1. Introduction
The lower Cambrian Normanville Group crops out on the Fleurieu Peninsula (eastern Stansbury Basin), South Australia, and has been the focus of geological and stratigraphic research for more than 100 years (Madigan, Reference Madigan1925, Reference Madigan1926, Reference Madigan1927; Segnit, Reference Segnit1939; Campana et al. Reference Campana, Wilson and Whittle1955). Five lithostratigraphic units, including (in ascending order) the Mount Terrible Formation, Wangkonda Formation, Sellick Hill Formation, Fork Tree Limestone and Heatherdale Shale, have been recognized and represent a transgressive succession (Abele & McGowran, Reference Abele and McGowran1959; Daily, Reference Daily1963; Alexander & Gravestock, Reference Alexander and Gravestock1990; Jago et al. Reference Jago, Dyson and Gatehouse1994, Reference Jago, Zang, Sun, Brock, Paterson and Skovsted2006; Gravestock, Reference Gravestock, Drexel and Preiss1995; Gravestock et al. Reference Gravestock, Drexel and Preiss1995, Reference Gravestock, Alexander, Demidenko, Esakove, Holmer, Jago, Lin, Melnikova, Rozanov, Ushatinskaya, Zang, Zhegallo and Zhuravlev2001; Gravestock & Gatehouse, Reference Gravestock and Gatehouse1995; Jenkins et al. Reference Jenkins, Cooper and Compston2002). Regional correlations suggest that this largely carbonate succession spans the Terreneuvian Series through provisional Stage 3 of the Cambrian System. However, lack of faunal data and detailed sedimentological analyses (especially for the Heatherdale Shale) has significantly impeded resolution of the age range, lithofacies and depositional environments of this transition. Broadly, the lower Heatherdale Shale is mainly calcareous with siliciclastic input increasing in the upper levels, although fine-grained lithologies and thicknesses can vary laterally (Abele & McGowran, Reference Abele and McGowran1959; Daily, Reference Daily1963; Foster et al. Reference Foster, Cernovskis and O’Brien1985; Jago et al. Reference Jago, Dyson and Gatehouse1994). Fossils from the Heatherdale Shale are rare (particularly by comparison to rich coeval deposits in the Arrowie Basin), but include sponges, hyoliths, univalve molluscs, brachiopods, gastropods, bivalved arthropods (Isoxys and the bradoriids Sinskolutella cuspidata and an undetermined phasoiid), organic-walled microfossils (Sphaerocongregus) and rare conocoryphid trilobites (Abele & McGowran, Reference Abele and McGowran1959; Daily, Reference Daily1963; Jago et al. Reference Jago, Daily, Von Der Borch, Cernovskis and Saunders1984; Foster et al. Reference Foster, Cernovskis and O’Brien1985; Jenkins & Hasenohr, Reference Jenkins and Hasenohr1989; Jell et al. Reference Jell, Jago and Gehling1992; Jenkins et al. Reference Jenkins, Cooper and Compston2002; Jago et al. Reference Jago, Zang, Sun, Brock, Paterson and Skovsted2006; Paterson et al. Reference Paterson, Edgecombe, García-Bellido, Jago and Gehling2010; Jacquet et al. Reference Jacquet, Jago and Brock2016). The sparse fossil records from the Heatherdale Shale in combination with more siliciclastic and pure carbonate textures indicate a deeper shelf depositional environment compared with coeval deposits in the Arrowie Basin. This paper provides a detailed sedimentological, palaeontological and chemostratigraphic investigation of the upper Fork Tree Limestone to lower Heatherdale Shale transition in the Heatherdale Shale (HS) section in Rocky Gully located close to Myponga Beach, Fleurieu Peninsula, South Australia (Fig. 1), in order to improve regional chronostratigraphic correlation and better constrain the depositional setting of this important lower Cambrian package in the Stansbury Basin. Combined biostratigraphic and chemostratigraphic data suggest a Terreneuvian, Stage 2 age for this interval, correlated with the Micrina etheridgei Zone (Betts et al. Reference Betts, Paterson, Jacquet, Andrew, Hall, Jago, Jagodzinski, Preiss, Crowley, Brougham, Mathewson, García-Bellido, Topper, Skovsted and Brock2018), refining the stratigraphic relationships and relative ages of this lithostratigraphic package in the Normanville Group.

