INTRODUCTION
Information derived from lake sediments is useful for inferring palaeoclimatic variations because these deposits provide continuous records of several proxies. Moreover, the possibility of obtaining centennial to decadal resolution highlights lacustrine sediments as powerful palaeoenvironmental recorders (Ortega Guerrero et al., Reference Ortega Guerrero, Thompson and Urrutia Fucugauchi2000; Lane et al., Reference Lane, Horn, Mora and Orvis2009; Danladi and Akçer-Ön, Reference Danladi and Akçer-Ön2018). Magnetic parameters recently became useful proxies for palaeoenvironmental changes (Li et al., Reference Li, Yu, Kodama and Moeller2006; Vázquez et al., Reference Vázquez, Ortega, Davies and Aston2010), but magnetic measurements should be interpreted in terms of climate using models that link magnetic variations of the sediments with climatic changes. This can be achieved by combining magnetic and nonmagnetic parameters (Geiss et al., Reference Geiss, Umbanhowar, Camill and Banerjee2003). In particular, environmental variations in the catchment area of a lake produce changes in particle properties that are transferred into the lake basin. These changes can be observed by three types of responses in magnetic measurements of the sediments obtained: differences in mineralogical content, variations in magnetic grain size, and/or magnetic concentration changes along the cores (Li et al., Reference Li, Yu, Kodama and Moeller2006; Irurzun et al., Reference Irurzun, Orgeira, Gogorza, Sinito, Compagnucci and Zolitschka2014). Peralta et al. (Reference Peralta, Costanzo-Álvarez, Carrillo, Durán, Aldana and Rey2013) argue that a complex relationship between magnetite granulometries and climate transitions could be linked to global temperature changes using ARM/SIRM (anhysteretic remanent magnetisation/saturation of isothermal remanent magnetisation) and its first derivative to infer time limits that bracket the Younger Dryas.
The Southern Hemisphere has much less land mass than the Northern Hemisphere, so fewer records are available. Nevertheless, many studies on lake sediments have been carried out in the last 20 yrs, some of them in South America (e.g., Mancini, Reference Mancini1994; Baker et al., Reference Baker, Seltzer, Fritz, Dunbar, Grove, Tapia, Cross, Rowe and Broda2001; Achaga et al., Reference Achaga, Irurzun, Gogorza, Goguitchaichvili, Morales, Loponte and Sinito2017). The southern extreme of this continent is Patagonia, where Laguna Potrok Aike (230 km south of Laguna Cháltel) has been investigated with geochemical, limnological, sedimentological, biological, seismological, and palaeomagnetic methods (Gogorza et al., Reference Gogorza, Irurzun, Sinito, Lisé-Pronovost, St-Onge, Haberzettl, Ohlendorf, Kastner and Zolitschka2012; Zolitschka et al., Reference Zolitschka, Anselmetti, Ariztegui, Corbella, Francus, Lücke, Maidana, Ohlendorf, Schäbitz and Wastegård2013; Kliem et al., Reference Kliem, Baumgarten, Gebhardt, Hahn, Ohlendorf and Zolitschka2017). Furthermore, Irurzun et al. (Reference Irurzun, Orgeira, Gogorza, Sinito, Compagnucci and Zolitschka2014) developed an experimental model to evaluate lake-level changes using magnetic parameters. The first requirement of this model is a uniform magnetic mineralogy, such that changes in concentration and magnetic grain sizes can be used as proxies. High concentrations of magnetic minerals with a small magnetic grain size were expected for high lake levels and vice versa. This was a useful approach to estimate details of the lake-level curve, but none of the magnetic parameters was identified statistically as the best proxy for lake-level variations of this lake. For Laguna Cháltel, Ohlendorf et al. (Reference Ohlendorf, Fey, Massaferro, Haberzettl, Laprida, Lücke and Maidana2014) developed an age/depth model and analysed several proxies to create a detailed lake-level curve.
For most lake sediments from Patagonia, palaeosecular variations (PSV) and relative palaeointensities (RPI) were the only parameters studied when magnetic analyses were used (Gogorza et al., Reference Gogorza, Sinito, Lirio, Nuñez, Chaparro and Vilas2002, Reference Gogorza, Lirio, Nuñez, Chaparro, Bertorello and Sinito2004, Reference Gogorza, Irurzun, Chaparro, Lirio, Nunez, Bercoff and Sinito2006; Irurzun et al., Reference Irurzun, Gogorza, Sinito, Chaparro, Nuñez and Lirio2008, Reference Irurzun, Gogorza, Torcida, Lirio, Nuñez, Bercoff, Chaparro and Sinito2009; Lisé-Pronovost et al., Reference Lisé-Pronovost, St-Onge, Gogorza, Haberzettl, Preda, Kliem, Francus and Zolitschka2013; Palermo et al., Reference Palermo, Irurzun, Gogorza, Sinito, Ohlendorf and Zolitschka2019). Just three previous investigations used magnetic measurements as indicators of environmental changes (Lisé-Pronovost et al., Reference Lisé-Pronovost, St-Onge, Gogorza, Jouve, Francus and Zolitschka2014, Reference Lisé-Pronovost, St-Onge, Gogorza, Haberzettl, Jouve, Francus, Ohlendorf, Gebhardt and Zolitschka2015; Irurzun et al., Reference Irurzun, Orgeira, Gogorza, Sinito, Compagnucci and Zolitschka2014), and all are from Laguna Potrok Aike.
The goal of this study is to test the lake-level curve of Laguna Cháltel (Ohlendorf et al., Reference Ohlendorf, Fey, Massaferro, Haberzettl, Laprida, Lücke and Maidana2014) with the experimental model developed for Laguna Potrok Aike (Irurzun et al., Reference Irurzun, Orgeira, Gogorza, Sinito, Compagnucci and Zolitschka2014) to identify the magnetic indicator that best correlates with lake-level changes. Additionally, we examine a core from the littoral of the same lake to infer local palaeoenvironmental changes and discuss assumptions about wind strength in the catchment area of the lake.
SITE DESCRIPTION
Laguna Cháltel (49°58′S, 71°07′W) is a permanent lake located in a volcanic crater on the “La Siberia” plateau at 788 m above sea level (m asl) in the province of Santa Cruz, southern Patagonia, Argentina (Fig. 1). The region consists of Miocene sediments and Pliocene TiO2-rich basaltic lavas (Corbella and Lara, Reference Corbella, Lara and Rabassa2008). The flat highland plain in which Laguna Cháltel is lies between 50 and 850 m asl. The plateau is thinly covered by sandy to gravel-rich sediments of alluvial and colluvial origin, partially cemented by calcium carbonate, and rests on Tertiary and Cretaceous continental and marine sediments. This geological formation is of ancient fluvial origin with erosive reworking (López et al., Reference López, Rial, Elissalde, Llanos and Behr2011).

