Introduction
The main mafic silicates in granitic rocks, such as amphiboles and biotite, have been investigated extensively, given that their chemistry and compositional variations help to trace evolutionary geochemical pathways in magmatic (e.g. Rasmussen and Mortensen, Reference Rasmussen and Mortensen2013; Papoutsa and Pe-piper, Reference Papoutsa and Pe-piper2014; Sarjoughian et al., Reference Sarjoughian, Kananian, Ahmadian and Murata2015; Siegel et al., Reference Siegel, Williams-Jones and van Hinsberg2017; Vasyukova and Williams–Jones, Reference Vasyukova and Williams-Jones2019) and hydrothermal (e.g. Parsapoor et al., Reference Parsapoor, Khalili, Tepley and Maghami2015; Vilalva et al., Reference Vilalva, Vlach and Simonetti2016; Jin et al., Reference Jin, Gao, Chen and Zhao2018; Xie et al., Reference Xie, Wang, Wang, Zhu, Che, Gao and Zhao2018) granitic systems. These data also allow us to estimate crystallisation conditions (e.g. Wones, Reference Wones1981; Anderson et al., Reference Anderson, Barth, Wooden, Mazdab, Putirka and Tepley2008; Zhang et al., Reference Zhang, Lentz, Thorne and Mcfarlane2016; Polzunenkov, Reference Polzunenkov2018; Huang et al., Reference Huang, Scaillet, Wang, Erdmann, Chen, Faure, Liu, Xie, Wang and Zhu2019; Pham et al., Reference Pham, Shellnutt, Yeh and Iizuka2020) and to identify magmatic lineages (e.g. Nachit et al., Reference Nachit, Razafimahefa, Stussi and Carron1985) together with their metallogenetic potentials (e.g. Baidya et al., Reference Baidya, Paul, Pal and Upadhyay2017; Huang et al., Reference Huang, Wang, Zhang, Li and Qin2018; Kaur et al., Reference Kaur, Chaudhri and Eliyas2018; Araujo et al., Reference Araujo, Martins, Pereira and Janasi2020).
There are many studies dealing with the composition and variability of the main mafic minerals occurring in A-type granites and related rocks, which substantially improved the understanding and implications for the magmatic and post-magmatic (including hydrothermal) crystallisation environments (e.g. Marks et al., Reference Marks, Vennemann, Siebel and Markl2003; Gualda and Vlach, Reference Gualda and Vlach2007a, Reference Gualda and Vlach2007b, Reference Gualda and Vlach2007c; Shellnutt and Iizuka, Reference Shellnutt and Iizuka2011; Piilonen et al., Reference Piilonen, McDonald, Poirier, Rowe and Larsen2013; Moreno et al., Reference Moreno, Molina, Montero, Abu Anbar, Scarrow, Cambeses and Bea2014; Vilalva et al., Reference Vilalva, Vlach and Simonetti2016; Papoutsa et al., Reference Papoutsa, Pe-Piper and Piper2016; Andersen et al., Reference Andersen, Elburg and Erambert2017; Bernard et al., Reference Bernard, Estrade, Salvi, Béziat and Smith2020). Textural and compositional features of these minerals in typical granite and syenite occurrences within the post-collisional A-type Graciosa Province (S-SE Brazil), have also been investigated, particularly to discriminate among granite associations or series and to constrain evolutionary pathways and intensive crystallisation parameters during the evolutionary history of the host rocks (Gualda and Vlach, Reference Gualda and Vlach2007a,Reference Gualda and Vlachb,Reference Gualda and Vlachc; Vilalva and Vlach, Reference Vilalva and Vlach2014; Vilalva et al., Reference Vilalva, Vlach and Simonetti2016 and references therein).
As a part of a research program to examine the mineralogy of the granites and related rocks in the province in detail, this contribution aims to provide quantitative mineralogical information for the main granite types of the Mandira Massif, which was previously lacking. We present the main textural characteristics and compositional variations (major and minor elements) for magmatic and post-magmatic amphibole-group minerals, biotite, chlorite and stilpnomelane in the main granites and related greisens. Trace and REE compositions were also obtained and examined for selected calcic, sodic–calcic and sodic amphiboles. These data, together with whole-rock geochemical information, are used to draw inferences on mineralogical evolution and crystallisation conditions (P, T, $f_{{\rm O}_ 2}$) of the host granites and greisens. Furthermore, they are used to estimate REE coefficient partitions for the typical primary amphiboles, then examined using the Lattice Strain Model (Blundy and Wood, Reference Blundy and Wood1994, Reference Blundy and Wood2003), and to estimate halogen (F, Cl) fugacity ratios on the basis of biotite compositions.
The Mandira Granite Massif
The Mandira Granite Massif is one of several Neoproterozoic occurrences in the A-type Graciosa Province in S-SE Brazil (Fig. 1a), and was emplaced during a post-collisional extensional regime associated with the final stage of the Brasiliano/Pan-African Orogeny in southern Brazil at ca. 580 Ma (Vlach et al., Reference Vlach, Siga, Harara, Gualda, Basei and Vilalva2011; Vilalva et al., Reference Vilalva, Simonetti and Vlach2019). This province comprises several A-type granites and syenite plutons, and related volcanic and subvolcanic mafic and silicic rocks, together with K-rich diorites, gabbros and hybrid rocks. A-type granites and syenites in the province are grouped broadly into two petrological associations, one alkaline and the other aluminous (Gualda and Vlach, Reference Gualda and Vlach2007a). The alkaline association includes mainly metaluminous alkali-feldspar syenites, quartz syenites and peralkaline hypersolvus alkali-feldspar granites, whereas the aluminous association comprises metaluminous to moderately peraluminous subsolvus syeno- and monzogranites (Gualda and Vlach, Reference Gualda and Vlach2007a,Reference Gualda and Vlachb).
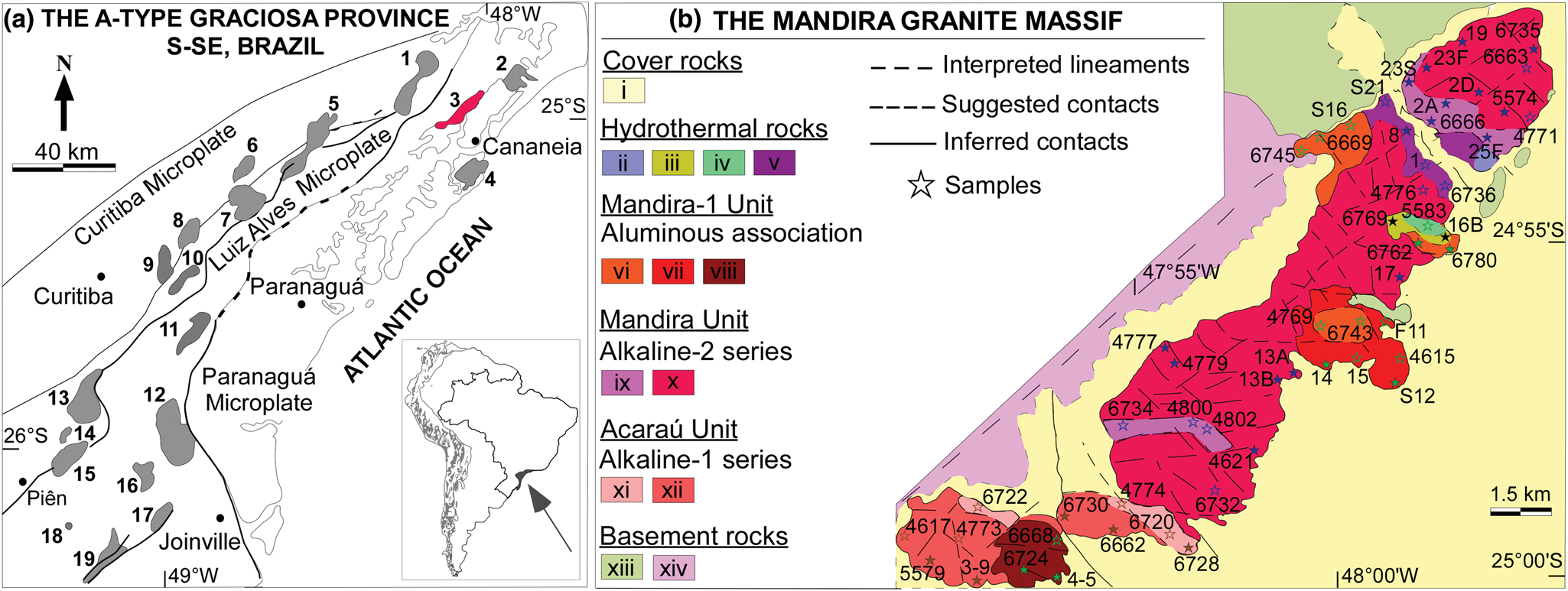
Fig. 1. Geology and setting of the granitic massif. (a) Geological setting and main units of the Neoproterozoic A-type Graciosa province in S-SE Brazil (Gualda and Vlach, Reference Gualda and Vlach2007a; Vilalva et al., Reference Vilalva, Simonetti and Vlach2019). (1) Guaraú Massif; (2) Serra do Paratiú/Cordeiro; (3) Mandira Massif; (4) Ilha do Cardoso; (5) Alto Turvo; (6) Capivari; (7) Órgãos; (8) Farinha Seca; (9) Anhangava; (10) Marumbi; (11) Serra da Igreja; (12) Morro Redondo Complex; (13) Palermo; (14) Agudos do Sul; (15) Rio Negro; (16) Dona Francisca; (17) Piraí; (18) Serra Alta; and (19) Corupá. (b) Geological map of the Mandira Granite Massif (adapted from Oliveira, Reference Oliveira1989). Legend: alluvial sediments (i); hydrothermal rocks (ii: veins, lenses, and pockets; iii: greisens and other metassomatites; iv: albitised granites; v: hydrothermal zone); aluminous granite facies (vi: inequigranular; vii: rapakivi; viii: porphyritic); alkaline-2 granite facies: (ix: porphyritic; x: inequigranular); alkaline-1 granite facies (xi: granophyric, xii: inequigranular); basement rocks (xiii: Turvo-Cajati Formation; xiv: Atuba Complex).
The Mandira massif crops out at the north-eastern region of the province along a N40°E–SW trend, with an exposure area of ~50 km2 (Fig. 1b). It intrudes orthogneisses and metasedimentary sequences of the Atuba Complex and the Turvo–Cajati Formation, respectively (Faleiros et al., Reference Faleiros, Campanha, Martins, Vlach and Vasconcelos2011), and is covered by Quaternary alluvial deposits along the Itapitangui River. Field work and representative fresh sample collection is very difficult in the exposure areas due to the extensive vegetation cover of the Atlantic Forest, and intense and deep weathering. Oliveira (Reference Oliveira1989) presented the first detailed study on the massif. On the basis of field mapping, petrography, and compositional data, Oliveira recognised the A-type nature of the granitic rocks and subdivided the massif into three main granite units: Acaraú and Mandira, which are alkali-feldspar granites, and the Mandira-1 unit, consisting of syenogranites.
The available geological map (Fig. 1b) suggests that these units should represent individual intrusions, plutons or stocks, as reported for other Graciosa occurrences (Gualda and Vlach, Reference Gualda and Vlach2007a; Vilalva and Vlach, Reference Vilalva and Vlach2014; Siachoque et al., Reference Siachoque, Garcia and Vlach2020); however structural relationships among them are not known and the suggested geological contacts are inferred, due to poor outcrop conditions. Gualda and Vlach (Reference Gualda and Vlach2007b) recognised contrasting magmatic series within the main alkaline and aluminous associations in the Serra da Graciosa occurrences in the province. Here we adopt a similar approach to subdivide the distinct alkali-feldspar granites from the Acaraú and Mandira units into two series, alkaline-1 and alkaline-2, respectively, whereas the syenogranites from the Mandira-1 unit are assigned to a single aluminous association. These units are relatively homogeneous from a petrographic perspective, however, each presents particular textural and mineralogical features, as well as a distinct crystallisation order inferred from textural analysis described briefly (see details in Oliveira, Reference Oliveira1989; Siachoque, Reference Siachoque2020; and our ‘Sampling and methods’ section), in the following sections. The available quantitative modal data are represented in Fig. 2 and our new modal data listed in Supplementary table S1.
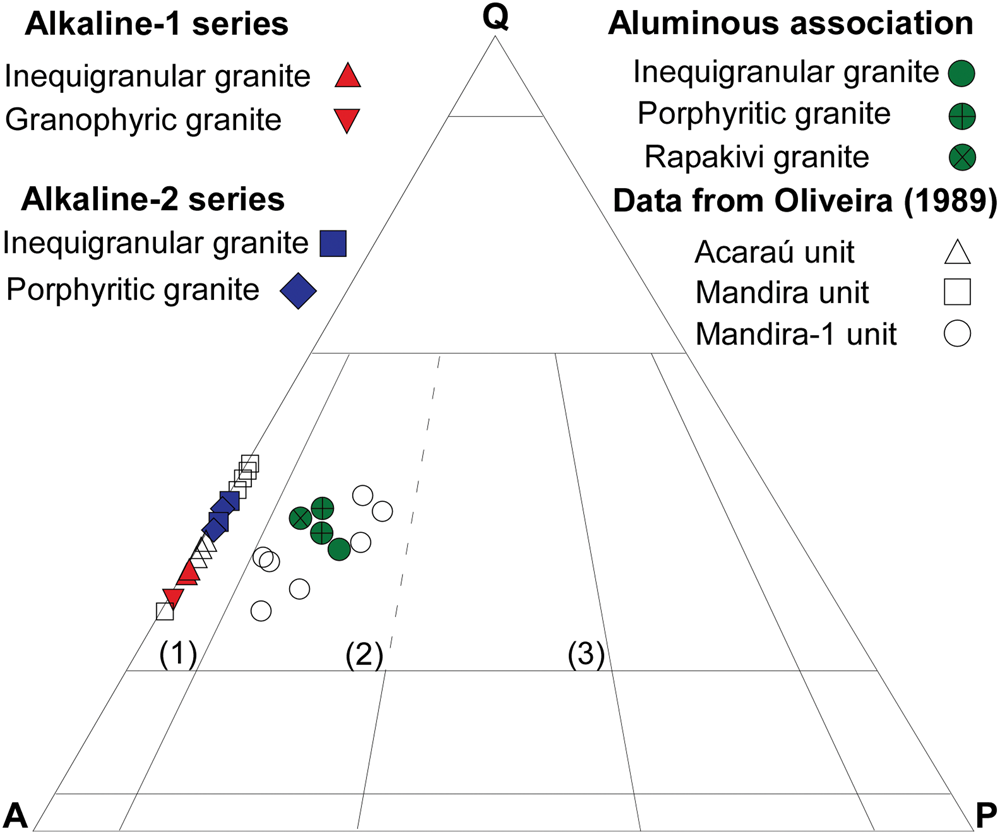
Fig. 2. QAP modal classification diagram (Streckeisen, Reference Streckeisen1976, see also Le Maitre et al., Reference Maitre, Streckeisen, Zanettin, Le Bas, Bonin and and Bateman2002) for the main granites from the Mandira Granite Massif. Numbered fields: (1) alkali-feldspar granite; (2) syenogranite; and (3) monzogranite.
Alkaline-1 series
The rocks from this series constitute a small pluton or stock (~8 km2) in the southernmost area of the massif (Fig. 1b). They are hypersolvus alkali-feldspar granites (Fig. 2) and include two main varieties: (1) a predominant greyish- to greenish-coloured massive granite with medium- to coarse-grained and inequigranular texture (Fig. 3a,b); and (2) a spatially restricted, greyish coloured, fine- to medium-grained granite, with cataclastic structure and variably inequigranular, granophyric and locally porphyritic textures with alkali-feldspar megacrysts (Fig. 3c). They are composed principally of mesoperthite alkali feldspar, quartz, calcic and sodic–calcic amphiboles, accompanied by apatite, Fe–Ti oxides (magnetite and ilmenite), chevkinite-(Ce), titanite and zircon as typical accessory phases. Albite, forming interstitial aggregates and swapped rims over alkali feldspar, allanite-(Ce), fluorite-(Y), xenotime, stilpnomelane and hematite are relatively common post-magmatic phases.
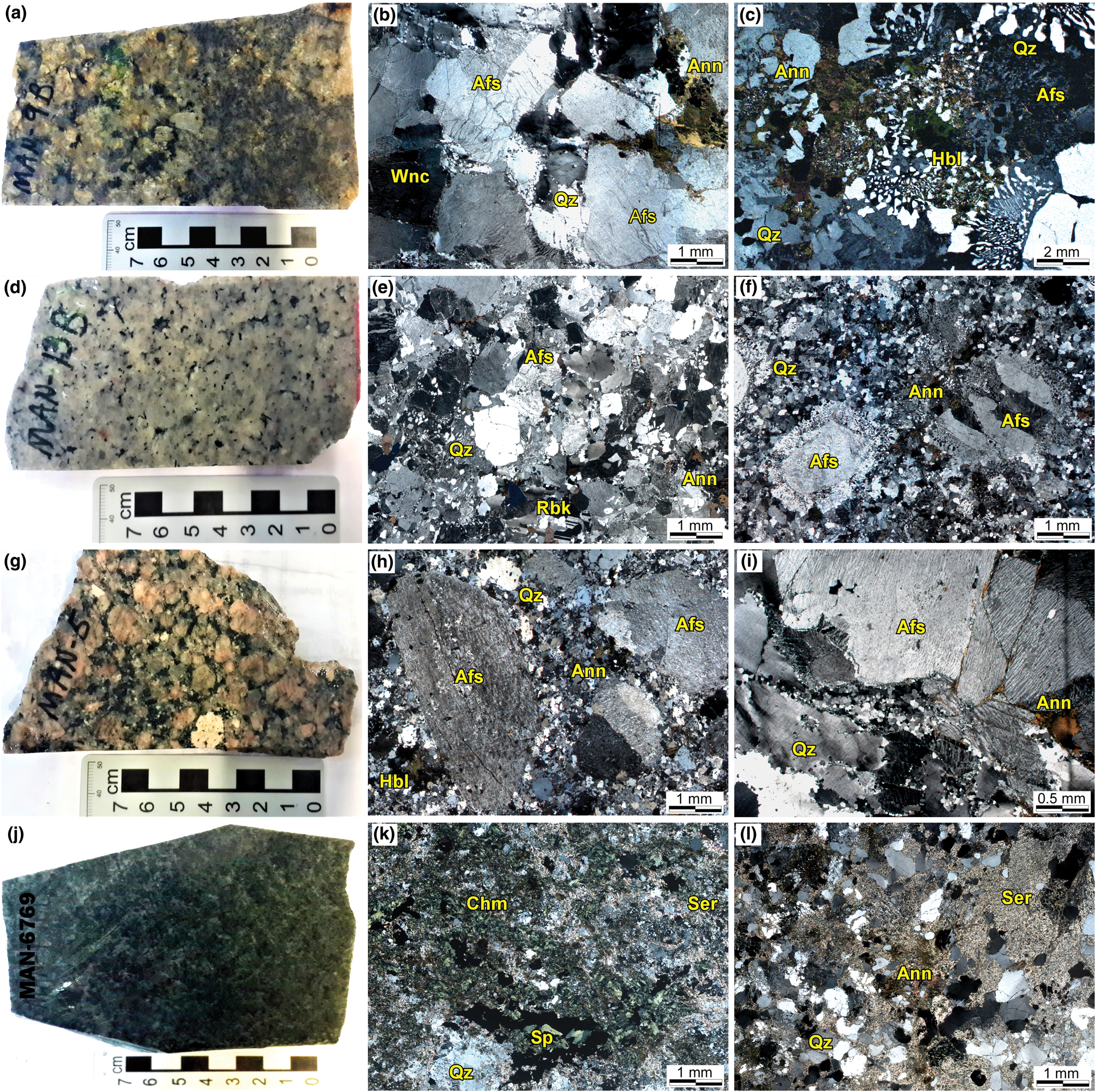
Fig. 3. Petrographic features in rock slabs and thin sections of the main granites and greisen from the Mandira Granite Massif. Photomicrographs obtained under plane polarised light: (a) massive greenish-coloured granite of the alkaline-1 association (Sample MAN-9A); (b) inequigranular granite (alkaline-1 series) showing medium- to coarse-grained texture with tabular mesoperthitic alkali feldspar (Afs), quartz (Qz), ferro-winchite (Wnc) and interstitial annite (Ann); (c) granophyric granite (alkaline-1 series) with abundant intergrowths between quartz and alkali feldspars and interstitial ferro-ferri-hornblende (Hbl) and annite; (d) massive greyish-coloured granite of the alkaline-2 series (Sample MAN-13A); (e) inequigranular granite (alkaline-2 series) with fine- to medium-grained texture presenting subhedral mesoperthitic alkali feldspar, quartz and interstitial riebeckite (Rbk) with aggregates of annite; (f) porphyritic granite (alkaline-2 series) with tabular megacrysts of alkali feldspars in a fine-grained matrix of quartz, alkali feldspar, albite and annite; (g) massive pinkish to slightly reddish-coloured granite of the aluminous association (Sample MAN-5); (h) porphyritic granite (aluminous association) with rounded megacrysts of alkali feldspars in a fine-grained matrix of recrystallised quartz, albite, ferro-ferri-hornblende and annite; (i) inequigranular granite (aluminous association) exhibiting cataclastic and solid-state deformations such as fractures along the alkali feldspars, sub-grain rotation and bulging recrystallisation of quartz; (j) greenish-coloured greisen associated with granites from the aluminous association (Sample MAN-6769); (k) greisen exhibiting heterogranoblastic medium- to fine-grained texture with quartz, chamosite (Chm), annite, sericite (Ser) and interstitial sphalerite (Sp); (l) quartz–sericite–mica greisen with heterogranoblastic fine- to medium-grained texture.
Alkaline-2 series
This series of alkali-feldspar granites forms the largest occurrence within the massif, cropping out over ~30 km2 and arguably comprises two distinct plutons inclined along the NE direction (Fig. 1b). The main granite is greyish coloured (Fig. 3d), has massive to slightly oriented structures, indicating some local sub-magmatic deformation, and a predominantly fine- to medium-grained inequigranular texture (Fig. 3e). A second variety is greyish coloured with a massive structure and medium- to coarse-grained porphyritic texture (Fig. 3f). This occurs in the outermost part of the northern pluton and in a restricted area of the central pluton (Fig. 1b). Both varieties are composed of mesoperthitic alkali feldspar, quartz, sodic amphiboles and biotite. Micrographic intergrowths between alkali feldspar and quartz are common in some samples. Astrophyllite, fluorite, ilmenite and zircon are typical accessories. In the porphyritic variety, astrophyllite is absent and discrete chalcopyrite, sphalerite and an unidentified Nb-bearing phase were observed. Albite, fluorite, fluocerite-(Ce), gagarinite-(Y), thorite and hematite were observed as post-magmatic phases.
Aluminous association
Granites of this association crop out as four distinct stocks distributed along the massif (Fig. 1b), covering a total area of ~12 km2. The southern stock within the massif is characterised by a pinkish- to slightly reddish-coloured massive granite with medium- to coarse-grained porphyritic texture (Fig. 3g,h). The central stock includes: (1) a greyish-coloured massive granite with fine- to medium-grained inequigranular texture; and (2) a pinkish-coloured massive granite with medium- to coarse-grained rapakivi texture. The northern stock is composed of a greyish- to whitish-coloured massive granite with variably fine-grained inequigranular and granophyric textures, affected significantly by hydrothermal processes leading to its transformation into a variety of hydrothermal rocks (Fig. 1b). The north-western stock is a greyish- to slightly pinkish-coloured granite with minor cataclastic structure and a fine- to medium-grained inequigranular texture (Fig. 3i). All types are syenogranites composed of perthitic alkali feldspar, quartz and subordinate sodic plagioclase (Fig. 2). Calcic amphibole and biotite are the main mafic minerals in the porphyritic granite, whereas in the rapakivi and inequigranular varieties, biotite is the sole mafic phase. Accessory minerals are apatite, allanite-(Ce), magnetite, ilmenite and zircon. Chlorite, epidote, sericite, albite, fluorite and stilpnomelane are common post-magmatic phases.
Hydrothermal rocks
