb/Sr, Rb/Ba, Zr/Hf and Nb/Ta are more appropriate to evaluate the degree of fractional crystallization (Halliday et al. Reference Halliday, Davidson, Hildreth and Holden1991; Deering & Bachmann, Reference Deering and Bachmann2010; Stepanov et al. Reference Stepanov, Mavrogenes, Meffre and Davidson2014; Ballouard et al. Reference Ballouard, Poujol, Boulvais, Branquet, Tartese and Vigneresse2016; Wu et al. Reference Wu, Liu, Ji, Wang and Yang2017). It is clear from Figure 10 that there is an increase in Rb/Ba ratio from the monzogranite to the syenogranite, indicating fractionation of biotite and K-feldspar. The marked negative Eu anomalies and the decrease in Sr content suggest the control of plagioclase and K-feldspar. The increase in Rb/Ba ratio can result from the fractionation of either biotite and K-feldspar with a 2:1 ratio (Fig. 10a) or by K-feldspar alone (Fig. 10b). Based on our petrographic observation, the increase in Rb/Ba ratio is correlated to biotite and K-feldspar rather than to K-feldspar only. Since it is difficult to generate prominent negative Eu anomalies from the fractionation of plagioclase alone (Figs 7a, 10b), we consider that the decrease in Sr content (Fig. 10a) and δEu are induced by the fractionation of both plagioclase and K-feldspar. The decrease in Zr/Hf ratios is correlated with the fractional crystallization of zircon (Deering & Bachmann, Reference Deering and Bachmann2010; Dostal et al. Reference Dostal, Kontak, Gerel, Shellnutt and Fayek2015; Zhang et al. Reference Zhang, Wang, Zhu, Li, Zhao, Zhang, Chen, Liu, Zheng, Wang and Liao2019), which is also consistent with the decrease in zircon content from the GPM to the XMS and XFS. As shown in Figure 7a, the GPM and XMS have similar LREE contents, but the XFS has relatively lower LREE contents, and the HREE contents increase gradually, resulting in the change in slopes of the REE patterns from steeply negative to nearly flat. In most granitoids, the majority of the REEs are carried by accessory minerals, and monazite and allanite are extremely rich in LREEs. The decrease in LREE content in the XFS may be related to the fractional crystallization of these two minerals (Miller & Mittlefehldt, Reference Miller and Mittlefehldt1982, Reference Miller and Mittlefehldt1984; Fisher et al. Reference Fisher, Hanchar, Miller, Phillips, Vervoort and Whitehouse2017; Zhang et al. Reference Zhang, Wang, Zhu, Li, Zhao, Zhang, Chen, Liu, Zheng, Wang and Liao2019). Because HREEs are not strongly concentrated in these minerals, which cause the depletion of LREEs, they behave as incompatible elements, and their concentrations gradually increase from the GPM to the XMS and XFS (Miller & Mittlefehldt, Reference Miller and Mittlefehldt1984).

Fig. 10. (a) Sr contents (ppm) versus Rb/Ba ratios; (b) Eu anomalies (δEu) versus Rb/Ba ratios for the Guyong monzogranite and Xiaolonghe medium- and fine-grained syenogranite. The partition coefficients for biotite, K-feldspar and plagioclase are based on Bachmann et al. (Reference Bachmann, Dungan and Bussy2005). White circles and blue lines on vectors show 10 % fractionation increments. Abbreviations: Bt – biotite; Kf – K-feldspar; Pl – plagioclase. The data sources are the same as those in Figure 5.