Figure 1. (color online) Locations of Laguna Cháltel and Laguna Potrok Aike in Argentinean Patagonia and locations of coring sites from Laguna Cháltel.
Climatically, Argentinian Patagonia is limited between the subpolar low-pressure belt around 60°S and two anticyclones around 30°S, one over the Atlantic Ocean and the other over the Pacific Ocean. Seasonal movements of these low- and high-pressure systems, together with the equatorial ocean currents, determine the regional rainfall patterns. In general, an increase in rainfall during winter is observed, and the Atlantic influence produces a uniform seasonal distribution of rainfall. In contrast, the humid air masses that come from the Pacific are limited by the Andes, which impose a natural barrier. Thus, most of the rain falls at the western (Chilean) side of the Andes, and the air becomes drier and warmer towards the eastern (Argentinean) side of the Andes (Paruelo et al., Reference Paruelo, Beltrán, Jobbágy, Sala and Golluscio1998). Due to this rain shadow effect, very dry conditions predominate in the catchment of Laguna Cháltel. Mean annual precipitation is around 155 mm and temperatures oscillate between 0 and 15°C (Argentina National Meteorological Service, www.smn.gob.ar, last accessed August 10, 2018). The climate is also influenced by the Southern Hemisphere westerlies (SHW), which are stronger in summer and move northward and weaken in winter (Garreaud et al., Reference Garreaud, Vuille, Compagnucci and Marengo2009). In summary, the region is characterised by a cool-temperate and semiarid climate with strong winds, resulting in steppe and semidesert vegetation (Zolitschka et al., Reference Zolitschka, Schäbitz, Lücke, Corbella, Ercolano, Fey and Haberzettl2006).
The lake had a maximum depth of 45 m at the time of fieldwork (2004) and is almost circular in shape. Three occasional tributaries exist, two in the north and one in the south. Relicts of lacustrine terraces and white carbonate crusts indicate lake-level variations of several meters in the past. Terraces were found along the northern and western shores, and crusts predominate at the eastern and southern shores. These observations suggest a lake level ~10 m higher than the lake level of March 2004 (Ohlendorf et al., Reference Ohlendorf, Fey, Massaferro, Haberzettl, Laprida, Lücke and Maidana2014).
MATERIAL AND METHODS
This study was carried out on six sediment cores collected with a gravity corer in 2004 (CHA04/1, CHA04/2, CHA04/3, CHA04/4, CHA04/6, CHA04/8), as shown in Figure 1. The cores were split in half, photographed, and lithologically described. One half was continuously subsampled for magnetic measurements using cubic plastic boxes of 8 cm3. The samples were stored at 4°C until measurements were carried out. Detailed sedimentologic and geochemical analyses as well as dating of cores CHA04/2 and CHA04/4 were reported by Ohlendorf et al. (Reference Ohlendorf, Fey, Massaferro, Haberzettl, Laprida, Lücke and Maidana2014). Cores CHA04/1, CHA04/2, CHA04/3, and CHA04/4 were analysed for rock magnetism by Palermo et al. (Reference Palermo, Irurzun, Gogorza, Sinito, Ohlendorf and Zolitschka2019) to investigate whether these sediments fulfil the necessary conditions for RPI (Tauxe, Reference Tauxe1993). These cores are denoted in this study as Palermo central cores (Pcc). Additionally, for this study, cores CHA04/6 and CHA04/8 were studied for rock magnetism. Finally, the data from all cores were combined to make palaeoenvironmental inferences.
Magnetic measurements
At least three parameters or ratios were used to infer the main magnetic characteristics of the samples. These characteristics are usually classified as magnetic mineralogy, concentration, and grain size (Evans and Heller, Reference Evans and Heller2003). Magnetic mineralogy was studied using the following measurements:
• Curie temperature: Samples were heated from 30 to 700°C and cooled down to 30°C at the same rate in a MS2 k-T equipped with a furnace (Bartington Instruments).
• S-ratio (isothermal remanent magnetisation with a reverse field of 300 mT, IRM−300mT divided by SIRM): A direct field with increasing steps at room temperature was applied to the samples with a pulse magnetiser IM-10-30 (ASC Scientific) until saturation (SIRM) was reached, then a reversed field was applied. After each application of a field, the samples were measured with a JR6A Dual Spinner Magnetometer (AGICo).
• Remanent coercivity (BCR, the reversed field necessary to erase the SIRM): After saturation, as for the S-ratio, reversed fields were applied until SIRM was erased. This measurement was also carried out with a pulse magnetiser IM-10-30 (ASC Scientific) and was measured after every application of a field with a JR6A Dual Spinner Magnetometer (AGICo).
The concentration of magnetic minerals was inferred from the following measurements:
• Volumetric (k) and specific (X) low-frequency susceptibility: Measured with a MS2 Magnetic Susceptibility Meter (Bartington Instruments).
• ARM: Imparted with an alternating field (AF) demagnetiser with a partial ARM device pARM (Molspin) and measured with a JR6A Dual Spinner Magnetometer (AGICo). The direct field was 0.05 mT, and the AF peak was 100 mT.
• SIRM: Obtained with a direct field of 1.2 T using a pulse magnetiser IM-10-30 (ASC Scientific) and measured with a JR6A Dual Spinner Magnetometer (AGICo).
Magnetic grain size was estimated using interparametric ratios: ARM/k, SIRM/k, and ARM/SIRM. In all cases, when the ratios increased, the magnetic grain size decreased (Turner, Reference Turner1997).
RESULTS
Magnetic mineralogy
For all Curie temperatures measured for 11 samples from different cores (Fig. 2A), a first magnetic phase with a temperature of 530 ± 20°C was observed. In 60% of the analysed samples, a second magnetic phase with a temperature of 671 ± 19°C was found. The first magnetic phase corresponds to Ti-magnetite, with percentages of Ti ranging from 5% to 12% (Evans and Heller, Reference Evans and Heller2003). The second magnetic phase could either be maghemite or Ti-hematite. If maghemite is present, the end value of k after cooling should be lower than the initial value, and some samples show this behaviour (Palermo et al., Reference Palermo, Irurzun, Gogorza, Sinito, Ohlendorf and Zolitschka2019). In this case, transformation from maghemite to hematite is observed. If Ti-hematite is present, the end value of k after cooling (when the temperature reaches 30°C) is similar to the initial value, and other samples show this behaviour (Fig. 2B). The percentage of Ti in these samples ranges from 0 to 3% (Evans and Heller, Reference Evans and Heller2003).

Figure 2. (A) Curie temperatures of samples from Laguna Cháltel with 1-sigma error (black). Percentage of the initial value of k remaining at 580°C (dark red). (B) Exemplary temperature acquisition curves from sample 5 of core CHA04-6. (For interpretation of the references to color in this figure legend, the reader is referred to the web version of this article.)