Hydrothermal rocks include albitised granites, greisens, metasomatites, veins, lenses and pockets which are associated mainly with the northern granites from the alkaline-2 series and the granites from the aluminous association (see detailed descriptions in Oliveira, Reference Oliveira1989). We selected representative greisen samples for this study which include greenish-coloured chlorite–sphalerite greisens and quartz–mica greisens with fine-grained heterogranoblastic textures (Fig. 3j,k,l), composed mainly of quartz, chlorite, sericite and some biotite. The main accessory phases are chalcopyrite, sphalerite, pyrite, fluorite, galena, monazite, cassiterite, topaz, thorite, xenotime and zircon.
Sampling and methods
Thirty three representative samples were selected for this study (see location in Fig. 1b). Dra. M.C.B. Oliveira provided most of the samples as thin-section-sized rock slabs, and we collected others in typical and accessible outcrops of the massif. They include 22 samples from the alkaline association (5 and 17 from the alkaline-1 and alkaline-2 series, respectively), 9 from the aluminous association, and 2 from the most typical greisens. The analytical work was undertaken in the laboratories of the GeoAnalitica core facility at the Institute of Geosciences in the University of São Paulo, Brazil.
Analytical procedures and data treatment
All samples were examined as polished thin sections, 30 and 60 μm in thickness, with a petrographic microscope using transmitted and reflected light. Subsequently, sections were carbon-coated for energy-dispersive spectrometry (EDS) and investigated using back-scattered electron (BSE) imaging and simultaneous qualitative spot analysis. The objectives of analyses were identification of rock textures and the main mineral assemblages. We used textural, mineral stability and mineral paragenetic criteria to identify the main magmatic, late magmatic and post-magmatic minerals phases, and to infer their crystallisation order. In this paper, the term post-magmatic refers to all subsolidus processes affecting the primary (magmatic) minerals, or leading to the precipitation of new stable minerals directly from the fluid phase and, therefore it includes hydrothermal processes. However, determining processes and mineral formation during the late- and/or post-magmatic stages is a difficult task in some cases, and for these cases we use the generic term late- to post-magmatic.
The BSE images were used to select spots for quantitative analyses by wavelength-dispersive spectrometry (WDS). The work was carried out with conventional JEOL JXA–8600S and a field emission JEOL JXA–FE–8530 electron probe microanalysers (EPMA). Quantitative spot analysis was undertaken with 15 kV, 20 nA, and 5 μm for the column accelerating voltage, beam current and diameter, respectively. Total counting times, equally distributed for peak and background readings, varied from 10 s (major and light elements) to 40 s (minor elements). The analytical routines are similar to those presented by Gualda and Vlach (Reference Gualda and Vlach2007b) and we used natural and synthetic standards from both the Smithsonian and the GellerTM collections: hornblende (Si and Al in amphiboles), diopside (Si and Mg in phyllosilicates, Mg in amphiboles), fayalite (Fe and Mn), anorthoclase (Al), wollastonite (Ca), orthoclase (K), rutile (Ti), albite (Na), zircon (Zr), willemite (Zn), benitoite (Ba), sodalite (Cl) and fluorapatite (F). Matrix effect corrections and conversions of the raw data to concentrations were performed with the PROZA and PRZ/Armstrong software, available with the 8600S and FE-8530 microprobes, respectively. For chlorite and stilpnomelane analyses, a fixed close-to-stoichiometry H2O content was added to improve the absorption corrections. The estimated analytical errors are ≤ 2% and between 5 to 10% for major and minor elements, respectively.
Amphibole-group mineral formulae were calculated considering O + OH + F + Cl = 24 atoms per formula unit (apfu) according to the IMA classification and nomenclature scheme (Hawthorne et al., Reference Hawthorne, Oberti, Harlow, Maresch, Martin, Schumacher and Welch2012; Oberti et al., Reference Oberti, Cannillo and Toscani2012) using the Excel spreadsheet of Locock (Reference Locock2014). In the case of sodic amphiboles, Li contents, measured by laser ablation inductively coupled plasma mass spectrometry (LA-ICP-MS), were considered where available in the computed formulae. Biotite formulae and classification are according to Rieder et al. (Reference Rieder, Cavazzini, Yakonov, Frank-kamenetskii, Gottardi, Guggenheim, Koval, Muller, Neiva, Radoslovich, Robert, Sassi, Takeda, Weiss and Wones1998), whereas the schemes suggested by Zang and Fyfe (Reference Zang and Fyfe1995), Guggenheim et al. (Reference Guggenheim, Adams, Bain, Bergaya, Brigatti, Drits, Formoso, Galan, Kogure and Stanjek2007) and Yavuz et al. (Reference Yavuz, Kumral, Karakaya, Karakaya and Yildirim2015), and those by Hutton (Reference Hutton1938) and Malczewski and Popiel (Reference Malczewski and Popiel2008) were applied for chlorite and stilpnomelane, respectively.
The LA-ICP-MS analyses were performed on amphibole-group minerals from selected samples, using both spot and raster beam modes. The equipment used was a Thermo Fisher iCAP Q instrument coupled with a New Wave 213 nm Y(Nd)AlG A/F laser system. The analytical conditions were a laser spot of 55 μm (raster) or 80 μm (spot), under a frequency of 15 Hz and an energy fluence of 2.14 J/cm2. The total acquisition time was 100 s, divided between 60 s for samples and 40 s for blank measurements. The NIST SRM 610 glass was used as the primary standard and the previous WDS results for Si and Ca were used as internal references. Data reduction was carried out using Glitter software (Griffin et al., Reference Griffin, Powell, Pearson, O'Reilly and Sylvester2008). Detection limits, accuracy and precision are reported with analytical results in the Supplementary materials (see below).
Seven fresh samples, including three representative samples of both alkaline series, three of the aluminous association, and one of the greisen, were selected for whole-rock geochemistry. Samples were cleaned with deionised water, crushed with a stainless-steel jaw crusher and split; one aliquot was then powdered with an agate mill. Major, minor and some trace elements were quantified using fused and pressed pellets, respectively, by X-ray fluorescence (XRF) spectrometry using PANanalytical AXIOS MAX Advanced equipment, following the procedure described by Mori et al. (Reference Mori, Reeves, Correia and Haukka1999). Other trace elements, including the REE, were analysed by ICP-MS using a Thermo Fisher iCAP Q instrument. Detailed analytical procedures, including instrumental and data acquisition parameters, sample digestion and preparation are described in Navarro et al. (Reference Navarro, Andrade, Ulbrich, Gomes and Girardi2008).
Results
Mineral distributions and textures
A remarkable textural contrast between the peralkaline and the metaluminous to slightly peraluminous granites from the alkaline series and the aluminous association, respectively, is their quite different crystallisation sequence (e.g. Gualda and Vlach, Reference Gualda and Vlach2007b; Vilalva and Vlach, Reference Vilalva and Vlach2014). In the former, the primary calcic, sodic–calcic, and sodic amphiboles appear typically interstitial to the alkali feldspar and quartz and certainly began crystallising after significant volumes of feldspar and quartz . This crystallisation pattern, in which the main mafic minerals began to crystallise later than the main felsic minerals, is known as the agpaitic crystallisation order or sequence, as defined initially for SiO2-undersatured agpaitic rocks (e.g. Sørensen, Reference Sørensen1997), and is not observed for the syenogranites from the aluminous association.
Amphibole-group minerals
In the alkaline-1 series, the primary calcic and sodic–calcic amphiboles occur as isolated crystals or as groups of a few crystals. They form either euhedral-to-subhedral (1–3 mm) crystals with prismatic habit and brownish–green pleochroism (Fig. 4a), or euhedral-to-anhedral large and elongated crystals (4–6 mm) with dark green to blue pleochroism, and several inclusions of zircon, chevkinite and ilmenite (Fig. 4b). Post-magmatic amphiboles include a colourless to pale green actinolite, which occurs mainly as thin overgrowths on some calcic amphibole crystals, and a sodic amphibole with indigo blue to greenish pleochroism, forming smaller acicular crystals, replacing the primary amphiboles along rims or fractures in the granophyric granites (Fig. 4c).
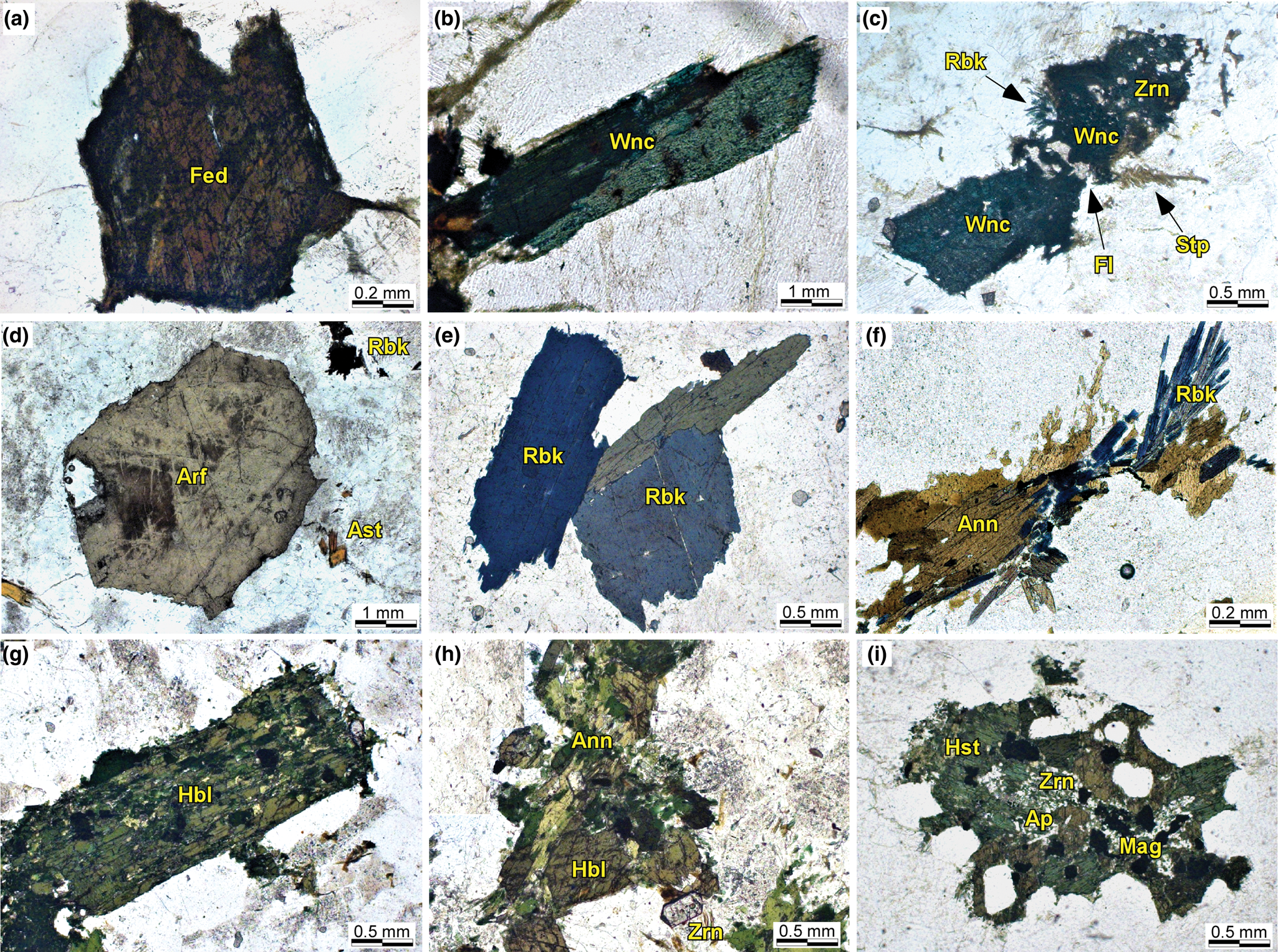
Fig. 4. Microtextural features of amphiboles in granites from the Mandira Granite Massif. Photomicrographs with plane polarised light: (a) subhedral ferro-edenite (Fed) with subtle patch zoning in granophyre granite (alkaline-1 series, Sample MAN-6728); (b) euhedral winchite (Wnc) showing zoning pattern in inequigranular granite (alkaline-1 series, Sample MAN-9A); (c) subhedral-to-anhedral winchite with zircon inclusions, partially altered and replaced by riebeckite and stilpnomelane (Stp) along margins in inequigranular granite (alkaline-1 series, Sample MAN-5579); (d) euhedral prismatic arfvedsonite (Arf) interstitial to alkali-feldspar crystals in inequigranular granite (alkaline-2 series, Sample MAN-4777); (e) large subhedral riebeckite (Rbk) with sharp terminations in inequigranular granite (alkaline-2 series, Sample MAN-13A); (f) fibrous secondary riebeckite intergrown with annite (Ann) between felsic minerals in inequigranular granite (alkaline-2 series, Sample MAN-5574); (g) euhedral prismatic hornblende (Hbl) with abundant inclusions of magnetite (Mag) in porphyritic granite (aluminous association, Sample MAN-5); (h) subhedral hornblende replaced partially by annite along cleavage planes and fractures in porphyritic granite (aluminous association, Sample MAN-5); (i) hastingsite (Hst) with abundant inclusions of apatite (Ap), magnetite and zircon (Zrn); crystal cores are partially altered (porphyritic granites – aluminous association, Sample MAN-6724).
In the alkaline-2 series, the sodic amphiboles crystallised either as isolated large euhedral prismatic crystals (up to 5 mm), with brownish–bluish pleochroism (Fig. 4d), or as smaller euhedral-to-subhedral crystals (up to 2 mm) with dominant dark bluish colours (Fig. 4e). Sometimes the crystals exhibit zircon inclusions and subtle irregular zoning patterns in BSE images (not shown). A distinct post-magmatic generation comprises smaller-to-minute bluish acicular crystals or aggregates of fibrous, very thin crystals, associated with biotite (Fig. 4f).
In the porphyritic granites from the aluminous association, calcic amphiboles occur as large euhedral prismatic crystals (2–4 mm), with dark green to yellowish pleochroism and local Fe–Ti oxides along their cleavages (Fig. 4g). Some grains are partly replaced by magmatic biotite of late crystallisation (Fig. 4h). A distinct Ca-amphibole occurs in some rocks as subhedral-to-anhedral medium-grained (1–2 mm) dark brown–green coloured crystals. They show some alteration to chlorite, epidote and opaque minerals and contain inclusions of apatite, zircon, magnetite and quartz (Fig. 4i).
Biotite
In the alkaline series the main biotite, as is typical for the inequigranular granites, forms subhedral-to-anhedral, individual, or grouped small (2–4 mm) crystals with brownish orange pleochroism with inclusions of zircon, ilmenite and fluorite. It is preferentially associated with the sodic–calcic amphibole and is partially-mantled by acicular riebeckite of post-magmatic origin (Fig. 5a,b). It is not clear whether such crystals precipitated during the late- or post-magmatic stage, or represent a transition between both, thus they are considered as late- to post-magmatic. A distinct type of post-magmatic biotite occurs solely in the porphyritic granites from the alkaline-2 series. This is characterised by small euhedral-to-subhedral (up to 1 mm) zoned crystals with a brownish green to pale green pleochroism, and occurs in association with fluorite and hematite (Fig. 5c).
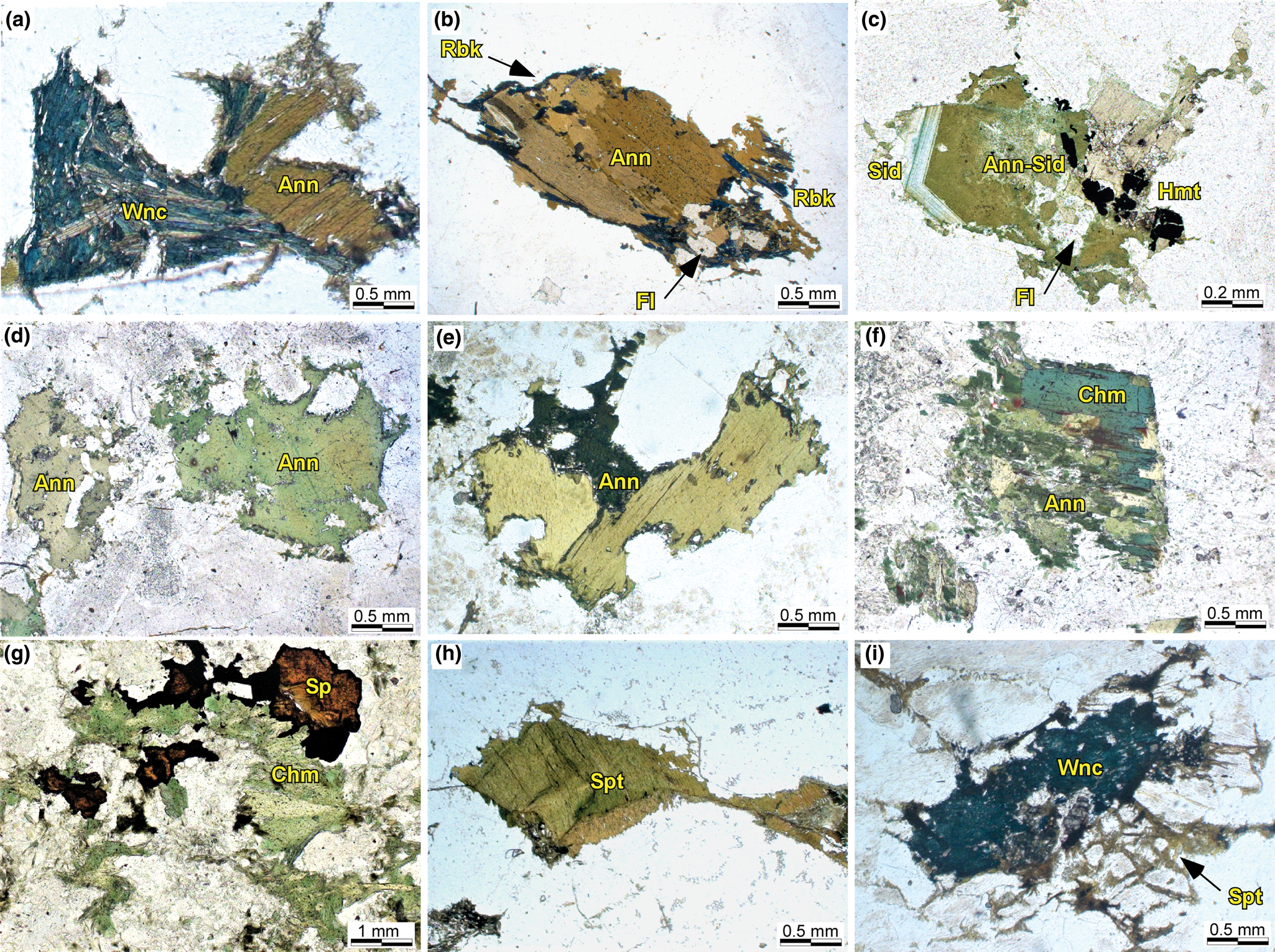
Fig. 5. Microtextural features of phyllosilicates in samples of the Mandira Granite Massif as observed in thin sections. Photomicrographs with plane polarised light: (a) interstitial annite (Ann) in relationship with winchite in inequigranular granite (alkaline-1 series, Sample MAN-9A); (b) subhedral platy annite surrounded by secondary riebeckite and inclusions of fluorite in inequigranular granite (alkaline-2 series, Sample MAN-5574); (c) zoned annite–siderophyllite (Ann–Sid) associated with fluorite (Fl) and hematite (Hem); crystal shows corroded rims and partially altered core in porphyritic granite (alkaline-2 series, Sample MAN-6666); (d) subhedral-to-anhedral annite interstitial to alkali feldspars and quartz in porphyritic granite (aluminous association, Sample MAN-5); (e) annite crystal cluster showing corroded rims in rapakivi granite (aluminous association, Sample MAN-14); (f) chamosite (Chm) replaces (full and partially) annite in inequigranular syenogranite (aluminous association, Sample MAN-5); (g) irregular chlorite and sphalerite (Sp) forming aggregates in quartz–sphalerite–sericite greisen (Sample MAN-6769); (h) anhedral stilpnomelane (Spt) displaying well-developed cleavage and irregular rims in granophyre granite (alkaline-1 series, Sample MAN-6730); (i) fine-grained fibrous stilpnomelane interstitial to feldspar and partially replacing winchite (alkaline-1 series, Sample MAN-9).
In porphyritic granites of the aluminous association, biotite is present as isolated, subhedral-to-anhedral crystals (3–6 mm), with dark green colours (Fig. 5d). This began to crystallise after the amphibole and sometimes partially replaces it (Fig. 4h). The rapakivi granites contain biotite crystals (2–5 mm in size) with pale to dark green pleochroism, including tiny apatite, zircon and magnetite crystals (Fig. 5e), whereas the inequigranular granites present biotite as irregular crystals with pale yellow to green pleochroism, which are more commonly replaced by chlorite (Fig. 5f)
Chlorite and stilpnomelane
Chlorite is one of the most common hydrothermal phases in the aluminous granites and related greisens. In the granites, chlorite occurs as dark green crystals partially or fully replacing biotite (Fig. 5f), or as crystals associated with sericite and epidote. In the greisens chlorite forms pale-green platy crystal intergrowths, distributed homogeneously within the groundmass and associated usually with sphalerite and chalcopyrite (Fig. 5g).
Stilpnomelane is observed occasionally in samples from the alkaline-1 series and the aluminous association. In the first case, it occurs either as isolated large interstitial crystals (up to 5 mm) displaying platy or fibrous-radiating habits, with strong dark brown to pale green pleochroism (Fig. 5h), or as brownish acicular crystals partially replacing late-magmatic amphiboles at their rims (Fig. 5i). In the aluminous association, stilpnomelane forms fine-grained fibrous brown crystal aggregates, distributed along fractures of primary feldspar crystals (not shown).
Mineral compositional variation
Representative WDS compositions and structural formulae for the mafic minerals along with LA-ICP-MS data for trace elements in amphiboles, are presented in Tables 1 to 5 and discussed in the following sections. The complete WDS and LA-ICP-MS data sets, with ~410 mineral analyses for the studied Mandira granite samples are presented in Supplementary tables S2 to S6.
Table 1. Representative compositions (WDS) and structural formulae for amphiboles from alkaline series and the aluminous association of the Mandira Granite Massif.

