Introduction
The intricacy and diversity of macrolichen morphology have profound ecological implications. Novel ways of imaging and quantifying features in three dimensions, from nano- to landscape-scales, can now capture morphological complexity, yielding new insights into larger taxonomic and environmental questions. Valladares et al. (Reference Valladares, Sancho and Ascaso1998), for example, employed stereology to study water storage traits in the lichen family Umbilicariaceae. More advanced methods such as scanning ion conductance microscopy can be used to map cell surfaces in 3D with high precision (Novak et al. Reference Novak, Li, Shevchuk, Stepanyan, Caldwell, Hughes, Smart, Gorelik, Ostanin and Moss2009). Microhabitat-scale lichen distribution has been recorded in the field using electromagnetic position-tracking (Broom et al. Reference Broom, Parkinson, Green and Seppelt1999). Fractal geometry, an index for shape complexity, has been shown to predict the distribution of lichen growth forms along fog gradients (Stanton & Horn Reference Stanton and Horn2013). Similarly, an increase in branchiness of boreal forest hair lichens at higher canopy heights allows the trapping of more external water (Esseen et al. Reference Esseen, Rönnqvist, Gauslaa and Coxson2017). Computer-generated three-dimensional models also offer unique tools for teaching plant development and architecture (Boudon et al. Reference Boudon, Pradal, Cokelaer, Prusinkiewicz and Godin2012), in addition to providing researchers with valuable taxonomic information (see Reeb et al. Reference Reeb, Kaandorp, Jansson, Puillandre, Dubuisson, Cornette, Jabbour, Coudert, Patiño and Flot2018). These models are still reduced versions of intricate 3D shapes and other methods such as CT scanning, 3D scanning or photogrammetry are needed to capture even greater complexity to explore the form-function relationship.
Photogrammetry, a process by which photographs are aligned and rendered to generate 3D models, has a variety of commercial and scientific applications. 3D digitization of insects, for example, allows for rapid sharing of detailed species information without the need for handling delicate specimens in scientific collections (Nguyen et al. Reference Nguyen, Lovell, Adcock and La Salle2014). On a larger scale, 3D models of entire coral reefs have opened up new opportunities for analyzing complex environmental landscapes (Young et al. Reference Young, Dey, Rogers and Exton2017). Photogrammetry techniques have also been used to distinguish and quantify mat-forming reindeer lichens (Cladonia spp.) in forest habitats (Korpela Reference Korpela2008) and make robust estimations of species-specific lichen cover in the Namib Desert (Hinchcliffe et al. Reference Hinchcliffe, Bollard-Breen, Cowan, Doshi, Gillman, Maggs-Kolling, de los Ríos-Murillo and Pointing2017), which provide detailed data regarding site productivity and long-term vegetation changes while avoiding time-consuming field-based measurements.
A lichen thallus is a miniature landscape. This microtopography can have ecological roles that parallel those at the landscape scale, such as shaping animal habitats (see Shorrocks et al. Reference Shorrocks, Marsters, Ward and Evennett1991) and influencing physical processes such as hydration. Quantifying morphological lichen traits commonly requires accurate thallus surface area measurements, determined using projected (i.e. planimetric) photographic images and software such as ImageJ. Planimetric area (hereafter referred to as A2D) provides a useful and rapid proxy for “true” 3D surface area (A3D), yet the correlation between A2D and A3D has rarely been examined in lichens.
Macrolichen surface complexity varies across species, from highly dissected branched fruticose growth forms to flattened or foliose morphologies. Nevertheless, even foliose lichens can exhibit structures such as apothecia or asexual propagules that are by no means two-dimensional. The lobes of the foliose Lobaria pulmonaria (L.) Hoffm. form a foveolate thallus topography with a conspicuous reticulum of ridges and intervening depressions (Fig. 1). The ridges offer mechanical support and may serve as transport lines, helping to move water and nutrients both laterally towards the lobe margins and distally towards the growing thallus tips during wetting-drying cycles. The depressions (foveoles) capture litter and probably accumulate nutrients for eventual transport to other parts of the thallus. These foveoles also visibly serve as water reservoirs that extend physiological activity through prolonged retention of rainwater (Fig. 1).