Table 1 shows the mean values of all measured parameters from Pcc (Palermo et al., Reference Palermo, Irurzun, Gogorza, Sinito, Ohlendorf and Zolitschka2019) and this study (cores CHA04/6 and CHA04/8). All cores show very similar BCR and S-ratio values, which vary in a very narrow range. BCR values are between 38 and 43 mT, suggesting (Ti-)magnetite and/or maghemite as dominant magnetic minerals (Peters and Dekkers, Reference Peters and Dekkers2003). The S-ratio oscillates from 0.93 to 0.97, that is, very close to 1, also indicating the presence of soft magnetic minerals. For analysis of IRM curves, a direct signal analysis (DSA) was applied to the experimental IRM. The DSA method has been widely described by Aldana et al. (Reference Aldana, Laredo, Bello and Suárez1994, Reference Aldana, Costanzo-Álvarez, Gómez, González, Díaz, Silva and Rada2011) and has been used to analyse IRM curves obtained from archaeological samples (Rada Torres et al., Reference Rada Torres, Costanzo-Álvarez, Aldana, Suárez, Campos, Mackowiak-Antczak and Brandt2011), sedimentary rocks (Costanzo-Álvarez et al., Reference Costanzo-Álvarez, Aldana, Bayona, López-Rodriguez, Blanco, Elmore, Muxworthy, Aldana and Mena2012, Reference Costanzo-Álvarez, Rapalini, Aldana, Díaz, Kietzmann, Iglesia-Llanos, Cabrera, Luppo, Vallejo and Walther2019), soils (Costanzo-Álvarez et al., Reference Costanzo-Álvarez, Devesa-Rey, Aldana, Barral, López-Rodríguez and Andrade2017a), and synthetic samples (Costanzo-Álvarez et al., Reference Costanzo-Álvarez, Kryczka, Guerra, Aldana, Bolívar and Guzmán2017b). Figure 3A and B shows the experimental and fitted curves for the shallow sample of core CH04/2, as well as the spectral histogram obtained. From this histogram, it is possible to obtain mean coercivities (logB1/2) associated with the number and type of magnetic minerals, as well as their relative proportions for studied samples. In the example presented, three phases can be clearly identified, with logB1/2 equal to 0.65, 1.74, and 2.9.

Figure 3. (color online) (A) Experimental and fitted curve for IRM results. (B) Spectral histogram obtained for data from A. (C) Relative proportion of magnetic phases identified for core CHA04-2.
Table 1. All magnetic measurements and ratios from central cores previously studied (Pcc: CHA04/1, CHA04/2, CHA04/3 and CHA04/4) and from this work (CHA04/6 [central] and CHA04/8 [shoreline]) expressed as average ± 1sigma error.

a Pcc, Palermo central cores from Palermo et al. (Reference Palermo, Irurzun, Gogorza, Sinito, Ohlendorf and Zolitschka2019).
Altogether, five magnetic phases were obtained after applying the DSA method to the studied samples (Fig. 3). The hard-magnetic phase F5 (top component) was identified as hematite with a coercivity of 794 mT (logB1/2 = 2.9) (Kruiver et al., Reference Kruiver, Dekkers and Heslop2001; Heslop et al., Reference Heslop, Dekkers, Kruiver and Van Oorschot2002; Aldana et al., Reference Aldana, Costanzo-Álvarez, Gómez, González, Díaz, Silva and Rada2011; Costanzo-Álvarez et al., Reference Costanzo-Álvarez, Aldana, Bayona, López-Rodriguez, Blanco, Elmore, Muxworthy, Aldana and Mena2012). The percentages of this phase range from 0.5% to 6%. A higher amount of hematite was found in the bottom samples. Phases F4 and F3 correspond to Ti-magnetite and magnetite (Peters and Dekkers, Reference Peters and Dekkers2003; Costanzo-Álvarez et al., Reference Costanzo-Álvarez, Aldana, Bayona, López-Rodriguez, Blanco, Elmore, Muxworthy, Aldana and Mena2012), based on their coercivities, which are between 25 and 63 mT (logB1/2 = 1.4 and logB1/2 = 1.8, respectively). The variability can be attributed to a variation in magnetic grain sizes.
Finally, the two magnetic phases F2 and F1 (bottom component) with very low mean coercivity values (logB1/2 = 0.9 and logB1/2 = 0.64, respectively) are observed. Martín-Hernández et al. (Reference Martín-Hernández, Dekkers, Bominaar-Silkens and Maan2008) have obtained values of logB1/2 that range from 0.95 to 1.30 after fitting IRM curves of pyrrhotite crystals using the approaches of Kruiver et al. (Reference Kruiver, Dekkers and Heslop2001) and Egli (Reference Egli2003). Although the values obtained for phase F2 in our samples intersect with these values, no trace of this mineral was observed in the Curie curves. It is important to note that the DSA algorithm, as well as those of Kruiver et al. (Reference Kruiver, Dekkers and Heslop2001) and Egli (Reference Egli2003), is based on the description by Robertson and France (Reference Robertson and France1994) of IRM curves, using a cumulative log-gaussian approach, which are symmetric with respect to the pulse field. Skewed distributions at low applied fields might need an extra magnetic component to be properly fitted. Hence, the phase F2 component has no physical meaning and should be considered as part of F3. Consequently, phases F2, F3, and F4 are considered to be one phase. A phase with values as low as logB1/2 = 0.6, close to the values of the F1 phase (black), has been observed (Martín-Hernández et al., Reference Martín-Hernández, Dekkers, Bominaar-Silkens and Maan2008) and associated with thermal relaxations and/or magnetic interactions in the samples.
In summary, the magnetic mineralogy experiments made for the six cores from Laguna Cháltel indicate a dominating presence of Ti-magnetite with low percentages of Ti. The percentages of Ti-magnetite have a mean value of 95.4% and a standard deviation of 6.1%, qualifying this mineral as the main carrier of the remanence. The presence of a very low amount of maghemite/Ti-hematite cannot be discarded.
Correlation of central cores
To date the sediments of this study (CHA04/6 and CHA04/8), a correlation with cores CHA04/1, CHA04/2, CHA04/3, and CHA04/4 (Palermo et al., Reference Palermo, Irurzun, Gogorza, Sinito, Ohlendorf and Zolitschka2019) was performed. Particularly, the central core CHA04/6 is similar to Pcc, regardless of the parameter used (Table 1, top two lines). CHA04/6 represents two units. Unit A (0–9.1 cm) is composed of grey-brown sandy silt. The upper part is reddish brown, and a brownish-grey to black lamination is observed between 3.8 and 7.3 cm. The main minerals are quartz, feldspars, calcite, and phyllosilicates. Unit B (9.1–25 cm) consists of silt in light grey-brown layers with dispersed black sand grains. Around 21–22 cm there are thin brownish-grey to black laminations. The mineralogical composition is quartz, followed by calcite, feldspars, and phyllosilicates.