Fig. 1. Regional geology of the Normanville Group on the eastern side of the Fleurieu Peninsula at Stansbury Basin of South Australia. Locations of Heatherdale Shale (HS) section and spot sample at Carrickalinga Head indicated. Star on Main South Road is locality of dated volcanic ash layer.
2. Geology, stratigraphy and locality
Stratigraphic nomenclature used herein is based on previous mapping and geological descriptions (Abele & McGowran, Reference Abele and McGowran1959; Daily, Reference Daily1963; Alexander & Gravestock, Reference Alexander and Gravestock1990; Jago et al. Reference Jago, Dyson and Gatehouse1994, Reference Jago, Zang, Sun, Brock, Paterson and Skovsted2006; Gravestock et al. Reference Gravestock, Drexel and Preiss1995, Reference Gravestock, Alexander, Demidenko, Esakove, Holmer, Jago, Lin, Melnikova, Rozanov, Ushatinskaya, Zang, Zhegallo and Zhuravlev2001). In the eastern Stansbury Basin, the Normanville Group (Cambrian) reaches c. 1000 m in thickness and disconformably overlies the ABC Range Quartzite (Ediacaran) of the Wilpena Group (Gravestock et al. Reference Gravestock, Alexander, Demidenko, Esakove, Holmer, Jago, Lin, Melnikova, Rozanov, Ushatinskaya, Zang, Zhegallo and Zhuravlev2001). In turn, the Normanville Group is disconformably overlain by the lower–middle Cambrian Kanmantoo Group, a 8–10-km-thick succession of fine to coarse siliciclastic sedimentary rocks with minor, mostly unfossiliferous, carbonates (Jago et al. Reference Jago, Dyson and Gatehouse1994, Reference Jago, Zang, Sun, Brock, Paterson and Skovsted2006).
The Normanville Group is composed of (in ascending order): the Mount Terrible Formation, Wangkonda Formation, Sellick Hill Formation, Fork Tree Limestone and Heatherdale Shale (Abele & McGowran, Reference Abele and McGowran1959; Daily, Reference Daily1963; Gravestock et al. Reference Gravestock, Drexel and Preiss1995, Reference Gravestock, Alexander, Demidenko, Esakove, Holmer, Jago, Lin, Melnikova, Rozanov, Ushatinskaya, Zang, Zhegallo and Zhuravlev2001; Jago et al. Reference Jago, Zang, Sun, Brock, Paterson and Skovsted2006). The entire succession represents a transgressive systems tract (sequence set 1 of Gravestock, Reference Gravestock, Drexel and Preiss1995) beginning with a shallow subtidal environment at the base (Mount Terrible and Wangkonda formations). This is followed by a shallow mid- to outer-shelf setting (Sellick Hill Limestone), which transitions into the Fork Tree Limestone. The Fork Tree Limestone is a 300-m-thick package of massive, grey, clean, archaeocyathid-rich limestone (Gravestock et al. Reference Gravestock, Alexander, Demidenko, Esakove, Holmer, Jago, Lin, Melnikova, Rozanov, Ushatinskaya, Zang, Zhegallo and Zhuravlev2001) that was deposited in an outer carbonate ramp setting, comprising lower coalesced archaeocyathid–microbial bioherms, thin-bedded carbonates and upper mottled (brecciated) micritic limestone (Daily, Reference Daily1963; Alexander & Gravestock, Reference Alexander and Gravestock1990; Gravestock & Gatehouse, Reference Gravestock and Gatehouse1995; Cann et al. Reference Cann, Lower and Jago2014). The Fork Tree Limestone gives way to a deep-water mudstone-dominated, mid- to outer-shelf setting in the Heatherdale Shale (Alexander & Gravestock, Reference Alexander and Gravestock1990; Jago et al. Reference Jago, Dyson and Gatehouse1994; Gravestock & Gatehouse, Reference Gravestock and Gatehouse1995; Gravestock et al. Reference Gravestock, Alexander, Demidenko, Esakove, Holmer, Jago, Lin, Melnikova, Rozanov, Ushatinskaya, Zang, Zhegallo and Zhuravlev2001; Jenkins et al. Reference Jenkins, Cooper and Compston2002). The nature of the contact between the upper Fork Tree Limestone and the overlying Heatherdale Shale is generally considered to be conformable (Daily, Reference Daily1963; Jago et al. Reference Jago, Dyson and