Notes: Li2O* from LA-ICP-MS measurements; Fe# = FeT/(FeT+Mg); FeT = Fe3+ + Fe2+; n.a.: not analysed; n.d.: not detected.
Amphibole
Main compositional variations
The compositions of the Mandira amphiboles vary widely (Table 1, Supplementary table S2). The calcic varieties are ferro-edenite and ferro-ferri-hornblende in the alkaline-1 series and ferro-ferri-hornblende and hastingsite in the aluminous association; ferro-ferri-winchite is the sodic–calcic amphibole exclusive to the alkaline-1 series, whereas arfvedsonite and riebeckite are the main magmatic sodic amphiboles in the alkaline-2 rocks. The post-magmatic calcic and sodic amphiboles in the alkaline series are ferro-actinolite and riebeckite (Fig. 6).

Fig. 6. Classification of amphiboles in the Mandira granites according to Hawthorne et al. (Reference Hawthorne, Oberti, Harlow, Maresch, Martin, Schumacher and Welch2012).
As compared to calcic amphiboles from the alkaline-1 series, the calcic amphiboles in the aluminous association are confirmed by the average relatively higher values of Ca (up to 1.75 vs. 1.61 apfu), Al (up to 1.62 vs. 0.93 apfu) and Ti (up to 0.23 vs. 0.15 apfu) in their structural formulae. The Fe3+/FeT (where FeT =Fe3++Fe2+) stoichiometry estimated ratios are similar: up to 0.20 (ferro-edenite and ferro-ferri-hornblende) in the alkaline-1 series and up to 0.24 (ferro-ferri-hornblende and hastingsite) in the aluminous association (Fig. 7a).