In general, specific magnetic susceptibility (χ, 10−8 m3/kg) is the preferred parameter for core correlation (Gogorza et al., Reference Gogorza, Sinito, Lirio, Nuñez, Chaparro and Vilas2002; Irurzun et al., Reference Irurzun, Gogorza, Chaparro, Lirio, Nuñez, Vilas and Sinito2006; Achaga et al., Reference Achaga, Irurzun, Gogorza, Goguitchaichvili, Morales, Loponte and Sinito2017). Eight samples from core CHA04/4 and one sample of submersed aquatic mosses were dated using accelerator mass spectrometry 14C. Radiocarbon ages were calibrated with SHCal04 using the CALIB 5.0 software. The resulting age/depth model shows a sigmoidal shape with a basal age of 4520 cal yr BP for CHA04/4 (Ohlendorf et al., Reference Ohlendorf, Fey, Massaferro, Haberzettl, Laprida, Lücke and Maidana2014). The ages were confirmed for cores CHA04/1, CHA04/2, CHA04/3, and CHA04/4 using PSV (Ohlendorf et al., Reference Ohlendorf, Fey, Massaferro, Haberzettl, Laprida, Lücke and Maidana2014; Palermo et al., Reference Palermo, Irurzun, Gogorza, Sinito, Ohlendorf and Zolitschka2019). The chronology of these sediments was then transferred to CHA04/6 using X for correlation (some of the tie lines are shown in Fig. 4). For the intermediate samples between two consecutive tie lines the following equation was used to infer the age:

where Agei.s. is the estimated age for intermediate samples; Depthi.s. is the depth of intermediate samples; Agel.t.l./Depthl.t.l. is the age/depth for the lower tie line and Ageu.t.l./Depthu.t.l. is the age/depth for the upper tie line.

Figure 4. (color online) Correlation tie lines between central cores from Laguna Cháltel. Data for Cha04/1–Cha04/4 are from Palermo et al. (Reference Palermo, Irurzun, Gogorza, Sinito, Ohlendorf and Zolitschka2019).
Because CHA04/6 is shorter than the other central cores, a basal age of only ~3000 cal yr BP could be obtained. The samples are 2 cm wide, and the depths correspond to the mean point. Taking this into account, the age for the last sample is between 2959 and 3107 cal yr BP.
Figure 5 shows the results for magnetic concentration parameters (X, ARM, and SIRM) and magnetic grain size (ARM/k, SIRM/k, and ARM/SIRM) after core correlation of the central cores (CHA04/1, CHA04/2, CHA04/3, CHA01/4, and CHA04/6). For concentration parameters, the three variables show the same trends, particularly from 4800 to 2500 cal yr BP, where the values are less dispersed. From 2500 to 500 cal yr BP, there is an increasing trend, which is more noticeable in ARM. From 500 cal yr BP to the present, ARM and SIRM show a tendency towards lower values, barely recorded by X. Magnetic grain-size indicators are also less dispersed from 4800 to 2500 cal yr BP, and a predominant low (coarse magnetic grain size) at 4000 cal yr BP is present in all interparametric ratios. ARM/k and ARM/SIRM have similar increasing and decreasing trends from 2500 cal yr BP to the present, while SIRM/k is only decreasing.

Figure 5. Parameters used to infer the concentration of magnetic minerals (right) and magnetic grain size (left) after correlation of all central cores. The arithmetic mean with 1 SD is also shown (dark red). (For interpretation of the references to color in this figure legend, the reader is referred to the web version of this article.)
Due to the similar behaviour obtained for every magnetic variable, an averaged curve was calculated for X, ARM, SIRM, ARM/k, SIRM/k, and ARM/SIRM for the central cores (Fig. 5). Owing to the unequal age distribution of the samples after core correlation, an interpolation every 50 yr was made to calculate the averages. The average curve closely follows the trend observed in the records, but changes are less abrupt due to the calculation of the mean values.
Correlation of littoral core (CHA04/8)
The magnetic mineralogy inferred by BCR and the S-ratio of the littoral core (CHA04/8) is the same as comparable with the central cores, but the other parameters are not even in the same range (see Table 1,two bottom lines). This indicates that the magnetic mineral that reaches all coring sites is the same but has different amounts and grain sizes closer to the shore. Some general trends between CHA04/8 and the central cores can be correlated using BCR and/or the S-ratio (Fig. 6, only the first parameter is shown). The basal age for core CHA04/8 is around 3212 cal yr BP. From 3200 to 2000 cal yr BP, the BCR of CHA04/8 follows the changes of the central core average, and from 2000 cal yr BP to the top, the values are very similar to those for CHA04/3.

Figure 6. (color online) Tie lines used for correlation of the littoral core CHA04-8 with central cores based on BCR. CHA04-3 is also shown.
DISCUSSION
Magnetic measurements
The central cores correlate very well, particularly from 4800 to 2500 cal yr BP. From 2500 cal yr BP to the present, all cores have the same trend, but values are more dispersed. According to the age/depth model (Ohlendorf et al., Reference Ohlendorf, Fey, Massaferro, Haberzettl, Laprida, Lücke and Maidana2014), the last 2500 yr have the lowest sedimentation rate. Thus, every value represents a mean of several decades. A greater dispersion is assumed, considering that fewer samples have been taken from this section. The interparametric ratios show a better correlation than the concentration parameters (Fig. 5). This result indicates that the magnetic grain size is the same for the central cores at the same depth, but the amount of magnetic minerals varies slightly from core to core. The coarser magnetic grain size was found at 4030 cal yr BP (around 52 cm depth), where the maximum of coarse sand grains of different colour is cemented by a carbonaceous matrix. At the same age, a higher number of total chironomids was counted, and the presence of the littoral chironomid Polypedilum was also observed (Ohlendorf et al., Reference Ohlendorf, Fey, Massaferro, Haberzettl, Laprida, Lücke and Maidana2014). High magnetic mineral concentrations were found between 4000 and 3500 cal yr BP (50–39 cm depth) and from 1000 to 500 cal yr BP (6–4 cm depth), where sandy silt was observed. These intervals also show a fine magnetic grain size based on interparametric ratios. The period 1500–300 cal yr BP (25–9 cm depth) is characterized by a low concentration of magnetic minerals of medium to fine magnetic grain size. This sector shows very low presence of sand in the sedimentary record, which is dominated by silt and clay grains (Ohlendorf et al., Reference Ohlendorf, Fey, Massaferro, Haberzettl, Laprida, Lücke and Maidana2014).