Gatehouse1994). The Heatherdale Shale crops out on the west side of the Fleurieu Peninsula, where faulting and folding have resulted in some of the succession being overturned (Abele & McGowran, Reference Abele and McGowran1959). The Heatherdale Shale was divided into two informal members by Abele & McGowran (Reference Abele and McGowran1959) in the Sellicks Hill – Carrickalinga Head area. The lower member consists of calcareous shale (up to c. 100 m thick) with recurrent interbeds of thin-bedded or nodular limestones and occasionally with large (up to 30 cm diameter) carbonate concretions in the upper levels. The upper member (280–300 m thick) is a siliciclastic succession consisting of fine phosphate-rich mudstones with apatite nodules (Abele & McGowran, Reference Abele and McGowran1959) and uniform grey to black pyritic shales suggestive of a reducing environment. The scattered, but common, occurrence of phosphatic nodules in the upper member suggests deposition below wave base (Gatehouse et al. Reference Gatehouse, Jago, Cooper, Jago and Moore1990; Cooper et al. Reference Cooper, Jenkins, Compston and Williams1992) during a transgression following deposition of the shallow-water carbonate ramp sediments of the Fork Tree Limestone (Jago et al. Reference Jago, Dyson and Gatehouse1994, Reference Jago, Zang, Sun, Brock, Paterson and Skovsted2006; Gravestock & Gatehouse, Reference Gravestock and Gatehouse1995; Gravestock et al. Reference Gravestock, Alexander, Demidenko, Esakove, Holmer, Jago, Lin, Melnikova, Rozanov, Ushatinskaya, Zang, Zhegallo and Zhuravlev2001).
Abele & McGowran (Reference Abele and McGowran1959, p. 319) suggested a possible break in sedimentation between the two members of the Heatherdale Shale given the abrupt shift from calcareous siltstones and shale to a completely fine-grained siliciclastic succession. Lateral thicknesses of the members can be variable and are interpreted to be the result of regional folding and faulting (Daily, Reference Daily1963, Reference Daily, Thompson, Daily, Coats and Forbes1976; Daily & Milnes, Reference Daily and Milnes1973; Jago et al. Reference Jago, Dyson and Gatehouse1994). In the Sellicks Hill area, at least 360 m of Heatherdale Shale (including lower and upper members) is exposed above the Fork Tree Limestone. However, at Myponga Beach and nearby localities, this is reduced to 60–100 m, and the calcareous silts and shales of the lower member of Heatherdale Shale are disconformably overlain by rocks of the Carrickalinga Head Formation (Kanmantoo Group) (Jago et al. Reference Jago, Dyson and Gatehouse1994). In the Sellicks Hill area, the top of the Heatherdale Shale is truncated by the Willunga Fault (Cann et al. Reference Cann, Lower and Jago2014).
The current investigation is based on detailed sampling of the HS section in the Rocky Gully area located c. 1.5 km south of Myponga Beach on the Fleurieu Peninsula (Fig. 1). The Fork Tree Limestone is well exposed in this area, and the base of the stratigraphic section intersects 15.6 m of the upper Fork Tree Limestone (corresponding to the upper mottled (brecciated) limestone of Abele & McGowran, Reference Abele and McGowran1959). The boundary between the Fork Tree Limestone and overlying Heatherdale Shale is transitional and conformable in the Rocky Gully area, although outcrop is intermittent (Betts et al. Reference Betts, Paterson, Jacquet, Andrew, Hall, Jago, Jagodzinski, Preiss, Crowley, Brougham, Mathewson, García-Bellido, Topper, Skovsted and Brock2018). In the HS section the Heatherdale Shale reaches a maximum thickness of 63 m (Fig. 2) and consists of thinly bedded, dark limestones interbedded with recessive yellow/khaki calcareous shales, muddy siltstone and mudstones (Fig. 3c–h) that become more dominant upsection. Rare large (> 20 cm) limestone nodules occur in upper parts of the section.