Fig. 7. Main compositional trends for the amphiboles from the Mandira granites: (a) Fe3+/ (Fe3+ + Fe2+) vs. Si + Na + K diagram; (b) (Na + K)/Al vs. Si + Na + K diagram. The suggested main coupled substitution schemes (in apfu): (c) [vacancy]A + [Fe3+]C vs. [Na,K]A + [Fe2+]C (Strong and Taylor, Reference Strong and Taylor1984); (d) 3[Al, Fe3+]C + Si vs. 2[Ti, Zr]C + [Mg,Fe2+,Mn,Zn]C + [Al]T (Vilalva et al., Reference Vilalva, Vlach and Simonetti2016). Symbols as in Fig. 6.
The ferro-ferri-winchite contains up to 1.49 and 1.31 apfu Ca and Na, with Ca > Na in the B (M4) sites; Al and Ti contents are up to 0.80 and 0.18 apfu, respectively. Core to rim compositional variations in zoned crystals show increasing Na, K, Al and Fe3+ values from 0.52 to 1.20, 0.03 to 0.19, 0.23 to 0.80, and 0.29 to 0.97 apfu, respectively, as represented by the variations of the Fe3+/FeT and peralkaline [(Na+K)/Al] ratios with Si + Na + K values (Fig. 7a,b).
Comparing the late-magmatic sodic varieties (see also Supplementary table S2), arfvedsonite has higher values (apfu) of Na (up to 2.31), F (up to 1.15) relative to riebeckite with values of 2.28 Na, 0.79 F and, as expected, lower Fe3+, up to 1.60 (arfvedsonite) vs. up to 1.90 (riebeckite). As a rule, both amphiboles show increasing [(Na+K)/Al] and Fe3+/FeT ratios from crystal cores to rims: 6.11 to 17.95 and 0.09 to 0.35 (arfvedsonite) and 8.11 to 15.38 and 0.26 to 0.41 (riebeckite), respectively (Fig. 7a,b). The post-magmatic riebeckite exhibits the highest [(Na+K)/Al] ratio, up to 30.53 apfu, and Fe3+/FeT ratios up to 0.50 (Fig. 7a,b).
The main compositional variations in the Mandira amphiboles can be explained by the following coupled reactions (Fig. 7c,d):