The contents of MgO and Fe2O3T clearly decrease with the increase in SiO2 (Fig. 6a, b) from the GPM to the XMS and XFS, which indicates the fractional crystallization of ferromagnesian minerals (mostly biotites) (Miller & Mittlefehldt, Reference Miller and Mittlefehldt1984). The saturation of apatite in the GPM caused a rapid decrease in P content from the GPM to the XMS and XFS with further fractional crystallization (Fig. 6c; Lee & Bachmann, Reference Lee and Bachmann2014). The TiO2 clearly decreases with the increase in SiO2 (Fig. 6d) and with the depletion of Ti (Fig. 7b) from the GPM to the XMS and XFS, and this outcome indicates the fractional crystallization of Ti-bearing oxides, such as ilmenite and titanite (Liu et al. Reference Liu, Hu, Gao, Feng, Huang, Lai, Yuan, Liu, Coulson, Feng, Wang and Qi2009; Stepanov et al. Reference Stepanov, Mavrogenes, Meffre and Davidson2014). Ti-bearing oxides or silicates (micas and amphiboles) are the major hosts for Ta and Nb in granites (Stepanov et al. Reference Stepanov, Mavrogenes, Meffre and Davidson2014; Tang et al. Reference Tang, Lee, Chen, Erdman, Costin and Jiang2019). According to Stepanov et al. (Reference Stepanov, Mavrogenes, Meffre and Davidson2014), fractionation of Ti-bearing oxides decreases the Ta/Nb ratios and Ta content (ilmenite and titanite), whereas biotite increases the Ta/Nb ratios and Ta content. The slight increase in Ta content and Ta/Nb ratios with decreasing Ti from the GPM to the XMS is significantly different from the trends displayed by fractionation of ilmenite and titanite (Fig. 11). Thus, the Ta–Nb distinction between the GPM and XMS is accounted for by the fractionation of biotite (Stepanov et al. Reference Stepanov, Mavrogenes, Meffre and Davidson2014).

Fig. 11. (a) Ta/Nb ratios versus TiO2 contents (wt %); (b) Ta/Nb ratios versus Ta contents (ppm) for the Guyong monzogranite and Xiaolonghe medium- and fine-grained syenogranite (Stepanov et al. Reference Stepanov, Mavrogenes, Meffre and Davidson2014). Abbreviations: Bt – biotite; Ms – muscovite; Mag – magnetite; Ilm – ilmenite; Rt – rutile; Ttn – titanite; Amph – amphibole. The data sources are the same as those in Figure 5.
In summary, the GPM, XMS and XFS were probably derived from the same batch of magma, and the difference in composition is attributed to the fractional crystallization of biotite, plagioclase, K-feldspar, apatite, ilmenite and titanite. The MUSH model can be invoked in this case, where crystal mush (crystals + melt) crystallized to form a granite rich in K-feldspar phenocrysts (GPM), whereas the residual melt extracted from the mush zone generated the XMS and XFS (Lee & Bachmann, Reference Lee and Bachmann2014; Lee & Morton, Reference Lee and Morton2015; Wu et al. Reference Wu, Liu, Ji, Wang and Yang2017).
5.c. Metallogenic implications
The U–Pb ages of cassiterite (72–74 Ma) and 40Ar–39Ar ages of muscovite (72–73 Ma) from the Xiaolonghe tin deposit (Chen et al. Reference Chen, Hu, Bi, Li, Lan, Zhao and Zhu2014; Cao et al. Reference Cao, Zou, Zhang, Zhang, Zheng, Zhang, Tang and Pei2016) indicate that the deposit formed simultaneously with the Guyong and Xiaolonghe plutons (Xu et al. Reference Xu, Yang, Lan, Luo, Huang, Shi and Xie2012; Chen, X. C. et al. Reference Chen, Hu, Bi, Zhong, Lan, Zhao and Zhu2015; Qi et al. Reference Qi, Zhu, Grimmer and Hu2015; Xie et al. Reference Xie, Zhu, Dong, Zhao, Wang and Mo2016), and this outcome implies a genetic relationship between the tin deposit and magmatism (Cao et al. Reference Cao, Zhang, Santosh, Zhang, Tang, Pei and Yang2018). Previous studies regarded the XMS to be genetically related to the tin deposit from the perspective of formation age and stable isotopic (C–H–O–S–Pb–He–Ar) compositions (Cao et al. Reference Cao, Zou, Zhang, Zhang, Zheng, Zhang, Tang and Pei2016; Chen et al. Reference Chen, Zhao, Zhu, Wang and Cui2018); little attention was paid to the relationship between the XFS