The differences observed in magnetic concentration and grain size between central lake cores and the nearshore core (CHA04/8) were expected. The concentration parameters are dependent on magnetic grain size in different ways. For example, magnetic susceptibility increases when magnetic grain size increases (Vázquez et al., Reference Vázquez, Ortega, Davies and Aston2010); ARM is better detected when the magnetic grain size is smaller (Turner, Reference Turner1997). Moreover, considering that there are always differences in grain size between the shore and the deep part of a lake (Graham and Rea, Reference Graham and Rea1980; Nichols, Reference Nichols2009), the correlation between central cores and the shoreline core (CHA04/8) should be made by considering only mineralogical changes. These are usually best associated with climatic changes experienced across the entire lake. The best magnetic mineralogical proxy is BCR according to the results observed in Table 1. Core correlation indicates an age of 3210 cal yr BP for the base of this core.
The analysis of magnetic mineralogy of the six cores using BCR, Curie temperatures, and the DSA method indicates that the sediments are composed mainly of Ti-magnetite. Only a low proportion (<5%) of maghemite and/or Ti-hematite has been recognized in a few samples. S-ratios are in a narrow range, suggesting that mainly soft magnetic minerals are present in the samples. Variations in proportion of Ti-magnetite suggest different magnetic grain sizes according to DSA (Aldana et al., Reference Aldana, Laredo, Bello and Suárez1994). Therefore, constant magnetic mineralogy was found for all coring sites for the last 4800 cal yr BP.
Test of the magnetic model to infer lake-level changes with central cores
The model for lake-level changes inferred from magnetic parameters (Irurzun et al., Reference Irurzun, Orgeira, Gogorza, Sinito, Compagnucci and Zolitschka2014) assumes that if a constant magnetic mineralogy is present, the changes in magnetic concentration are directly related to rainfall and/or meltwater runoff. More precipitation and related runoff transfer more minerogenic matter into the lake. Particularly arid regions or low vegetation coverage leave the catchment area more exposed to weathering or erosion. Laguna Cháltel is in a steppe, and semidesert vegetation was found around the lake (Ohlendorf et al., Reference Ohlendorf, Fey, Massaferro, Haberzettl, Laprida, Lücke and Maidana2014), so more rainfall could transport more minerogenic matter. According to Nichols (Reference Nichols2009), grain size decreases from littoral to profundal zones in lake basins. Regarding magnetic grain size, the model predicts a coarser magnetic grain size (low interparametric values) for a low lake level because the shore is near to the coring site. Conversely, if the lake level is high, the magnetic grain size would be smaller (high interparametric values) and the shore is farther away from the coring site. Accordingly, high concentrations of small magnetic grains are expected for the central part of the lake when the lake level rises and vice versa.
If a different magnetic mineralogy is detected, the samples or sections must be analysed separately and the model cannot be applied. Irurzun et al. (Reference Irurzun, Orgeira, Gogorza, Sinito, Compagnucci and Zolitschka2014) found some periods where greigite was the main magnetic mineral in Laguna Potrok Aike. Those periods were analysed in detail to conclude that stratification of the lake was the cause of the greigite formation. But for periods of different magnetic mineralogy, the model using magnetic concentration and magnetic grain size cannot be used. For those cases, a combination of analysis from other disciplines is needed to arrive at conclusions about variations in hydrology and/or environmental changes.
As stated before, all magnetic analyses indicate homogeneous magnetic mineralogy. This is fundamental for the application of the model proposed for Laguna Potrok Aike (Irurzun et al., Reference Irurzun, Orgeira, Gogorza, Sinito, Compagnucci and Zolitschka2014) in the entire period covered by the cores. Consequently, the hydrologic changes observed in Laguna Cháltel (Ohlendorf et al., Reference Ohlendorf, Fey, Massaferro, Haberzettl, Laprida, Lücke and Maidana2014) only reflect variations in the amount of magnetic material (concentration parameters) or their magnetic grain sizes, either of which is another requirement for the use of the Laguna Potrok Aike magnetic proxy model.
Ohlendorf et al. (Reference Ohlendorf, Fey, Massaferro, Haberzettl, Laprida, Lücke and Maidana2014) developed a lake-level curve for the last 4800 cal yr BP (Fig. 7) that makes it easy to test the palaeomagnetic model for lake-level changes developed for Laguna Potrok Aike. The Pearson correlation coefficient (r) was used as an indicator of the magnetic proxy that compares best with the lake-level reconstruction by Ohlendorf et al. (Reference Ohlendorf, Fey, Massaferro, Haberzettl, Laprida, Lücke and Maidana2014; Fig.7).

Figure 7. Average magnetic concentration parameters (brown, left) and magnetic grain size (brown, right) for the central lake basin cores, lake-level variations (according to Ohlendorf et al., Reference Ohlendorf, Fey, Massaferro, Haberzettl, Laprida, Lücke and Maidana2014; blue), and Pearson correlation coefficients (r) for each comparison. (For interpretation of the references to color in this figure legend, the reader is referred to the web version of this article.)
All r-values are positive, confirming that the model works. The best correlations are obtained for ARM/k (0.85), ARM (0.86), and ARM/SIRM (0.94). ARM/SIRM follows the variations proposed by Ohlendorf et al. (Reference Ohlendorf, Fey, Massaferro, Haberzettl, Laprida, Lücke and Maidana2014) very closely. However, the last 1000 cal yr BP shows one major difference: the lake-level high stand observed at 150 cal yr BP seems to be displaced by 350 yr for the ARM/SIRM record. ARM is also a good proxy to infer lake-level variation, but from 4800 to 4000 cal yr BP the general trend in hydrologic changes is detected less precisely. ARM/k has a good Pearson coefficient too (0.85), but from 3960 to 2150 cal yr BP the average from central cores is higher than the lake level suggests. SIRM and X have the same trend as the lake-level curve from 2700 to 500 cal yr BP, when the coring site was under 19 to 41 m of water. SIRM/k shows an almost inverse correlation with a low value for r (−0.26).
ARM is more selective than SIRM to distinguish between fine to coarse magnetic particles. Moreover, X results from the contribution of all magnetic grains and mineralogies (Thompson and Oldfield, Reference Thompson and Oldfield1986; Turner, Reference Turner1997). Considering that Palermo et al. (Reference Palermo, Irurzun, Gogorza, Sinito, Ohlendorf and Zolitschka2019) found pseudo–single domain grains of ferrimagnetic minerals in four of the five central cores analysed, the best correlation coefficients are expected to be ARM, ARM/k, and ARM/SIRM. The presence of ferromagnetic pseudo–single domain grains also explains the low r obtained for X, SIRM, and SIRM/k.
Comparison of magnetic proxies with lake-level changes for the shore core
As in the study for the central cores, the logs of magnetic concentration and grain size were compared with the lake-level variations proposed by Ohlendorf et al. (Reference Ohlendorf, Fey, Massaferro, Haberzettl, Laprida, Lücke and Maidana2014). However, in the case of CHA04/8, some extra considerations must be taken into account. First, its position in the lake basin is 25 m above CHA04/2. Therefore, between 3200 and 2350 cal yr BP, CHA04/8 was not covered by water and was probably part of the northwestern tributary (Fig. 8A). This period is called the river lake period in Figure 9. From 2350 to 1620 cal yr BP, CHA04/8 was nearshore, and the coring site was covered only by a shallow layer of water (Fig. 8B). This period is called the shallow lake period in Figure 10. From 1620 cal yr BP to the present, CHA04/8 was under deeper water, and the coast was farther away from the coring site (Fig. 8C). This period is called the deep lake period in Figure 9.