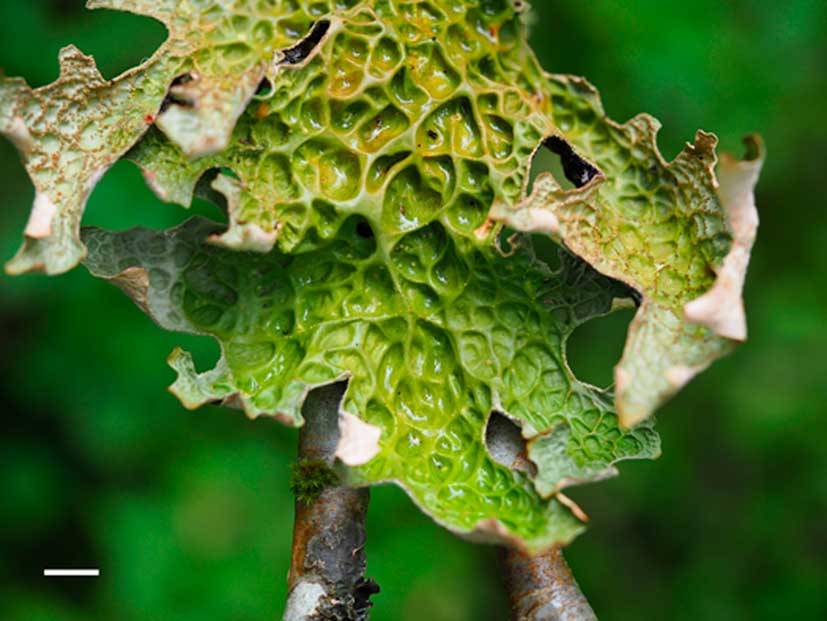
Fig. 1 Lobaria pulmonaria from a boreal rainforest in Trøndelag, western Norway, exhibiting reticulate thallus topography. Depressions in the reticulum allow water to pool, extending hydration after rain and/or dewfall. Thinner portions of the thallus with shallower depressions dry out first. Scale=1 cm.
Using photogrammetry, this study aims to quantify these morphological features in order to assess the variability in external water pools across L. pulmonaria thalli. The lichen’s deviation from the A2D can be assessed via the A3D:A2D ratio (i.e. photogrammetry-generated, “true” area : projected area). This ratio, used to generate a measure of surface roughness in larger landscape applications (see Jenness Reference Jenness2004), also has the potential to be applied to much smaller scales, including that of a foliose lichen such as L. pulmonaria. This study aims to test if the application of this technique can yield a rapid and robust estimation of thallus three-dimensionality. To achieve this, digital 3D models of L. pulmonaria thallus topography were built using photogrammetry, and the 3D dorsal surface areas were quantified. Since the 3D:2D area ratio likely influences water interception and retention, the relationship between three-dimensionality and external water-holding capacity is explored.
Materials and Methods
Twenty-five healthy thalli of Lobaria pulmonaria, ranging in size from 2·6–152 cm2 (wet A2D), were randomly selected from three Populus tremula trees growing in a mixed boreal rainforest near Namsos, Trøndelag, western Norway (64°21′N, 11°20′E). A custom-built LED light box was used to provide consistent photography lighting. A manual turntable (20 cm diam.) served as the photography platform, upon which a millimetre scale and non-overlapping thalli were placed (i.e. as many thalli as could fit on the turntable at one time). Photographs were taken with an Olympus OM-D E-M5 Mark II 16MP camera fitted with an Olympus M.Zuiko Digital ED 60mm f/2.8 macro lens. Fifty-four images were used to build 3D photogrammetric models of the upper (dorsal) thallus surfaces; each image was a composite of 23 automatically focus-bracketed photographs to ensure that all parts of each final image were in focus. This was crucial for accurate photograph alignment and model building in the following steps.
The stacked images were imported into Agisoft PhotoScan Professional 1.4.0 (Agisoft LLC, St. Petersburg, Russia) and aligned. Dense point clouds were then generated, from which polygonal meshes were built. Markers within Agisoft were calibrated with the turntable millimetre scale and were used to create digital scale bars. Individual thallus surface areas (A3D) were then measured. For A2D measurements, the thalli were photographed from above and measured using ImageJ 1.50i (Bethesda, MD, USA). Images were captured for each thallus in dry and fully hydrated states for both A3D and A2D measurements. The ratio A3D:A2D was calculated to provide a measure of three-dimensionality (Fig. 2).