and tin mineralization. The granites from the Guyong and Xiaolonghe plutons have relatively high Sn contents (8.14–12.3 ppm for GPM, 8.72–21.1 ppm for XMS, and 11.0–20.2 ppm for XFS), which are higher than normal granites without tin mineralization (4.3 ppm; Tischendorf, Reference Tischendorf, Stemprok, Burnol and Tischendorf1977), and the Sn contents of the Xiaolonghe granites (XMS and XFS) are closer to those of Sn specialized granites (Sn ≥15; Neiva, Reference Neiva1984; Fig. 12); this outcome suggests that the Xiaolonghe granites have more Sn metallogenic potential. Ore-forming granites crystallize from silicate melt in the latest stage of crystallization differentiation, which generates volatiles through exsolution and changes the magma system from crystal–melt interaction to crystal–melt–magmatic volatile interaction (Lehmann, Reference Lehmann1990; Halter & Webster, Reference Halter and Webster2004). The Sn contents increase with the increase in SiO2 from the GPM to the XMS and XFS (Fig. 12), indicating that the fractionation of magma can enhance the concentrations of Sn in the residual melt, which is a necessary precursor for the mineralization and formation of tin deposits (Mustard et al. Reference Mustard, Ulrich, Kamenetsky and Mernagh2006; Gardiner et al. Reference Gardiner, Hawkesworth, Robb, Whitehouse, Roberts, Kirkland and Evans2017). The XFS has a similar Sn content as the XMS, indicating that the Sn concentration might reach a critical threshold for exsolution from melt to fluid (Gardiner et al. Reference Gardiner, Hawkesworth, Robb, Whitehouse, Roberts, Kirkland and Evans2017).

Fig. 12. Evolution of Sn contents (ppm) versus SiO2 contents (wt %) for the Guyong monzogranite and Xiaolonghe medium- and fine-grained syenogranite. The green hollow circles represent the data from Chen, X. C. et al. (Reference Chen, Hu, Bi, Zhong, Lan, Zhao and Zhu2015) and the red hollow circles represent our unpublished data. The Sn content of normal granites is from Tischendorf (Reference Tischendorf, Stemprok, Burnol and Tischendorf1977) and the specialized granites is from Neiva (Reference Neiva1984); the other data sources are the same as those in Figure 5.
The tetrad effect of granites is an efficacious indicator of the metallogenic potential of rare metals (Irber, Reference Irber1999; Monecke et al. Reference Monecke, Dulski and Kempe2007; Yang et al. Reference Yang, Wang, Zhang, Dan, Zhang, Qi, Xia and Zhao2018). Figure 13a shows that as the Nb/Ta ratios decrease, the degree of the tetrad effect (TE 1–3) quantified by Irber (Reference Irber1999) increases significantly from the GPM to the XMS and XFS. The XFS displays a high tetrad effect (TE 1–3 >1.1) with low Nb/Ta ratios (<∼5), which are similar to the SMG (Jiang et al. Reference Jiang, Li, Jiang, Wang and Wei2017; Li et al. Reference Li, Myint, Yonezu, Watanabe, Algeo and Wu2018), and this outcome suggests an interaction between magma and fluids (Ballouard et al. Reference Ballouard, Poujol, Boulvais, Branquet, Tartese and Vigneresse2016). The Zr/Hf ratio is also a marker of either fractional crystallization or magmatic–hydrothermal interactions (Ballouard et al. Reference Ballouard, Poujol, Boulvais, Branquet, Tartese and Vigneresse2016). In Figure 13b, the XFS shows similar characteristics to the SMG and belongs to the field of rare metal-related granites (Ta–Cs–Li–Nb–Be–Sn–W); both the GPM and XMS belong to the field of barren granites. In addition, the average zircon δEu values of the GPM and XMS are greater than 0.08 (online Supplementary Material Table S4), which are similar to barren granites (Gardiner et al. Reference Gardiner, Hawkesworth, Robb, Whitehouse, Roberts, Kirkland and Evans2017); meanwhile, those of the XFS are equal to or less than 0.08, which indicates tin-producing granites. Therefore, we conclude that the latest XFS is an ore-forming intrusion and that Sn is scavenged by the exsolved hydrothermal fluids.