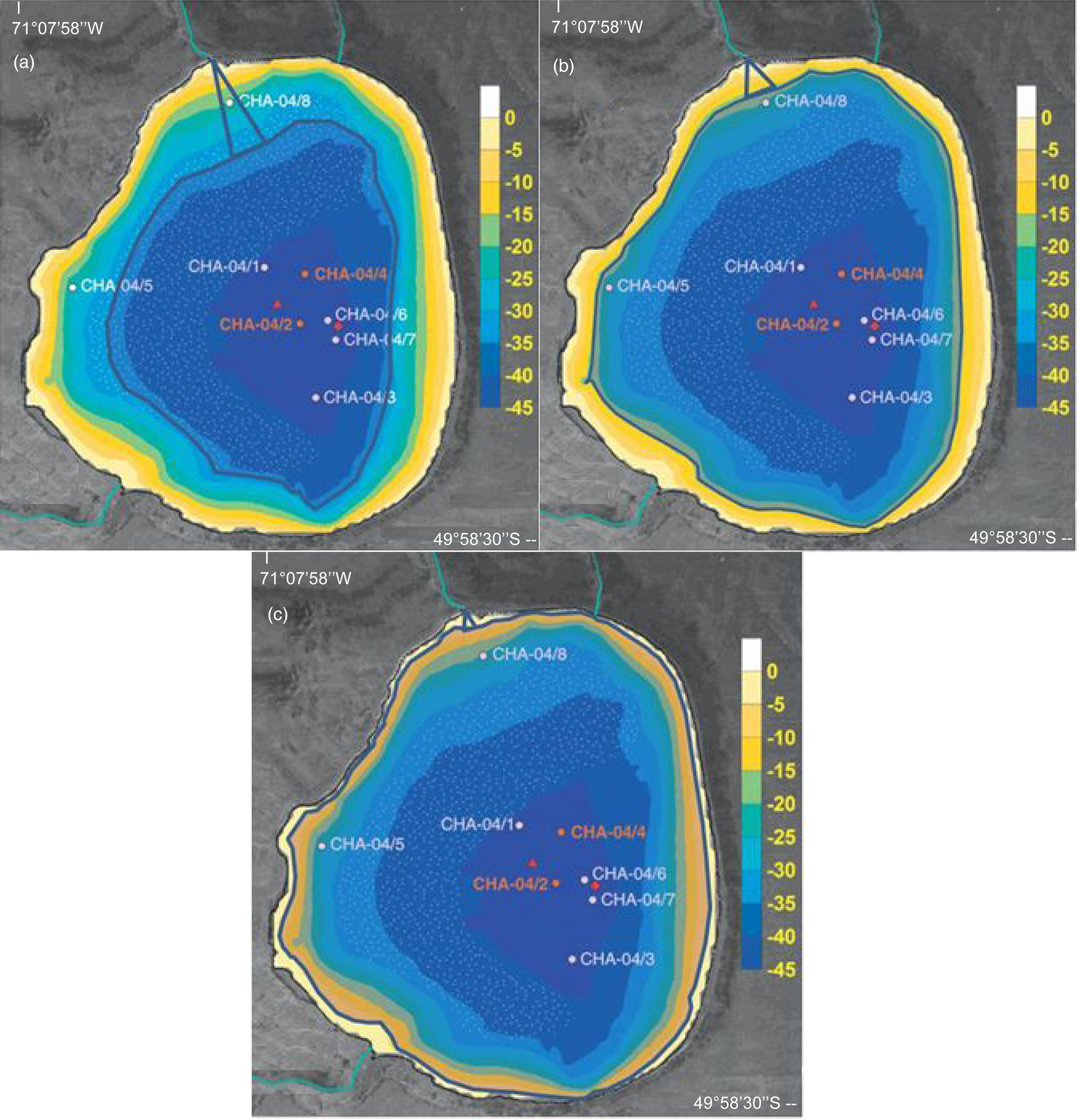
Figure 8. Lake-level schematic for three different time slices according to the definition of lake periods on the coring site of CHA04/8. The positions of the lake levels are indicated by thick blue lines. (A) River lake period (3210 cal yr BP). (B) Shallow lake period (1890 cal yr BP). (C) Deep lake period (1080 cal yr BP) (modified after Ohlendorf et al., Reference Ohlendorf, Fey, Massaferro, Haberzettl, Laprida, Lücke and Maidana2014). (For interpretation of the references to color in this figure legend, the reader is referred to the web version of this article.)

Figure 9. Lake-level changes since 3300 cal. BP (blue) according to Ohlendorf et al. (Reference Ohlendorf, Fey, Massaferro, Haberzettl, Laprida, Lücke and Maidana2014) with magnetic concentration parameters and magnetic grain-size ratios (pink). Pearson correlation coefficients (r) are shown. (For interpretation of the references to color in this figure legend, the reader is referred to the web version of this article.)

Figure 10. Averaged ARM vs. averaged ARM/SIRM. Data points located outside the 95% confidence intervals are encircled by pink ellipsoids. (For interpretation of the references to color in this figure legend, the reader is referred to the web version of this article.)
Second, the model tested for the central cores cannot be applied to a coring site in the river lake period, because it is designed for lacustrine cores. The concentration parameters show different patterns, with several highs and lows at different ages (Fig. 9, left). The interparametric ratios seem to have similar changes. All of them start with a low value (coarse magnetic grain size), then show higher values (small magnetic grain size), and finally decrease again at around 2490 cal yr BP (Fig. 10, right). Even if the model cannot be applied because the core was out of the lake basin during this period, changes in grain size can be used to identify the strength of inflow. Small grains need low energy to not be moved towards the centre of the lake. This suggests that around 3000 to 2900 cal yr BP less runoff was derived from the northwestern canyon.
In the shallow lake period, as for CHA04/6, the best magnetic proxies are ARM and ARM/SIRM. Both provide highest r-values: 0.64 and 0.87, respectively. The other magnetic grain-size indicator, SIRM/k, shows a behaviour similar to ARM/SIRM but does not follow the changes in lake level as clearly as ARM/SIRM. Instead, X and SIRM show an inverse behaviour to lake-level variations with r-values of −0.33 and −0.2, respectively.
In the deep lake period, all r-values are negative, and this inverse correlation is again best described by ARM for concentration parameters (−0.8) and by ARM/SIRM for magnetic grain-size indicators (−0.77). X (−0.52) and SIRM (−0.54) have a similar trend compared with ARM, but r is much lower. In the case of ARM/k (−0.73), the trend since 1345 cal yr BP is equal to ARM/SIRM. SIRM/k does not show any significant correlation (−0.07).