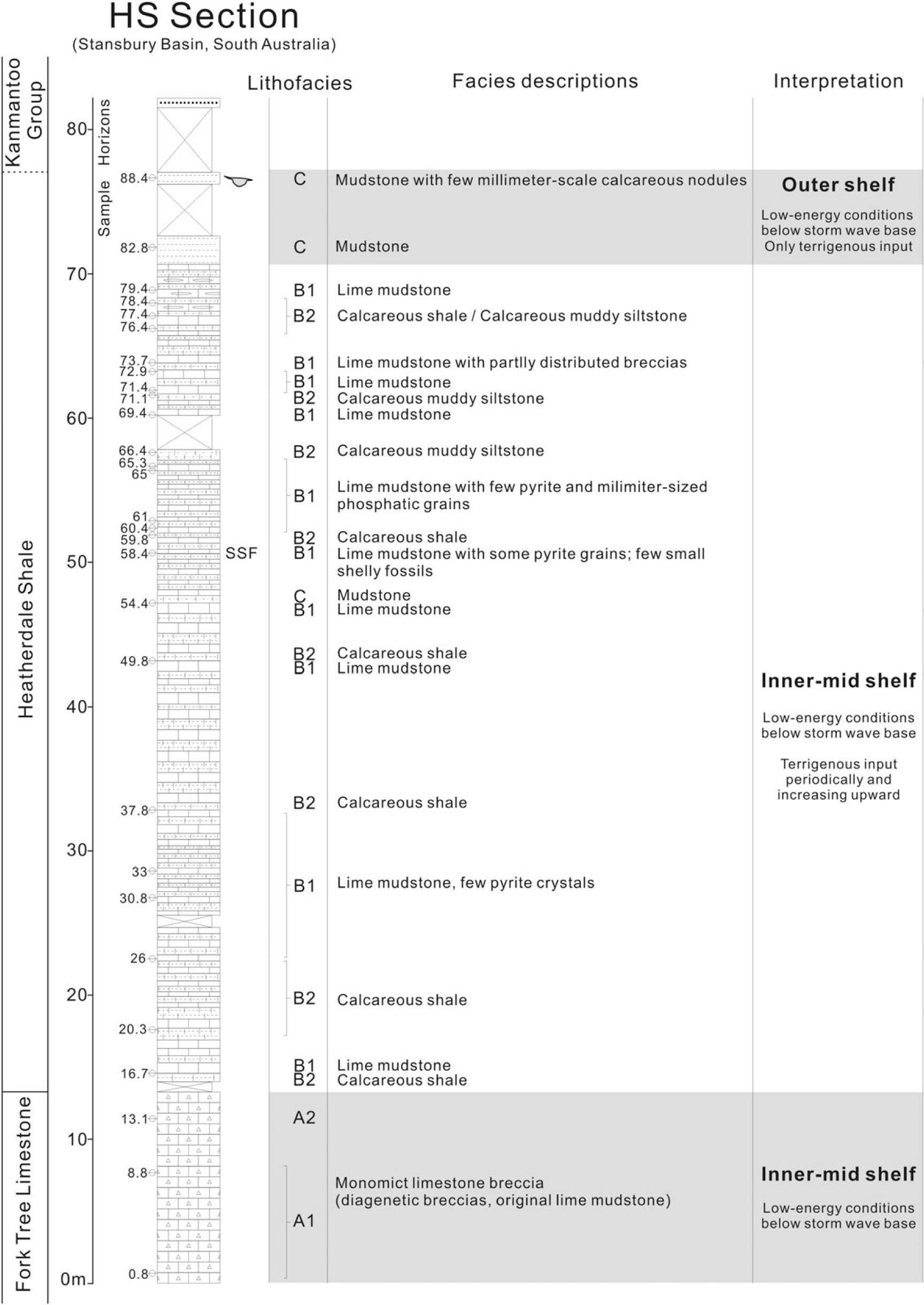
Fig. 2. Heatherdale Shale (HS) stratigraphic section through the Fork Tree Limestone and Heatherdale Shale on the Fleurieu Peninsula. The rock samples collected for polished slabs are indicated on the left side of column. Three identified lithofacies, description of main facies types and interpretation of depositional environments from different layers are included. Fossil-yielding horizons are indicated by fossil symbol and SSF on the column. The intervals HS/58.4 and HS/88.4, yielding small shelly fossil fragments and Isoxys, are indicated by fossil symbols. Lithofacies capital letters correspond to: A, monomict (diagenetic) limestone breccia (most breccias in A1 > 20 mm, breccias in A2 usually < 10 mm); B, interbeded lime mudstone (B1) with calcareous shale and calcareous muddy siltstone (B2); and C, mudstone.