Fig. 13. (a) Evolution of Nb/Ta ratios versus degree of tetrad effect (TE 1–3); (b) Nb/Ta ratios versus Zr/Hf ratios diagram to differentiate barren and ore-forming granites for the Guyong monzogranite and Xiaolonghe medium- and fine-grained syenogranite (Ballouard et al. Reference Ballouard, Poujol, Boulvais, Branquet, Tartese and Vigneresse2016). The data for the SMG are from Jiang et al. (Reference Jiang, Li, Jiang, Wang and Wei2017) and Li et al. (Reference Li, Myint, Yonezu, Watanabe, Algeo and Wu2018); the other data sources are the same as those in Figure 5.
5.d. Tectonic implications
Granites can be produced in diverse tectonic settings, such as continental collisions, continental rifts, post-continental settings and subduction zones (Jagoutz & Klein, Reference Jagoutz and Klein2018). The main arguments about the tectonic setting of the Tengchong Late Cretaceous granites in previous studies are as follows: (1) The culmination of crustal thickening, induced by the eastward subduction of the Neo-Tethys and plate convergence, and subsequent extensional collapse led to the generation of the Late Cretaceous granites in the Tengchong Block (Xu et al. Reference Xu, Yang, Lan, Luo, Huang, Shi and Xie2012; Ma et al. Reference Ma, Deng, Wang, Wang, Zhang and Li2013; Cao et al. Reference Cao, Zhang, Tang, Hollis, Zhang, Pei, Yang and Zhu2019); (2) underplating of basaltic magma generated by partial melting of the mantle during the eastward subduction of the Neo-Tethys resulted in the melting of the thickened lower crust which formed the Late Cretaceous granites in the Tengchong Block (Yang et al. Reference Yang, Xu, Huang, Luo and Shi2009); (3) the Tengchong Late Cretaceous granites are A-type granites generated during the post-orogenic extension stage after collision of the Tengchong and Baoshan blocks (Jiang et al. Reference Jiang, Gong, Zhang and Ma2012; Zhang et al. Reference Zhang, Jin, Fan, Zhang, Shen, Gao and Cheng2013); (4) the collision between the Tengchong and Burma blocks along with subduction of the Neo-Tethys caused the thickening of crust, and subsequent extensional collapse during Late Cretaceous times produced the Xiaolonghe granites (Chen, X. C. et al. Reference Chen, Hu, Bi, Zhong, Lan, Zhao and Zhu2015).
The Tengchong Block and Mogok–Mandalay–Mergui belt in the SE Asian tin belt have been structurally linked since Early Cretaceous times (Xu et al. Reference Xu, Wang, Cai, Dong, Li, Chen, Duan, Cao, Li and Burg2015). The Late Cretaceous SMG are typical Sn-bearing granites from the SE Asian tin belt and are mostly derived from the partial melting of ancient crust during the subduction of the Neo-Tethys Ocean (Jiang et al. Reference Jiang, Li, Jiang, Wang and Wei2017; Myint et al. Reference Myint, Zaw, Swe, Yonezu, Cai, Manaka, Watanabe, Barber, Zaw and Crow2017; Li et al. Reference Li, Myint, Yonezu, Watanabe, Algeo and Wu2018). The certain geochemical similarities of the Late Cretaceous granites suggest a genetic link between them (Figs 7, 9, 13). Furthermore, some of the Late Cretaceous deposits from the SE Asian tin belt and the Xiaolonghe deposit have almost the same metallogenic ages (Cao et al. Reference Cao, Zou, Zhang, Zhang, Zheng, Zhang, Tang and Pei2016; Gardiner et al. Reference Gardiner, Robb, Morley, Searle, Cawood, Whitehouse, Kirkland, Roberts and Myint2016). Therefore, the geochronological and geochemical evidence indicates that they experienced consistent tectono-magmatic histories during Late Cretaceous times (Cao et al. Reference Cao, Zou, Zhang, Zhang, Zheng, Zhang, Tang and Pei2016; Xie et al. Reference Xie, Zhu, Dong, Zhao, Wang and Mo2016).