Since 2350 cal yr BP, water has covered the coring site, and the model can be considered operational. Thus, the proximity to the shore and the amount of runoff from the northwestern canyon can be estimated. If we consider the hypothesis of the river lake period (small grains in low-energy environments) since 2350 cal yr BP, correlation values are the result of a combined lake–river influence. From 2350 to 1620 cal yr BP(shallow lake period), the river model shows only a minor effect on the proxies considering that correlations are >0.5 (r = 0.64 for ARM and 0.87 for ARM/SIRM) and the model of lake-level variations is dominant. From 1620 to 1080 cal yr BP (deep lake period), not only are correlation values highly negative, but ARM and ARM/SIRM also show a marked trend towards initial values when the coring site was part of the river lake period. This could be an indication of a high-energy environment, where fluvial runoff from the northwestern canyon was stronger than during the previous period. Due to low sedimentation rates, only three samples were obtained from CHA04/8 since 1080 cal yr BP. Despite this, there is a long-term trend that seems to follow the lake-level variations proposed by Ohlendorf et al. (Reference Ohlendorf, Fey, Massaferro, Haberzettl, Laprida, Lücke and Maidana2014) but with coarse magnetic grain size and low concentrations,suggesting a combination of the magnetic model of lake-level variations with the riverine origin of sediments.
Palaeoenvironmental evolution using magnetic indicators
As noted by Ohlendorf et al. (Reference Ohlendorf, Fey, Massaferro, Haberzettl, Laprida, Lücke and Maidana2014), most of the meteorological and catchment factors are unknown due to the remoteness of Laguna Cháltel. In consequence, past meteorological conditions are hard to infer, but some environmental conditions can be obtained based upon the information derived from magnetic parameters.
The most noticeable low in lake level is found around 4000 cal yr BP. All parameters, magnetic or nonmagnetic, indicate the same situation: the driest event for Laguna Cháltel during the last 4700 yrs. At Laguna Potrok Aike, only a small regression of the lake level is observed, but it is superimposed on an increasing lake-level trend (Irurzun et al., Reference Irurzun, Orgeira, Gogorza, Sinito, Compagnucci and Zolitschka2014).
The fact that the ARM/SIRM ratio is a better indicator of changes in lake level than ARM is attributed to different amounts of magnetic minerals of the same size being delivered via a process different from runoff. Considering that glaciers are far from the lake and cannot be considered as a source of mineral grains, the other sediment source could be wind. This is more likely, as Laguna Cháltel is strongly influenced by the SHW. If no wind effect was present, both data sets (ARM/SIRM and ARM) should be within the prediction bands (Fig. 10, 95% confidence interval). Two periods are marked where the data are outside these confidence bands. From 3700 to 3630 cal yr BP, the concentration is higher, indicating more minerogenic influx and therefore suggesting stronger winds. Highest SIRM values are recognized during this period, indicating a larger volume of magnetic minerals with a coarse grain size reaching the central zone of the lake. From 3700 to 3400 cal yr BP, Ohlendorf et al. (Reference Ohlendorf, Fey, Massaferro, Haberzettl, Laprida, Lücke and Maidana2014) detected a Ti/coh peak indicating an increase of clastic matter transfer into Laguna Cháltel and high sedimentation rates. Lisé-Pronovost et al. (Reference Lisé-Pronovost, St-Onge, Gogorza, Haberzettl, Jouve, Francus, Ohlendorf, Gebhardt and Zolitschka2015) found high-intensity winds around 3500, 2000, and 1000 cal yr BP for Laguna Potrok Aike using the median destructive field of IRM as a wind-intensity indicator. This result was also confirmed by records from Chile (Schimpf et al., Reference Schimpf, Kilian, Kronz, Simon, Spötl, Wörner, Deininger and Mangini2011). Björck et al (Reference Björck, Rundgren, Ljung, Unkel and Wallin2012) used aeolian sand influx as a wind-intensity proxy and detected high winds and persisting SHW centred around 4000–3700 cal yr BP on Isla de los Estados (700 km southeast of Laguna Cháltel). Tonello et al. (Reference Tonello, Mancini and Seppä2009) attributed several peaks in annual precipitation to higher SHW intensity at Cerro Frías (125 km south west of Laguna Cháltel), the biggest between 3600 and 3100 cal yr BP. Ohlendorf et al. (Reference Ohlendorf, Fey, Massaferro, Haberzettl, Laprida, Lücke and Maidana2014) concluded that regional models of wind intensity lead to complex relationships, because local conditions need to be considered due to the location of Laguna Cháltel. In this sense, the only regional strengthening of the SHW recorded by magnetic parameters for the central part of the lake is around 3650 cal yr BP. Zolitschka et al. (Reference Zolitschka, Fey, Janssen, Maidana, Mayr, Wulf and Haberzettl2019) have proposed an expansion of the SHW belt, with stronger winds at the margins of the SHW belt and weaker winds in the central part. Taking this into account, Laguna Cháltel would be part of the northern margin of the SHW belt. Other records with high SHW either are not detected with this magnetic model or do not reach the latitude of Laguna Cháltel and only affect higher latitudes.
From 3000 to 2900 cal yr BP and from 2350 to 1620 cal yr BP, minor or no river contributions to the sediments are observed for CHA04/8. This suggests that the northwestern tributary was weaker. In addition, the lake levels inferred by Ohlendorf et al. (Reference Ohlendorf, Fey, Massaferro, Haberzettl, Laprida, Lücke and Maidana2014) during these periods show a less pronounced growth, and the ARM/SIRM ratio suggests a decrease. Paruelo et al. (Reference Paruelo, Beltrán, Jobbágy, Sala and Golluscio1998) indicate less rainfall during summer periods. On the other hand, fluvial runoff is stronger during snowmelt (Ohlendorf et al., Reference Ohlendorf, Fey, Massaferro, Haberzettl, Laprida, Lücke and Maidana2014). Consequently, this could be indicative of years with warm temperatures or extended summer conditions and/or less snow cover. Glasser et al. (Reference Glasser, Harrison, Winchester and Aniya2004) analysed glacier fluctuations in Patagonia and found drier conditions west of the Andes from 2400 to 1600 14C yr BP (2335–1405 cal yr BP), almost the same period discussed here.
Conversely, between 1620 and 1080 cal yr BP, the effect of the tributary seems to be strong considering that ARM/SIRM and ARM from core CHA04/8 are both negatively correlated with the lake-level increase observed in both, the magnetic model for the central cores and by Ohlendorf et al. (Reference Ohlendorf, Fey, Massaferro, Haberzettl, Laprida, Lücke and Maidana2014). Considering the assumptions for the previous period, the observations from 1350 ± 270 cal yr BP could indicate higher runoff during snowmelt and /or several years with low temperatures and more rainfall. Broad regional trends in glacier advances found between 1405 and 1273 cal yr BP (Glasser et al., 2014) support this assumption.