where reaction (1) represents the substitution of Fe3+ for Fe2+ (e.g. Strong and Taylor, Reference Strong and Taylor1984) and reaction (2) accounts for substitutions of Ti and Zr occurring in the amphibole C-site (e.g. Vilalva et al., Reference Vilalva, Vlach and Simonetti2016). Except for reaction (1) for calcic amphiboles from the aluminous association, which have a relatively lower but still significant correlation coefficient (r 2) of 0.59, all other cases have r 2 ≥ 0.90.
Trace-element and REE compositions
Multi-element and REE distribution patterns for the amphiboles are depicted in Fig. 8. Trace-element distribution patterns show variable but strong negative Sr, Ti and V–Co anomalies, and slightly positive Rb, Nb and Sn anomalies for all amphiboles. In addition to the REE (discussed further below), some distinctive features are the positive Ta anomaly (up to 105 ppm, Fig. 8a) for calcic and sodic–calcic varieties from the alkaline-1 series, the significant Li abundances (up to 2533 ppm, Fig. 8c) in sodic–calcic and sodic amphiboles from both alkaline series, and the slightly positive Hf anomaly (up to 45 ppm, Fig. 8c) in the sodic amphiboles of the alkaline-2 series. Compared to the alkaline series, calcic amphiboles in the aluminous association are relatively depleted in Zr (107 vs. 306 ppm, respectively: Fig. 8a,e), as well as exhibiting a distinctive negative Pb anomaly (Fig. 8e).

Fig. 8. Trace element (a), (c), (e) and REE (b), (d), (f) distribution patterns for amphiboles from the Mandira granites normalised to the primitive mantle and chondrite values of McDonough and Sun (Reference McDonough and Sun1995), respectively. Grey-bars in (a), (c) and (e) represent distinctive anomalies between granites such as Ta (alkaline-1 series), Li (alkaline-1 and 2 series) and Zr (alkaline-1 series and aluminous association).
The REE distribution patterns show distinct behaviour between and within amphibole groups (Fig. 8b,d,f). Typically, total REE abundances decrease from calcic (up to 2665 ppm), to sodic–calcic (up to 524 ppm), and sodic varieties (up to 245 ppm) (see also Tables 2 and S3). Comaprison of calcic amphibole patterns for the alkaline-1 and aluminous granites shows that ferro-edenite in the alkaline-1 series displays significant fractionation of LREE relative to HREE (6.3 ≤ LaN/Yb/N ≤ 8.7), accompanied by a significant fractionation in both LREE and HREE, with 2.0 ≤ LaN/SmN ≤ 3.3 and 1.3 ≤ GdN/LuN ≤ 2.4, and a strong negative Eu anomaly, with Eu/Eu* = 0.11 [Eu* = (SmN*GdN)0.5]. In contrast, the ferro-ferri-hornblende and the hastingsite of the aluminous association exhibit LREE distribution patterns with a concave shape (0.33 ≤ LaN/SmN ≤ 1.38) and flat HREE patterns (0.9 ≤ GdN/LuN ≤ 2.1) exhibiting a subtle relative enrichment from Er to Lu; the Eu anomaly is similar, with Eu/Eu* reaching 0.09.
Table 2. Representative mean trace-element compositions (LA-ICP-MS) for amphiboles from alkaline series and the aluminous association of the Mandira Granite Massif.

Note: S.D. – standard deviation.
Ferro-ferri-winchite crystals display much lower REE and LREE/HREE fractionation (0.11 ≤ LaN/Yb/N ≤ 4.6), relatively flat LREE (0.11 ≤ LaN/SmN ≤ 1.64), and concave HREE patterns (0.5 ≤ GdN/LuN ≤ 1.4), with discrete steep enrichment from Er to Lu when compared to the ferro-edenite variety of the alkaline-1 series; the Eu anomaly is also less pronounced (Eu/Eu* = 0.06).
Magmatic arfvedsonite and riebeckite record similar REE distribution patterns, although riebeckite has higher REE content (up to 286 ppm). These patterns are relatively flat with some LREE depletion and HREE enrichment (0.07 ≤ GdN/YbN ≤ 0.34) and a positive slope from Ho to Lu; Eu/Eu* ranges from 0.04 to 0.11.
Biotite
The most significant compositional characteristics of biotite from the Mandira granites are summarised in Fig. 9 (see also Tables 3 and S4). Biotite in the granites from the aluminous association is annite with 0.10 ≤ VIAl ≤ 0.45 (Fig. 9a), 0.74 ≤ Fe# [= FeT/(FeT+Mg)] ≤ 0.87 and Ti varying from 0.03 to 0.14 apfu (Fig. 9b). Such compositions are markedly different from those observed in the alkaline series. In the main inequigranular granites the late- to post-magmatic crystals are annite, which has the highest Fe# (0.97 to 0.99) and the lowest VIAl (up to 0.15) contents of all mica varieties (Fig. 9a), with Ti contents in the range 0.01 to 0.18 apfu (Fig. 9b). In contrast, the post-magmatic biotite in the porphyritic granite of the alkaline-2 series has a greater VIAl variation, with 0.3 ≤ VIAl < 1.0, 0.93 ≤ Fe# ≤ 0.97 and Ti ≤ 0.15 apfu (Fig. 9a). In the typically zoned crystals, the crystal cores and rims are annite–siderophyllite and siderophyllite, respectively; the former being relatively richer in Fe and Ti (Fig. 9a,b).

Fig. 9. Classification and compositional variations of biotite varieties from the Mandira granites: (a) VIAl vs. Fe/(Fe + Mg) classification diagram; end-members according to Rieder et al. (Reference Rieder, Cavazzini, Yakonov, Frank-kamenetskii, Gottardi, Guggenheim, Koval, Muller, Neiva, Radoslovich, Robert, Sassi, Takeda, Weiss and Wones1998): (b) Ti vs. Fe/(Fe + Mg) plot; (c) F vs. Cl (wt.%) plot for biotite varieties, the inset shows the same plot for the amphibole-group minerals; (d) (Fe + Mg + Mn) + Ti vs. 2VIAl diagram to highlight the siderophyllite cationic substitution (Speer, Reference Speer and Bailey1984). All quantities in apfu, except in (c).
Table 3. Representative compositions (WDS) and structural formulae for biotite from the alkaline series and aluminous association of the Mandira Granite Massif.
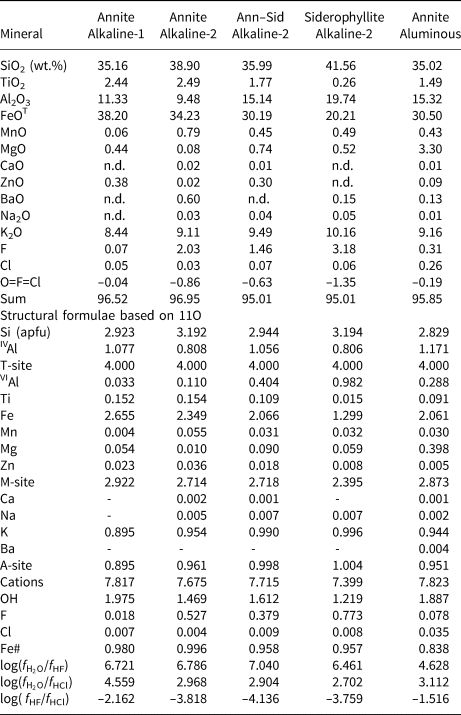
Notes: Fe# = FeT/(FeT+Mg); n.d. – not detected.
Fluorine and Cl contents show contrasting behaviour between the alkaline and aluminous associations. Annite from both alkaline series has the highest F contents among all biotite varieties, varying from 0.5 to 3.24 wt.%, (Fig. 9c), whereas the annite from the aluminous association is characterised by relatively high contents of Cl (up to 0.5 wt.%). The volatile contents and behaviour in biotite from all rocks are similar to those in amphibole-group minerals, as shown in the inset of Fig. 9c.
The main compositional variations of biotite can be described by the siderophyllite exchange vector (Speer, Reference Speer and Bailey1984) represented in Fig. 9d and the reaction:

Chlorite and stilpnomelane
The chlorite-group minerals (Table 4 and Supplementary table S5) are trioctahedral with relatively high Fe# ratios and classify as chamosite in both the rapakivi granite and the related greisen from the aluminous association. In each rock chamosite compositions are relatively homogeneous (Fig. 10a) and, comparing granite and greisen, VIAl + Fe3+ (apfu) and Fe# average values are 1.42, 1.50 and 0.87, 0.98, respectively. The main observed compositional variations are best explained by the coupled substitution:

as suggested by Zane et al. (Reference Zane, Sassi and Guidotti1998) and represented in Fig. 10b.

Fig. 10. Classification and compositional variations of the chlorite-group minerals from the Mandira granites and associated greisen: (a) VI[Al3++ Fe3+] vs. Fe/(Fe + Mg) classification diagram (Guggenheim et al., Reference Guggenheim, Adams, Bain, Bergaya, Brigatti, Drits, Formoso, Galan, Kogure and Stanjek2007); (b) IVAl + VIAl vs. Si + (Fe2+ + Mg) diagram illustrating the main cationic substitution trend, as suggested by Zane et al. (Reference Zane, Sassi and Guidotti1998). All quantities in apfu.
Table 4. Representative compositions (WDS) and structural formulae for chlorite (chamosite) from the aluminous association of the Mandira Granite Massif.

Notes: H2O* and Fe3+* computed by stoichiometry; Fe# = FeT/(FeT+Mg); n.d. – not detected.
The stilpnomelane crystals are ferri-stilpnomelane on the basis of their Fe3+/Fe2+ estimated ratios. Significant differences between the compositions for the alkaline-1 and aluminous associations suggest contrasted chemical signatures for the hydrothermal fluids associated with the respective magmas (Fig. 11a; Tables 5 and Supplemental table S6). Thus, in the aluminous porphyritic granite stilpnomelane records relatively higher AlT (from 1.32 to 1.56), Mg (from 0.48 to 0.64) and Mn (from 0.19 to 0.31) values (apfu) when compared to those observed in the inequigranular peralkaline granite, with ranges of 1.04–1.26, 0.72–0.15 and 10.15–0.22, respectively. As expected, the FeT contents are significantly higher in stilpnomelane from the alkaline-1 series (from 5.15 to 5.69) in comparison to those from the aluminous association sample (from 4.70 to 4.96). The compositional variations in the stilpnomelane structure can be described by the coupled substitution:

as suggested by Eggleton (Reference Eggleton1972) and represented in Fig. 11b.

Fig. 11. Main compositional variations in stilpnomelane from the Mandira granites: (a) AlT vs. FeT/Mn diagram; (b) IVAl +VIAl vs. Si + (Fe + Mn+ Mg) diagram (Zane et al., Reference Zane, Sassi and Guidotti1998). Note the contrasted compositions in the inequigranular and porphyritic granites. All quantities in apfu.
Table 5. Representative compositions (WDS) and structural formulae for ferri-stilpnomelane from the alkaline-1 series and the aluminous association of Mandira Granite Massif.