The geothermal gradients of 20 °C km−1 cannot generate enough heat for the melting of crustal rocks (Petford et al. Reference Petford, Cruden, McCaffrey and Vigneresse2000). Therefore, an external heat source for the melting of crustal rocks is required. Although granitic rocks cannot be generated by the partial melting of mantle peridotite, asthenosphere upwelling in the orogenic belt can provide thermal input for the melting of crustal rocks. The mafic dykes in the Lianghe area generated by the partial melting of upwelling asthenosphere indicate the break-off of the Neo-Tethys slab at ∼40 Ma beneath the Tengchong Block (Xu et al. Reference Xu, Lan, Yang, Huang and Qiu2008). Wang et al. (Reference Wang, Zhang, Cawood, Ma, Fan, Zhang, Zhang and Bi2014) considered that the rollback of the Neo-Tethys slab beneath the west Tengchong Block during ∼55–50 Ma marks the transition from subduction to initial collision of India–Asia. Ma et al. (Reference Ma, Wang, Fan, Geng, Cai, Zhong, Liu and Xing2014) considered that the juvenile mantle-derived basaltic magma underplating the ancient crust beneath the west Tengchong Block during ∼60–70 Ma resulted from the subduction of the Neo-Tethys, and the initiation of the collision of India–Asia was not earlier than ∼55 Ma. The upwelling and underplating of mantle-derived magma induced by subduction of the Neo-Tethys provided the heat source for the partial melting of crustal rocks (Chen, X. C. et al. Reference Chen, Hu, Bi, Zhong, Lan, Zhao and Zhu2015; Jiang et al. Reference Jiang, Li, Jiang, Wang and Wei2017). The subduction of the Neo-Tethys beneath the Tengchong Block changed from flat to steep, and the convergence rate increased rapidly from c. 70 Ma (Cao et al. Reference Cao, Zhang, Santosh, Zhang, Tang, Pei and Yang2018). Thus, we consider that the Late Cretaceous (∼75 Ma) magma in the central Tengchong Block was produced by the change in subduction angle, which resulted in the upwelling of the asthenosphere that provided heat for the melting of crustal rocks. For the SMG, the heat is also provided by the upwelling of the asthenosphere that is induced by the rollback of the Neo-Tethyan subducting slab (Jiang et al. Reference Jiang, Li, Jiang, Wang and Wei2017; Myint et al. Reference Myint, Zaw, Swe, Yonezu, Cai, Manaka, Watanabe, Barber, Zaw and Crow2017). Correlating the ages of the Tengchong Late Cretaceous granites and the SMG (Cao et al. Reference Cao, Zou, Zhang, Zhang, Zheng, Zhang, Tang and Pei2016; Jiang et al. Reference Jiang, Li, Jiang, Wang and Wei2017), we propose that the subduction of the Neo-Tethys resulted in the upwelling of the asthenosphere and subsequent partial melting of the lower crust, resulting in the formation of the parental magma of the Tengchong Late Cretaceous granites and the SMG. Then, the process of fractional crystallization led to the enrichment of Sn and the generation of Sn-bearing granites.
6. Conclusions
The key results from this study are as follows.
(1) Compared with the GPM, the XMS and XFS have more quartz, less feldspar and biotite, and show strong depletion in Eu, Ba, Sr, P and Ti and relative enrichment of HREEs, Rb and Ta.
(2) The parental magma of the GPM was derived from the partial melting of ancient crust in the Tengchong Block. The XMS and XFS are considered products of differentiation of the GPM through the fractionation of feldspars, apatite, biotite, apatite, ilmenite and titanite.
(3) The XFS is regarded as the ore-forming granite, and Sn was scavenged by exsolved hydrothermal fluids.
(4) The Late Cretaceous magmatic activities in the Tengchong Block were related to the subduction of the Neo-Tethys, which resulted in the upwelling of the asthenosphere and subsequent partial melting of the lower crust.
Supplementary Material
To view supplementary material for this article, please visit https://doi.org/10.1017/S0016756819001493
Acknowledgements
This work was supported by The National Key Research and Development Program (Grant No. 2016YFC0600502) and National Science Foundation (9175520034) of China. The two anonymous referees and editor Kathryn Goodenough are also thanked for offering constructive comments and suggestions for improving this manuscript.