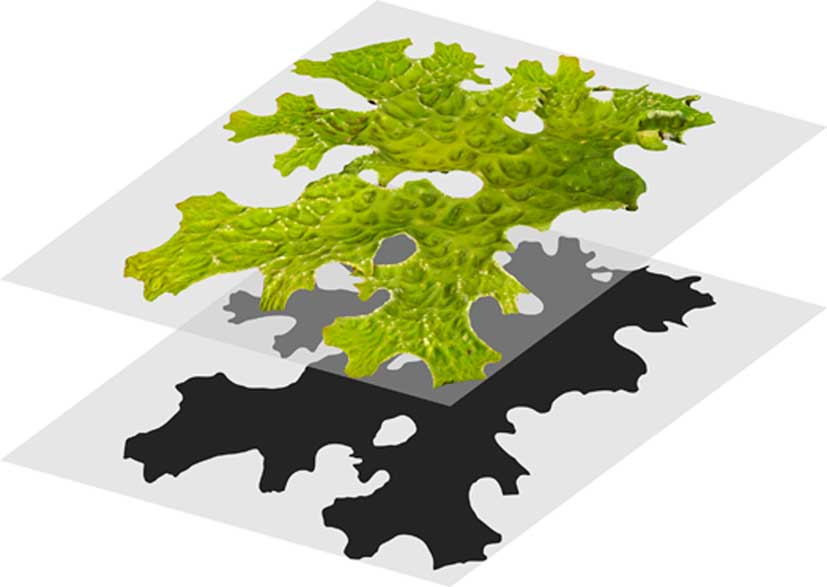
Fig. 2 Calculation of the A3D:A2D ratio of the thallus of Lobaria pulmonaria was undertaken by dividing the thallus surface area from photogrammetric models (top thallus, A3D) by the surface area from projected photographs (bottom thallus, A2D). This ratio is always >1. A2D=planimetric area; A3D=3D surface area.
Dry mass (DM) was determined by adjusting the mass of air-dried lichens with a correction factor to account for residual ambient intrathalline water. Specific thallus mass (STM) denotes the mass per unit thallus area (STM=DM/A2D). Saturated thalli were sprayed with water and immediately weighed to measure total wet mass (WMtotal) and then gently blotted with filter paper to remove external water films (WMinternal). The resulting measurements were used to calculate water-holding capacity (WHC=(WM−DM)/A2D) both after spraying (WHCtotal) and after blotting (WHCinternal). External water-holding capacity (WHCexternal) was computed as WHCtotal−WHCinternal.
Results
Projected (2D) images of both dry and wet Lobaria pulmonaria thalli underestimated the A3D by 19·3 ± 0·9% (dry area mean ± 1SE) and 16·9 ± 1·2% (wet area mean ± 1SE). There was a strong linear relationship between A2D and A3D (r 2 adj=0·998). Following hydration, L. pulmonaria increased in A2D by 39·2 ± 1·2% and A3D by 36·2 ± 0·6%.
The A3D:A2D ratio was a strong predictor of both WHCexternal and STM (Fig. 3A & B). Both dependent variables increased positively with the A3D:A2D ratio. STM was a slightly weaker predictor for WHCexternal (r 2 adj=0·541; regression not shown) than was the A3D:A2D ratio (r 2 adj=0·591, Fig. 3A). There was a high correlation between the A3D:A2D ratio and STM (r=0·770, P<0·001).

Fig. 3 The relationship between the A3D:A2D ratio in Lobaria pulmonaria and external water-holding capacity (A) and specific thallus mass (B). Individual specimen values (open circles), linear regressions (solid lines) and 95% confidence intervals (dotted lines) are shown. A2D=planimetric area; A3D=3D surface area.