Notes: H2O* computed by stoichiometry; Fe# = FeT/(FeT+Mg); n.d. – not detected.
Whole-rock geochemistry
A brief geochemical characterisation of the rocks investigated is presented to support our mineralogical studies and the estimates of intensive crystallisation parameters. The compositions of the analysed granites and a related quartz–mica greisen are given in Table 6, together with CIPW norms for the intrusive rocks, and Table 7. The data are portrayed in selected geochemical diagrams and compared with other occurrences from the Graciosa Province in Fig. 12.

Fig. 12. Main geochemical characteristics of the Mandira granites: (a) Alumina Saturation Index [A/CNK = Al2O3/(CaO + Na2O + K2O) mol.] vs. Agpaitic Index [AI = (Na2O + K2O)/Al2O3 mol.]; (b) AI vs. Fe-number [Fe* = FeOT/(FeOT + MgO), wt.%]; (c) multi-trace-element and (d) REE patterns normalised to the primitive mantle and chondrite values of McDonough and Sun (Reference McDonough and Sun1995), respectively. (e) (Na2O+K2O)/CaO vs. Zr+Nb+Ce+Y diagram of Whalen et al. (Reference Whalen, Currie and Chappell1987). (f) Y–Nb–3*Ga ternary diagram for discriminating A1- and A2-type granites (Eby, Reference Eby1992). (g) Nb vs. Y tectonic discrimination diagram of Pearce et al. (Reference Pearce, Harris and Tindle1984). In (a), (b), (e), (f) and (g), our data is compared with those for typical occurrences in the Graciosa Province (1: Ilha do Cardoso; 2: Orgãos; 3: Farinha Seca; 4: Anhangava; 5: Papanduva; 6: Corupá; 7: Serra do Paratiú; 8: Capivari; 9: Orgãos; 10: Marumbi; 11: Quiriri; 12: Desemborque; data from Weber et al., Reference Weber, Basei, Siga and Sato2001; Garin, Reference Garin2002; Martins et al., Reference Martins, de Andrade e Silva and de Oliveira2004; Gualda and Vlach, Reference Gualda and Vlach2007a; Vilalva and Vlach, Reference Vilalva and Vlach2014; Garcia, Reference Garcia2015).
Table 6. Compositions (XRF) and CIPW norms for granites from the alkaline series, the aluminous association, and greisen of the Mandira Granite Massif.

Notes: n.d.: not detected; LOI: Lost on Ignition; Fe* = Fe number; A/CNK: Alumina Saturation Index; AI: Agpaitic (Peralkaline) Index; n.c.: not calculated.
Table 7. Trace-element compositions (ICP-MS) for granites from alkaline series and the aluminous associations of the Mandira Granite Massif.

The hypersolvus alkali-feldspar granites are peralkaline, with an agpaitic (peralkaline) index [AI = (Na2O+K2O)/Al2O3, molar] of ~1.03 (alkaline-1 series) and 1.03–1.05 (alkaline-2 series), and high Fe numbers [Fe* = FeOT/(FeOT + MgO) wt.% oxides], close to 1.0 (Fig. 12a,b). These are the highest Fe numbers observed in the Graciosa Province. The syenogranites from the aluminous association are metaluminous to slightly peraluminous with A/CNK [= Al2O3/(CaO+Na2O+K2O) molar] between 0.96 and 1.0, and 0.82 ≤ Fe* ≤ 0.89 (Fig. 12a,b). The quartz–mica greisen, when compared to its host syenogranite, has relatively higher Al2O3 and Fe2O3, with lower F, Na2O + K2O and K2O/Na2O. Contents of Zn are two orders of magnitude higher than the average values observed in the granites (Table 6).
The trace-element and REE distribution (Fig. 12c,d), exhibit a relative enrichment in the high-field-strength elements (HFSE, e.g. Nb, Zr, Hf), Th, U and Pb, and depletion in Ba, K, P and Ti, with variable negative anomalies. These are common features of the granites from the alkaline and aluminous association in the Graciosa Province (e.g. Vilalva and Vlach, Reference Vilalva and Vlach2014; Vilalva et al., Reference Vilalva, Simonetti and Vlach2019). Granites from the alkaline-1 series contain higher amounts of F, Zn and REE, being relatively enriched in most of the LREE compared to those from the alkaline-2 series, which, in turn, are relatively enriched in HREE. The syenogranites contain the highest REE abundances, with a strong LREE fractionation over the HREE.
When plotted on discriminating diagrams for granite types and their tectonic settings (Fig. 12e–g), as expected, our data corresponds to typical A-type granites according to Whalen et al. (Reference Whalen, Currie and Chappell1987) and plot as the A2-type of Eby (Reference Eby1992), in agreement with their post-collisional emplacement setting, and as ‘Within Plate Granites’, as defined by Pearce et al. (Reference Pearce, Harris and Tindle1984).
Discussion
Mineral constraints on crystallisation conditions
Pressure and temperature
The granites of the aluminous association exhibit the proper mineral assemblage, however, given the high Fe# values (~0.81) in calcic amphiboles, the Al-in-hornblende (e.g. Hammarstrom and Zen, Reference Hammarstrom and Zen1986; Mutch et al., Reference Mutch, Blundy, Tattitch, Cooper and Brooker2016) and the hornblende–plagioclase barometers (e.g. Blundy and Holland, Reference Blundy and Holland1990; Molina et al., Reference Molina, Moreno, Castro, Rodríguez and Fershtater2015) give overestimated pressures (up to 350–400 MPa) and are not applicable (e.g. Anderson and Smith, Reference Anderson and Smith1995) for these samples. Roughly alternative estimates may be extracted from the normative Qz–Ab–Or ternary diagram (not shown), as well as the empirical equations proposed by Yang (Reference Yang2017):


where Qz, Ab and Or are the CIPW normative quartz, albite and orthoclase values recalculated to 100 wt.% and P is given in MPa. These equations are applicable for peralkaline, metaluminous, and peraluminous granites with relatively low mafic minerals contents and a sodic plagioclase, when this phase is present. The pressures obtained average 177 ± 4 MPa and 93 ± 22 MPa for the alkaline-1 and alkaline-2 series, respectively, and 195 ± 4 MPa for the aluminous association. We have no geological arguments supporting these differences, however, which are ascribable to some trapped quartz or alkali-feldspar crystals and/or contrasted volatile contents in the emplaced magmas. Regardless, they indicate relatively shallow emplacement levels for the Mandira intrusions (in-between 3 and 7 km), which are compatible with the general geological setting, occurrence of hypersolvus granites and textures (e.g. micrographic intergrowths of alkali-feldspar and quartz), as inferred for the other intrusions in the Graciosa Province (e.g. Gualda and Vlach, Reference Gualda and Vlach2007a; Vilalva and Vlach, Reference Vilalva and Vlach2014).
Temperatures were estimated for both magmatic and hydrothermal evolutionary stages of the Mandira granites. Zircon and apatite saturation temperatures (Watson and Harrison, Reference Watson and Harrison1983; Harrison and Watson, Reference Harrison and Watson1984) for samples from the aluminous association range from 793 to 884°C and from 806 to 865°C, with similar averages of 846°C and 828°C, respectively, which are our best estimates for close-to-liquidus temperatures, as rock textures indicate that these minerals were among the first phases that precipitated from the magmas. The hornblende–plagioclase equilibrium temperatures (Holland and Blundy, Reference Holland and Blundy1994) for the porphyritic granite in this association give values from 754 to 779°C, averaging 776 ± 35°C, which seems reasonable, however, given our amphibole compositions, they should be interpreted with some caution.
The original zircon saturation model of Watson and Harrison (Reference Watson and Harrison1983) was formulated for peraluminous and metaluminous compositions; given that Zr may also be present in significant amounts in other mineral phases in peralkaline rocks, the model is not widely applicable. Unpublished data for Ti-in-zircon (Siachoque, Reference Siachoque, Garcia and Vlach2020) point to temperature averages ~902°C and 843°C for the alkaline-1 and alkaline-2 series, respectively, which are in good agreement with previous estimates by Vilalva et al. (Reference Vilalva, Simonetti and Vlach2019) for similar rocks in the province. Moreover, qualitative information concerning solidus temperatures might be obtained from the occurrence of sodic amphiboles and pyroxenes (Ernst, Reference Ernst1962, Reference Ernst1968; Scaillet and Macdonald, Reference Scaillet and Macdonald2001). Accordingly, the occurrence of primary ferro-ferri-winchite, arfvedsonite and riebeckite suggests maximum temperatures for the system solidus between 655 and 750°C.
Temperatures for hydrothermal alteration and formation of the greisens in the aluminous association were estimated by chlorite thermometry, using IVAl variation with Fe/(Fe+Mg) ratios as a function of temperatures. We applied the calibrations of Zang and Fyfe (Reference Zang and Fyfe1995) and El-Sharkawy (Reference El-Sharkawy2000) and the results are similar for both the main inequigranular granite and the hosted greisens: 266 to 279°C and 263 to 283°C, based on the calibration of Zang and Fyfe (Reference Zang and Fyfe1995), and 244 to 256°C and 239 to 255°C according to El-Sharkawy (Reference El-Sharkawy2000), respectively (see Supplementary table S5). These results agree with previous equilibration temperatures between 165 and 350°C for fluid inclusion in quartz, obtained by Oliveira (Reference Oliveira1989) for a similar greisen.
Redox conditions
Qualitative information on the oxygen fugacity ($f_{{\rm O}_ 2}$) conditions during crystallisation of the Mandira magmas of the aluminous association may be obtained from the relative contents of Fe and Mg in calcic amphibole and biotite, on the basis of experimental and empirical data (Wones, Reference Wones1981; Anderson and Smith, Reference Anderson and Smith1995; Anderson et al., Reference Anderson, Barth, Wooden, Mazdab, Putirka and Tepley2008). Both amphiboles and biotite in this association have high Fe# values, up to 0.94, implying crystallisation at relatively low $f_{{\rm O}_ 2}$
conditions (Fig. 13a,b) buffered at ~ –1 ≤ QFM ≤ 0, close to the limit between granite rocks from the ilmenite and magnetite series (Ishihara, Reference Ishihara1977). These estimates indicate that Mandira aluminous granites were formed under more reduced conditions when compared with other plutons from the aluminous association within the Graciosa Province (in the 0 ≤ QFM ≤ +3 range, cf. Gualda and Vlach, Reference Gualda and Vlach2007a; Vilalva and Vlach, Reference Vilalva and Vlach2014).

Fig. 13. Fe/(Fe + Mg) vs. AlT diagrams for primary calcic-amphiboles (a) and biotite (b) in granites from the aluminous association. Qualitative redox conditions estimates and correlation with the magnetite and ilmenite series from Ishihara (Reference Ishihara1977) according to Anderson and Smith (Reference Anderson and Smith1995) and Anderson et al. (Reference Anderson, Barth, Wooden, Mazdab, Putirka and Tepley2008), respectively.
Furthermore, Fe3+/FeT ratios in sodic and sodic–calcic amphiboles (e.g. Clowe et al., Reference Clowe, Popp and Fritz1988; Papoutsa and Pe-piper, Reference Papoutsa and Pe-piper2014; Vilalva et al., Reference Vilalva, Vlach and Simonetti2016) furnish some qualitative information concerning redox conditions for the alkaline series. Accordingly, 0.05 ≤ Fe3+/FeT ≤ 0.25 values point to moderate oxidising conditions, close to or buffered by the Titanite–Magnetite–Quartz–Amphibole–Ilmenite (TMQAI) buffer (Wones, Reference Wones1989), as supported by the observed mineral assemblage in the alkaline-1 series. In the case of the alkaline-2 series, sodic amphiboles with significant Li contents and 0.05 ≤ Fe3+/FeT ≤ 0.41 suggests oxidising conditions above QFM (e.g. Marks et al., Reference Marks, Vennemann, Siebel and Markl2003; Pe-Piper, Reference Pe-Piper2007; Vilalva et al., Reference Vilalva, Vlach and Simonetti2016), somewhat higher than those inferred for the alkaline-1 series.
Volatile (H2O, F and Cl) fugacity relations
Halogen contents of hydrous minerals, such as biotite, are sensitive indicators of the physicochemical conditions of late-stage or hydrothermal fluids (Ayati et al., Reference Ayati, Yavuz, Noghreyan, Haroni and Yavuz2008; Siahcheshm et al., Reference Siahcheshm, Calagari, Abedini and Lentz2012; Idrus, Reference Idrus2018; Moshefi et al., Reference Moshefi, Hosseinzadeh, Moayyed and Lentz2018). Thus, we computed the fugacity ratios f HF/f HCl and $f_{{\rm H}_ 2{\rm O}}$/f HF following Munoz (Reference Munoz and Reeder1984, Reference Munoz1992), on the basis of the revised coefficients for F–Cl–OH exchange between biotite and hydrothermal fluids (Zhu and Sverjensky, Reference Zhu and Sverjensky1991, Reference Zhu and Sverjensky1992). The calculated molecular fractions for biotite components and fugacity ratios are presented in Table 3 and Supplementary table S4.
The correlation between mole fractions of Mg (XMg) and F (XF), to examine the Fe–F avoidance (e.g. Munoz, Reference Munoz and Reeder1984; Mason, Reference Mason1992) is shown in Fig. 14a). Primary annite in the aluminous association has the highest XMg (up to 0.22) and XF (up to 0.03) values, whereas the late- to hydrothermal annite–siderophyllite presents intermediate values (up to 0.03 and to 0.13, respectively), and annite in the alkaline granites has lower values (up to 0.03 and 0.06, respectively). All micas plot in the ‘Clusters’ field in this diagram (Fig. 14a), which is generally ascribed to Fe- or F-rich biotite and indicates possible grouping of atoms (e.g. Fe–F, OH–OH) to minimise violations of the Fe–F avoidance principle (Mason, Reference Mason1992).