For the last century, the concentration of magnetic parameters obtained from ARM data is below the 95% prediction bands, indicating that a smaller amount of magnetic material with a grain size similar to that of other periods reached the lake. Unlike the previous case, we assume this is indicative of weaker winds. Lamy et al. (Reference Lamy, Kilian, Arz, Francois, Kaiser, Prange and Steinke2010) and Lisé-Pronovost et al. (Reference Lisé-Pronovost, St-Onge, Gogorza, Haberzettl, Jouve, Francus, Ohlendorf, Gebhardt and Zolitschka2015) found a trend towards low wind intensities for the last century. Björck et al. (Reference Björck, Rundgren, Ljung, Unkel and Wallin2012) found a trend towards low wind intensity for the last 150 yr. Garreaud et al. (Reference Garreaud, Lopez, Minvielle and Rojas2013) observed decreasing SHW between AD 1968 and AD 2001 around 45°S using high-resolution numerical simulations of data from several meteorological stations in Patagonia. Cúneo et al. (Reference Cúneo, Cerne and Llano2016) has observed a decrease in wind intensity by 10.4% for Trelew (840 km northeast of Laguna Cháltel) since AD 1997. In summary, several regional records indicate weaker SHW in recent years, but they differ in their timing of this decrease. Thus, we assume that this phenomenon started around AD 1890 for the Laguna Cháltel location.
CONCLUSIONS
The magnetic mineralogy of Laguna Cháltel is Ti-magnetite with percentages of Ti less than 12%, and Ti-hematite/maghemite less than 2.5%. The main magnetic mineralogy (Ti-magnetite) is also suggested by the S-ratio and BCR. The correlation of cores CHA04/6 and CHA04/8 was carried out based on concentration and mineralogy of magnetic parameters, yielding around 3000 and 3200 cal yr BP for the bottom sediments, respectively.
The lake-level model used for Laguna Potrok Aike (Irurzun et al., Reference Irurzun, Orgeira, Gogorza, Sinito, Compagnucci and Zolitschka2014) was applied to Laguna Cháltel to find the best magnetic proxy for lake-level variations. The requirement for having uniform magnetic mineralogy is the main characteristic of the model. Laguna Cháltel fulfils this requirement, as more than 90% of the main magnetic carrier is Ti-magnetite. Thus, increasing values of magnetic concentration imply more runoff, that is, the lake level should rise. Moreover, increasing values of interparametric ratios, which indicate smaller magnetic grain sizes, imply that the shore is farther away from the coring site, suggesting a rising lake level as well. These results were tested with the lake level inferred from a multiproxy sediment record of Laguna Cháltel (Ohlendorf et al., Reference Ohlendorf, Fey, Massaferro, Haberzettl, Laprida, Lücke and Maidana2014).
The central cores show that the best magnetic concentration parameter to infer a lake-level change is ARM. The best magnetic grain-size indicator to infer changes in lake level is ARM/SIRM, with this ratio being a better parameter for hydrologic variation than ARM. ARM versus ARM/SIRM shows periods when the concentration was higher than expected, indicating higher/lower influx of magnetic minerals of the same grain size than in other periods. This difference in concentration was used to estimate the strength of local winds. Higher/lower ARM values than expected can also be used as an indicator for wind strength. High wind strength was observed between 3700 and 3600 cal yr BP, consistent with other regional records. Low wind strength was observed for the last century, as indicated by other records from Patagonia.
The nearshore core shows a different behaviour compared with the central cores. At the beginning of the record, these sediments were not covered by water. Our analyses consider three zones: mainly river influence, shallow lake, and deep lake. The model for lake-level changes could only be applied to the period from 2350 to 1600 cal yr BP, that is, the lacustrine periods. The results also suggest that ARM and ARM/SIRM are the best proxies to infer changes in lake level. For the rest of the period covered by CHA04/8, the small magnetic grain size suggests low influx of minerogenic matter into the lake from the northwestern tributary. Particularly low runoff from this sector was suggested from 2900 to 300 cal yr BP, and high runoff at around 3200–3100, 2700–2450, and 1600–1080 cal yr BP. The last 1000 yr seem to indicate a combination of high runoff with a rising lake level. However, because of low sedimentation rates, only general observations can be made.
Considering the Pearson coefficient (r) between the magnetic parameters and the lake-level model proposed previously by Ohlendorf et al. (Reference Ohlendorf, Fey, Massaferro, Haberzettl, Laprida, Lücke and Maidana2014) is not exactly 1, some uncertainties should be considered. ARM/SIRM is very useful as an indicator of magnetic grain size when comparing different periods, but it does not provide an exact measure of magnetic grain size itself. In the samples in this study (8 cm3 of sediment material), a mix of different grain sizes was expected, as reflected in the error associated to the average (Fig. 7), particularly when mostly small grain size was found. Thus, small samples should be used to obtain more accurate hydrologic changes.
To conclude, in Patagonian lakes like Laguna Cháltel, where soft magnetic minerals such as Ti-magnetite are obtained as uniform magnetic mineralogy, the following magnetic ratios and parameters can be used as environmental indicators:
• ARM/SIRM ratio (preferably) and ARM as lake-level indicators in central cores.
• ARM versus ARM/SIRM as wind strength indicators in central cores.
• Magnetic grain size as runoff intensity of tributaries for cores taken from more proximal locations.
ACKNOWLEDGMENTS
We thank the late Cristóbal Kennard, Capt. Jorge D. Moreteau, and the staff of Instituto Nacional de Tecnología Agropecuaria (INTA), Río Gallegos, for their tireless assistance in organizing the logistics of fieldwork. The “puma hunter” Ávila is acknowledged for transporting equipment by horseback to the lake. This is a contribution to the project South Argentinean Lake Sediment Archives and Modelling (SALSA) within the framework of the German Climate Research Program DEKLIM (grants 01 LD 0034 and 0035) of the German Federal Ministry of Education and Research (BMBF). MAI wants to thank the team of Geomorphologie und Polarforschung (GEOPOLAR) and Thomas Frederichs at MARUM–Center for Marine Environmental Section University of Bremen (Germany) for the kind assistance she received during her stay in Bremen. MAI was supported by the Ministry of Science, Technology and Innovation Production (MINCYT, Argentina) and the German Federal Ministry of Education and Research (BMBF) in the framework of the bilateral project Palaeoproxy Records in Potrok Aike Maar Lake Sediments (52°S, Argentina): A Contribution to the ICDP Deep Drilling Project PASADO. The authors also want to thank the two reviewers Janine Roza and María Julia Orgeira and the associate editor Jaime Urrutia Fucugauchi for their helpful suggestions.