Discussion
Thallus size strongly influences water retention (Merinero et al. Reference Merinero, Hilmo and Gauslaa2014), governing the duration of photosynthetically active periods after an hydration event (Gauslaa & Solhaug Reference Gauslaa and Solhaug1998; Gauslaa et al. Reference Gauslaa, Solhaug and Longinotti2017). While STM drives internal water-holding capacity (Gauslaa Reference Gauslaa2014; Esseen et al. Reference Esseen, Olsson, Coxson and Gauslaa2015) and water vapour uptake from humid air (Phinney et al. Reference Phinney, Solhaug and Gauslaa2018) in lichens, external thallus water is also important for lichen water economy (Esseen et al. Reference Esseen, Rönnqvist, Gauslaa and Coxson2017). The data here show that increasing surface water in Lobaria pulmonaria is achieved not only by a larger thallus area, but also by having a reticulum, in which easily visible water pools (Fig. 1) can accumulate. The reticulum in L. pulmonaria is more pronounced in larger specimens that can consequently hold more external water per thallus area than smaller ones. This may pose a challenge for the establishment of new colonies, in which young thalli are prone to rapid desiccation and often have a short photosynthetically active period after rain or dewfall. However, a small size could imply more rapid and/or more frequent activation by humid air, which may partly explain why Larsson & Gauslaa (Reference Larsson and Gauslaa2011) found that relative growth rates of juvenile L. pulmonaria thalli decrease with increasing size. For larger thalli that require more water to reach saturation, the chance of activation by water vapour or light rain decreases, suggesting a size-dependent increase in demand for structures facilitating water storage enabling prolonged active periods after heavier rainfall.
In addition, the three-dimensional morphology of L. pulmonaria has been shown to mitigate photoinhibition and chlorophyll degradation by producing small shaded regions on the thallus during desiccation-induced curling (Barták et al. Reference Barták, Solhaug, Vrábliková and Gauslaa2006). There is nevertheless an inherent compromise between outward area growth that maximizes light harvesting and three-dimensionality, which is likely to reduce photoinhibition (see Gauslaa et al. Reference Gauslaa, Palmqvist, Solhaug, Hilmo, Holien, Nybakken and Ohlson2009) but increase WHCexternal. In shaded habitats, efficient light harvesting by a flat photosynthetic surface would probably be optimal for lichen growth, whereas in light-exposed sites with higher evaporative demands, a 3D structure maximizing water storage would be an advantage. Although photosynthesis can occur at lower thallus water contents, fungal hyphal expansion requires sufficient turgour pressure, which can drive area growth even in the dark (Bidussi et al. Reference Bidussi, Gauslaa and Solhaug2013). If the thallus depressions remain hydrated longer than the ridges, this could explain the deepening of the fovea over time. Both apical/marginal pseudomeristematic and intercalary growth have been reported in L. pulmonaria (Honegger Reference Honegger1993), which have the combined effect of increasing both projected area and three-dimensionality. Deepened fovea would also benefit internal cyanobacteria (Nostoc)-containing cephalodia, specialized nitrogen-fixing structures that, like cyanolichens, require liquid water for photosynthesis (Lange et al. Reference Lange, Kilian and Ziegler1986). Exceptionally, cephalodia are found on the thallus ridges but more often they occur within the fovea (Cornejo & Scheidegger Reference Cornejo and Scheidegger2013) where they are likely to experience longer hydration cycles.
This study illustrates the utility of photogrammetry in quantifying lichen morphology. In the case of L. pulmonaria, the A3D:A2D ratio predicts WHCexternal values, thereby demonstrating that the reticulum influences the lichen’s water economy. Other epiphytic foliose lichens such as L. scrobiculata and Platismatia norvegica also feature reticulate surfaces, which probably provide comparable ecophysiological functions to those of L. pulmonaria. The methods described here could be extended to other growth forms or adapted to different analyses, such as fractal dimension (see Stanton & Horn Reference Stanton and Horn2013) or fluid dynamics; applying computational fluid dynamics simulations to 3D models of lichens could reveal how air flows around lichen thalli and water vapour is captured. This would be particularly interesting in lichens for which water vapour is a key hydration source.
Although photogrammetry offers novel data to explore trait function, traditional methods undoubtedly remain invaluable. As the correlation between A2D and A3D is high, photogrammetry is unnecessary when quantifying commonly used functional traits (e.g. STM) for classical ecological and ecophysiological investigations. Simple photographs offer rapid and accurate data, while it is labour-intensive to capture an adequate number and quality of photographs for high-resolution photogrammetry. However, with an automated photography set-up and increased computational power, the process could be expedited significantly.
Photogrammetry has some notable advantages over higher-resolution alternatives such as micro CT scanning, including lower cost and the capability of producing natural-colour models (see Nguyen et al. Reference Nguyen, Lovell, Adcock and La Salle2014). Macro 3D scanners also have the potential to be used in the study of complex morphology and have seen vast improvement in recent years. Future work should incorporate these tools to add depth to the ever-expanding study of morphological diversity.
A sincere thank you to Trevor Goward and Yngvar Gauslaa for their insightful comments on the manuscript.