Fig. 14. Main volatile relations deduced from biotite compositions of the Mandira granites: (a) XF vs. XMg (molar fractions) diagram depicting the Fe–F avoidance allowed and violated fields, following Mason (Reference Mason1992): (b) Variation of the fugacity ratios expressed as log(f HF/f HCl) vs. log$( f_{{\rm H}_ 2{\rm O}}$/f HF); (c) log (XF/XCl) vs. XMg diagram. Equal ratio lines calculated following Munoz (Reference Munoz1992), assuming biotite–fluid equilibrium at 750°C for primary annite and at 350°C for secondary annite–siderophyllite (see also Salazar-Naranjo and Vlach, Reference Salazar-Naranjo and Vlach2018). Symbols as in Fig. 9.
Log(f HF/f HCl) and log$( f_{{\rm H}_ 2{\rm O}}$/f HF) values were computed considering temperatures of 750°C for the primary annite in the aluminous granites and 350°C for the late-crystallised to hydrothermal annite and annite–syderophyllite in both the alkaline-1 and alkaline-2 granites. The results vary between –4.3 ≤ log(f HF/f HCl) ≤ –0.9 and 4.3 ≤ log$( f_{{\rm H}_ 2{\rm O}}$
/f HF) ≤ 7.2 (Fig. 14b). Annite crystals from the aluminous association record higher log(f HF/f HCl) ratios (from –2.63 to –0.89) compared to the alkaline–1 (from – 2.51 to – 2.11) and alkaline–2 (from –4.23 to –2.97) series. Moreover, the transition from magmatic to hydrothermal crystallisation stage shows increasing log($f_{{\rm H}_ 2{\rm O}}$
/f HF) and decreasing log(f HF/f HCl) values. This tendency, in part related to the strong dependence of these parameters on the crystallisation temperature, agrees with the general F and H2O preference for the hydrothermal crystallisation stages.
Some additional inferences on the biotite–fluid equilibrium may be made considering the XMg vs. log(f XF/f XCL) diagram coupled with computed log(f HF/f HCl) values, as represented by dashed lines in Fig. 14c (e.g. Salazar-Naranjo and Vlach, Reference Salazar-Naranjo and Vlach2018). Annite crystals in the alkaline-1 series present relatively constant log(f HF/f HCl) = –2 values, whereas annite and siderophyllite types in the aluminous and the alkaline-2 series present values with a large dispersion, between –2.5 and –1.1 and –3.8 and –2.3, respectively. This suggests that biotite–fluid equilibration occurred under relatively more homogeneous fluid compositions in the former case.
Amphibole compositional trends and comparison with other occurrences in the Graciosa Province
Amphibole compositional variations are plotted in the Ca2+ + IVAl vs. Si + Na + K diagram of Giret et al. (Reference Giret, Bonin and Leger1980) to examine the main compositional trends and compare them with similar occurrences within the Graciosa Province in Fig. 15. Three main compositional trends can be seen and, on the whole, they reinforce the compositional contrasts in the Mandira granites and have several similarities with other occurrences in the province (Gualda and Vlach, Reference Gualda and Vlach2007c; Vilalva and Vlach, Reference Vilalva and Vlach2014; Vilalva et al., Reference Vilalva, Vlach and Simonetti2016): (1) a short trend corresponding to calcic amphiboles in the aluminous association; (2) a compositionally expanded continuous trend in the alkaline-1 series, which reinforces the established connection between calcic and sodic–calcic amphiboles in this series; and (3) a relatively less expanded trend for arfvedsonite and riebeckite in the alkaline-2 series. The relatively expanded compositional variations registered in calcic and sodic–calcic amphiboles of the alkaline-1 series suggest that the original magmas were subjected to more extensive fractionation processes, with increasing alkalinity in the crystallising environment.

Fig. 15. Si + Na + K vs. Ca+IVAl plot (apfu) for amphibole-group minerals from the Mandira granites. Typical evolutionary trajectories determined for other plutons from the aluminous (Farinha Seca/Orgãos; Orgãos/Capivari; Quiriri) and alkaline (Anhangava/Farinha Seca/Orgãos; Papanduva; Corupá) petrographic associations in the Graciosa Province (Garin, Reference Garin2002; Gualda and Vlach, Reference Gualda and Vlach2007c; Vilalva et al., Reference Vilalva, Vlach and Simonetti2016) are also represented for comparison. Symbols as in Fig. 6.
A well-defined compositional gap between the primary sodic–calcic amphiboles and the hydrothermal riebeckite in the alkaline-1 series is observed. This might suggest a late destabilisation of ferro-ferri-winchite and consequent precipitation of riebeckite in a contrasted, Na-rich, higher oxidising environment as suggested by Giret et al. (Reference Giret, Bonin and Leger1980). Gualda and Vlach (Reference Gualda and Vlach2007c) reported similar compositional gaps within alkaline granites from the Farinha Seca and Orgãos plutons in the Graciosa province (Fig. 15); however, in this case, a progressive increase in Na-Fe3+ from the cores of the crystals to the rims was more evident. The compositions of sodic amphiboles in the alkaline-2 series vary from intermediate arfvedsonite/riebeckite to an almost pure riebeckite end-member; a feature clearly related to an evolution under increasing alkalinity and oxidising conditions (e.g. Strong and Taylor, Reference Strong and Taylor1984, Fig. 8a,b); these compositional trends are similar to those observed in the Papanduva and Corupá peralkaline granites in the province (Fig. 15).
REE amphibole/melt partition coefficients and controls
Rare earth and other trace element incorporation in the mineral structure of the amphibole-group minerals may be complex (Bottazzi et al., Reference Bottazzi, Tiepolo, Vannucci, Zanetti, Brumm, Foley and Oberti1999; Hilyard et al., Reference Hilyard, Nielsen, Beard, Patinõ–Douce and Blencoe2000; Tiepolo et al., Reference Tiepolo, Vannucci, Bottazzi, Oberti, Zanetti and Foley2000, Reference Tiepolo, Oberti, Zanetti, Foley, Vannucci, Foley, Hawthorne, Oberti, Della Ventura and Mottana2007; Wood and Blundy, Reference Wood, Blundy, Holland and Turekian2014; Shimizu et al., Reference Shimizu, Liang, Sun, Jackson and Saal2017), and there are relatively few data, particularly for sodic and sodic–calcic varieties in the literature (e.g. Marshall et al., Reference Marshall, MacDonald, Rogers, Fitton, Tindle, Nejbert and Hinton2009; Vilalva et al., Reference Vilalva, Vlach and Simonetti2016; Vasyukova and Williams-Jones, Reference Vasyukova and Williams-Jones2019). Thus, on the basis of our whole-rock and mineral data, we use our data and the Lattice Strain Model (LSM, Blundy and Wood, Reference Blundy and Wood1994) to examine REE behaviour and evaluate partition coefficients (amph/LD REE) for the ferro-ferri-hornblende in the aluminous association and the ferro-ferri-whinchite and riebeckite from alkaline series 1 and 2, assuming that our whole-rock compositions are reliable estimates of the original magma compositions. As these minerals are not liquidus phases in the host granites, the liquidus compositions at the onset of their crystallisation needs to be estimated by extracting the appropriate REE quantities incorporated in the previous crystallised phases, a particularly critical situation in the case of the sodic varieties in granites subjected to an agpaitic crystallisation sequence.
To model REE (including Y, Sc) contents of the liquidus at the beginning of amphibole crystallisation, we started from our whole-rock geochemical data, the modal compositions converted into weighted fractions, the interpreted mineral crystallisation sequence and partition coefficients from the literature (see Supplementary table S7 for parameter settings and references). We applied both the Rayleigh fractional and the equilibrium crystallisation models. Because results from both were similar and the rocks studied were emplaced at relatively shallow crustal levels, the results for the first model is given by:

where C 0(i) is the concentration of the element i in the initial liquid, Di is the bulk partition coefficient for the element i, F is the fraction of liquid remaining (= 100 – crystallised fraction) during crystallisation, and C L(i) is the concentration of i in this liquid. We estimated that amphibole began crystallising when the remaining liquid values were 80, 70 and 50 for the aluminous, alkaline-1 and alkaline-2 granite samples, respectively. Modelled parameters and the obtained REE liquid compositions at the beginning of amphibole crystallisation in each case are also given in Supplementary table S7.
Calculated partition coefficients for REE (amph/LD REE = average REE concentrations measured in amphibole/REE concentrations in the modelled liquid) for our calcic, sodic–calcic, and sodic amphiboles are given in Table 8 and depicted in Fig. 16a. As expected from previous studies (Marks et al., Reference Marks, Halama, Wenzel and Markl2004; Vilalva et al., Reference Vilalva, Vlach and Simonetti2016), the partition coefficients are highest for ferro-ferri-hornblende and decrease through ferro-ferri-winchite to riebeckite (in both the M2 and M4 sites for the latter two minerals) with increase in compatibility from LREE to HREE especially for the alkali amphiboles and decreasing negative Eu anomaly. The Amp/LD REE values for both LREE and HREE in ferro-ferri-hornblende and ferro-ferri-winchite are correlated positively with the high Al and Ca contents from these varieties and their corresponding rocks, supporting a conclusion that Al and Ca enhances REE incorporation into these amphiboles (Bottazzi et al., Reference Bottazzi, Tiepolo, Vannucci, Zanetti, Brumm, Foley and Oberti1999; Vilalva et al., Reference Vilalva, Vlach and Simonetti2016), whereas low Amp/LD REE in riebeckite indicate a limited REE incorporation due to its low Al and Ca and high Na and Fe3+ contents (e.g. Siegel et al., Reference Siegel, Williams-Jones and van Hinsberg2017). The REE+3 incorporation in the samples studied may be described by the coupled substitution reaction:

as proposed by Vilalva et al. (Reference Vilalva, Vlach and Simonetti2016) for other alkaline granites from the province.
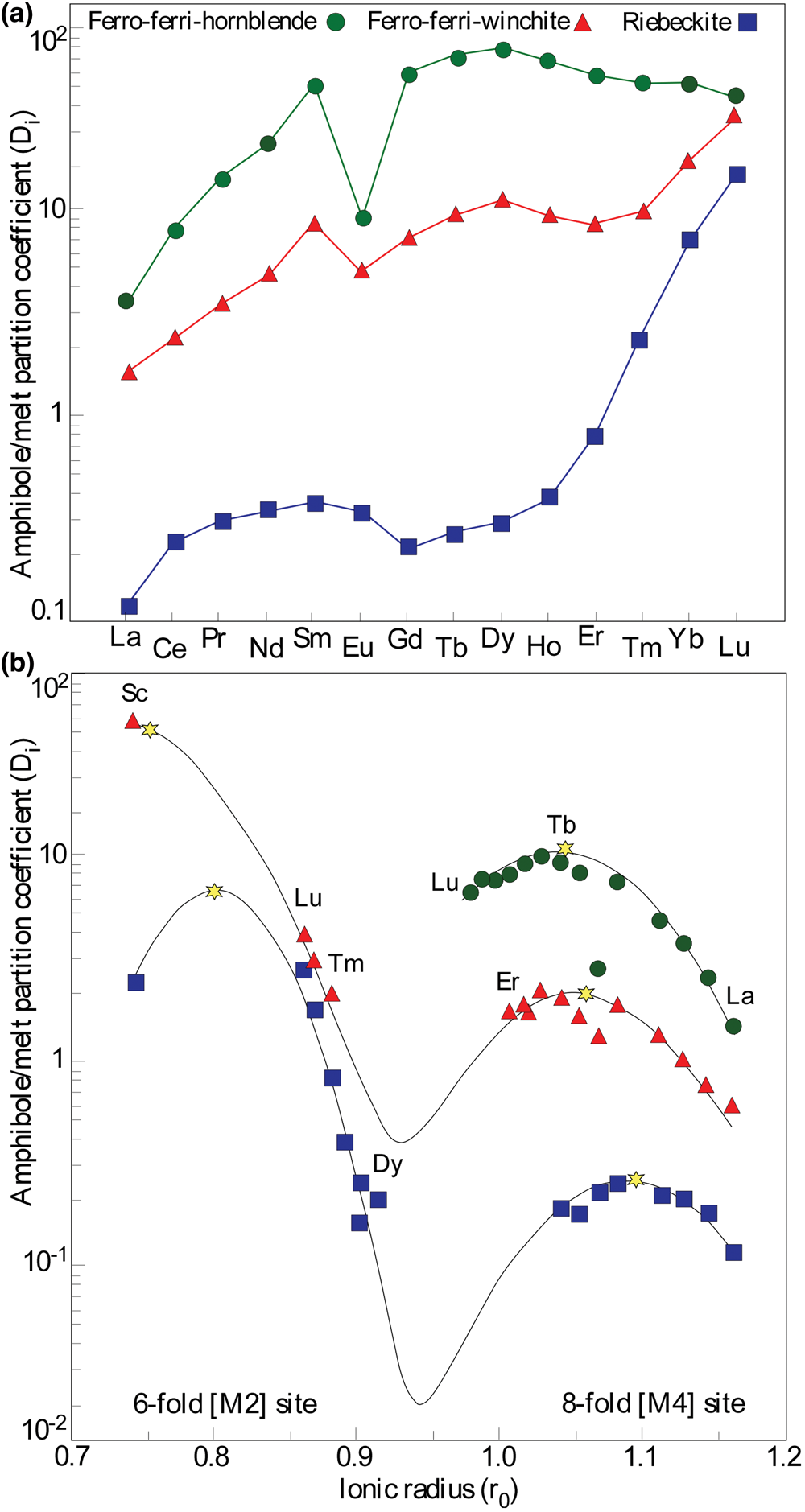
Fig. 16. (a) Plot of the calculated amph/LD REE in calcic, sodic–calcic, and sodic amphiboles from the Mandira Massif. (b) Onuma-type parabolic curves modelled by the Strain Lattice Model. Yellow asterisks represent the modelled ideal ionic radius for M2 and M4 sites. See discussion in the text. Symbol colours as in Fig. 6.
Table 8. Partition coefficients (Amp/LD REE) for REE (+Y, Sc) and LSM site parameters in amphiboles from the Mandira Granite Massif.

Notes: Computed (Amp/LD REE) and LSM adjusted (Amp/LD*REE) values. D 0 = partition coefficient for a cation with radius r 0 in the site M; E is the Young modulus of the site.
By applying the calculated REE coefficient partitions and respective cation radius, LSM parabolic curves were single- or double-fitted by using software of Dalou et al. (Reference Dalou, Boulon, T. Koga, Dalou and Dennen2018), assuming crystallisation temperatures of ~ 800, 750 and 700°C for ferro-ferri-hornblende, ferro-ferri-winchite and riebeckite, respectively. The adjusted (Amp/LD*REE) values, together with the respective LSM fitted parameters, are presented in the Table 8 for comparison with the calculated values and represented in Fig. 16b. Except for Eu in ferro-ferri-hornblende, Amp/LD REE and Amp/LD*REE values are in good agreement.
Previous alkali-amphibole–melt partition studies demonstrated that REE are incorporated mainly in two crystallographic sites; HREE (Dy–Lu) and Sc occupy preferentially the 6-fold [M2] site, whereas LREE–MREE (La–Tb) prefer the larger 8-fold [M4] sites (Bottazzi et al., Reference Bottazzi, Tiepolo, Vannucci, Zanetti, Brumm, Foley and Oberti1999; Reguir et al., Reference Reguir, Chakhmouradian, Pisiak, Halden, Yang, Xu, Kynický and Couëslan2012; Vilalva et al., Reference Vilalva, Vlach and Simonetti2016; Vasyukova and Williams-Jones, Reference Vasyukova and Williams-Jones2019). In contrast, in calcic amphiboles all REE might be accommodated in the M4 sites (Bottazzi et al., Reference Bottazzi, Tiepolo, Vannucci, Zanetti, Brumm, Foley and Oberti1999; Hilyard et al., Reference Hilyard, Nielsen, Beard, Patinõ–Douce and Blencoe2000).
In the case of hornblende, the single-fitted r 0 = 1.041±0.002 Å value for the M4 site is significantly lower than the ideal Ca radius (1.12 Å), a result close to that obtained by Bottazzi et al. (Reference Bottazzi, Tiepolo, Vannucci, Zanetti, Brumm, Foley and Oberti1999) for their kaersutite sample (r 0 = 1.03 Å). These authors attributed this departure from the ideal value to the occurrence of two distinct M4 sites, a relatively larger M4 occupied mainly by Ca and a smaller M4’, occupied by cations such as Fe2+ and Mn2+, resulting from a ‘cummingtonite’ component in the amphibole composition. As a rule, the REE should prefer such smaller sites. This appears not to be our case, as our structural formulae suggest only Ca and Na occupying the M4 sites; as a matter of precaution however, it is worth noting that our compositions have significant Ti contents (higher than 0.15 apfu, see Bottazzi et al. Reference Bottazzi, Tiepolo, Vannucci, Zanetti, Brumm, Foley and Oberti1999). Nevertheless, the most significant diffferent features of our hornblende, as compared with the kaersutite of Bottazzi et al. (Reference Bottazzi, Tiepolo, Vannucci, Zanetti, Brumm, Foley and Oberti1999), in addition to its lower TiO2, are the lower Al2O3 and higher FeOT contents and Fe2+/Fe3+ ratios. Such compositional differences should lead arguably to the occurrence of somewhat smaller M4’ sites, also present in our case, which should be preferred by the REE.
In contrast, the LREE and MREE enter the riebeckite M4 site, with a double-fit modelled r 0 = 1.09±0.02 Å, relatively close to the Ca radius, whereas the HREE fill octahedral M2 sites with r 0 = 0.80±0.03 Å, a value approaching the Fe2+ octahedral radius (0.78 Å), as estimated for sodic amphiboles (e.g. Vilalva et al., Reference Vilalva, Vlach and Simonetti2016; Vasyukova and Williams-Jones, Reference Vasyukova and Williams-Jones2019). It must be noted that our riebeckite contains up to 0.3 apfu Li and is Ca-poor (≤ 0.19 apfu, see Table 2). Winchite, however, is relatively Ca-rich (with up to 1.49 apfu in M4), approaching the composition of Ca-amphiboles, and contains some Li in the M2 and, eventually, M4 sites (see Supplementary table S3). The REE site allocation seems to be more unusual in this mineral, as REE from La to Er appear to best conform to the M4 sites, whereas Tm to Lu (and Sc) fit better to the M2 sites (Fig. 16b). Thus, La–Er and Tm–Lu distributions are like those described for hornblende and riebeckite, respectively, with model r 0 values of 1.06±0.02 for the M4 and 0.75±0.03 for the M2 sites.
The above discussion reinforces conclusions that REE distributions and site occupancies within amphibole-group minerals seem to be more complex and, in general, support the hypothesis that the M4 (M4 and M4’) and M2 sites should contain different proportions of REE in these minerals, depending on their compositions (e.g. Bottazzi et al., Reference Bottazzi, Tiepolo, Vannucci, Zanetti, Brumm, Foley and Oberti1999).
Conclusions
The Mandira Granite Massif in the Graciosa Province of A-type granites and syenites, SE Brazil, consists of discrete intrusions of alkali-feldspar hypersolvus granites represented by two petrographically distinct series (1 and 2) of alkaline granites, and coeval aluminous syenogranites. Textural and compositional properties of magmatic and post-magmatic amphibole-group minerals and phyllosilicates in the granites provide information on the crystallisation conditions and mineralogical evolution within the massif, as summarised below.
Amphibole compositions vary widely from calcic, sodic–calcic to sodic. Primary calcic amphiboles correspond to ferro-edenite and ferro-ferri-hornblende (alkali-1 series) and ferro-ferri-hornblende and hastingsite (aluminous association). Ferro-ferri-winchite is the main primary sodic–calcic phase in the alkaline-1 series, whereas both arfvedsonite and riebeckite are primary phases in the alkaline-2 series. Post-magmatic riebeckite is typical of both alkaline series, whereas post-magmatic ferro-actinolite occurs only in the alkaline-1 granites. Trace-element compositions of primary calcic, sodic–calcic, and sodic amphiboles indicate relatively high abundances of large-ion lithophile element (LILE) and HFSE. Concentrations of REE are high in calcic and moderate to low in the sodic–calcic and sodic phases, respectively. The estimated REE partition coefficients indicate an increase in compatibility from LREE to HREE and well-marked preference of HREE over LREE in the sodic–calcic and, particularly, the sodic amphiboles.
Biotite (annite) crystallised as a magmatic phase directly from the magmas or from a late-magmatic reaction, replacing previous calcic amphiboles in the aluminous association. In the alkaline series biotite is mainly a late- to post-magmatic phase. It corresponds to iron-rich varieties varying from late- to post-magmatic annite (alkaline-1 and -2 series) to post-magmatic annite–siderophyllite (alkaline-2 series). Chlorite (chamosite) is a typical hydrothermal phase in the aluminous granites and related quartz–mica greisens, being relatively Fe- and Mn-rich and Mg-poor in the latter Ferri-stilpnomelane occurs in both aluminous and alkaline-1 granites, being relatively Fe- and Zn-rich and Mg- and K-poor in the latter.
Estimated crystallisation conditions indicate magma emplacement at relatively shallow crustal levels, between 90 and 230 MPa, with close-to-liquidus temperatures ~900°C (alkaline-1), 845°C (alkaline-2) and 830°C (aluminous). In the amphibole-bearing granites of the aluminous association, plagioclase–hornblende temperatures give, on average, 766°C. Chlorite geothermometry indicates temperatures of ~240–280°C for the hydrothermal alteration of granites from the aluminous association and the development of the related greisens. Redox crystallisation conditions were estimated as relatively low $f_{{\rm O}_ 2}$, buffered ~ –1 ≤ QFM ≤ 0 for the aluminous granites. The data indicate that these granites are the most reduced varieties in the aluminous associations from the Graciosa Province known at present. Ratios of Fe3+/FeT for primary sodic–calcic and sodic amphiboles in the alkaline series suggest crystallisation above the QFM buffer, under low/moderate $f_{{\rm O}_ 2}$
conditions for the alkaline-1 series and higher oxidising conditions for the alkaline-2 series, in agreement with previous estimates for similar plutons in the province.
Halogen fugacity ratios in biotite suggest crystallisation conditions under ~ –2 ≤ log(f HF/f HCl) ≤ –1 (magmatic biotite in the aluminous association), log(f HF/f HCl) = –2 (alkaline-1 series), and –3.5 ≤ log(f HF/f HCl) ≤ –2.5 (alkaline-2 series), reinforcing the preference of F over Cl for the late- to post-magmatic stages of crystallisation.
Supplementary material
To view supplementary material for this article, please visit https://doi.org/10.1180/mgm.2021.65
Acknowledgements
This research was supported by the Fundação de Amparo à Pesquisa do Estado de São Paulo (FAPESP, grants 2008/00562-0 and 2019/17343-4). AS benefits from a PhD scholarship from Coordenação de Pessoal de Nível Superior (CAPES, Finance Code 001), and CS from an undergraduate scholarship (FAPESP Proc. 2009/04321-0). We thank Dra. MC Oliveira for giving access to rock samples of the Mandira granites, the staff of the GeoAnalitica Core Facility for guidance and support during laboratory work, and Dr G Szabó for English revision. Dr R Halama and an anonymous reviewer are acknowledged for revisions, and Dr Ian Coulson, Dr Roger Mitchell and Dr Helen Kerbey for editorial handling.