Fig. 3. Rock slabs from HS section showing examples from the three lithofacies. (a) Monomict (diagenetic) limestone breccia of A1; note the good match of the clasts (Aubrecht & Szulc, 2006) and microfractures (HS/8.8, MPAL-0726). (b) Monomict (diagenetic) limestone breccia of A2; note millimetre-sized calcite-filled veins (HS/13.1, MPAL-0727). (c) Lime mudstone (B1) and inner fossil fragments indicated by yellow arrow and pyritic grain indicated by blue arrow (HS/58.4, MPAL-0728). (d) Lime mudstone (B1) and inner phosphatic grain indicated by yellow arrow (HS/60.4, MPAL-0729). (e) Lime mudstone with partly distributed micritic diagenetic breccias (HS/73.7, MPAL-0730). (f) Calcareous shale (HS/37.8, MPAL-0731). (g) Calcareous muddy siltstone (HS/71.1, MPAL-0732). (h) Mudstone (HS/88.4, MPAL-0733).
3. Age and correlation of the upper Fork Tree Limestone – lower Heatherdale Shale
3.a. Radiometric dating
A thin volcanic ash layer in the uppermost Heatherdale Shale on Main South Road, Fleurieu Peninsula (see Fig. 1; located at 35° 20’ 17.18″ S, 138° 27’ 54.55″ E) produced zircon grains that were dated using U–Pb series via chemical abrasion isotope dilution thermal ionization mass spectrometry (CA-TIMS). This produced a weighted absolute mean age of 514.98 ± 0.22 Ma (mean square weighted deviation or MSWD, 0.7; probability of fit, 0.57) interpreted as the maximum depositional age for the tuff (Betts et al. Reference Betts, Paterson, Jacquet, Andrew, Hall, Jago, Jagodzinski, Preiss, Crowley, Brougham, Mathewson, García-Bellido, Topper, Skovsted and Brock2018). This CA-TIMS date significantly refined the SHRIMP date of 522.0 ± 2.0 Ma reported for the same tuff (Jenkins et al. Reference Jenkins, Cooper and Compston2002) and provides an absolute temporal tie point for the uppermost part of the formation. Importantly, a 10-cm-thick green tuff layer in the Third Plain Creek Member of the Mernmerna Formation, Bunkers Range in the Arrowie Basin (MMF/58.1 locality at 31° 11′ 44″ S; 138° 52′ 32′’ E) also reported by Betts et al. (Reference Betts, Paterson, Jacquet, Andrew, Hall, Jago, Jagodzinski, Preiss, Crowley, Brougham, Mathewson, García-Bellido, Topper, Skovsted and Brock2018) has a weighted mean of 514.56 ± 0.13 Ma (MSWD, 1.2; probability of fit, 0.29), suggesting broadly contemporaneous volcanic activity in neighbouring basins.
3.b. Biostratigraphy
Small shelly fossils from the upper (mottled) Fork Tree Limestone and limestone nodules in the lower Heatherdale Shale at Rocky Gully belong to the Kulparina rostrata biozone based on the occurrence of Askepasma sp. cf. A. saproconcha, key accessory taxa Dailyatia ajax and D. macroptera (Betts et al. Reference Betts, Paterson, Jago, Jacquet, Skovsted, Topper and Brock2016, Reference Betts, Paterson, Jacquet, Andrew, Hall, Jago, Jagodzinski, Preiss, Crowley, Brougham, Mathewson, García-Bellido, Topper, Skovsted and Brock2018). Alexander & Gravestock (Reference Alexander and Gravestock1990) reported the presence of a single specimen of Micrina etheridgei from loose float material near the top of the Fork Tree Limestone in Rocky Gully, suggesting that the lower part of the M. etheridgei Zone occurs in the transitional succession into the lower Heatherdale Shale. However, detailed sampling by Betts et al. (Reference Betts, Paterson, Jacquet, Andrew, Hall, Jago, Jagodzinski, Preiss, Crowley, Brougham, Mathewson, García-Bellido, Topper, Skovsted and Brock2018) and in this study through this succession has failed to replicate this discovery. An undescribed species of Isoxys (Paterson et al. Reference Paterson, Edgecombe, García-Bellido, Jago and Gehling2010) was reported from mudstone in the upper part of the HS section in Rocky Gully (Figs 2, 4a, b), which at least suggests a likely Cambrian Stage 3 position for this level based on the known distribution of Isoxys (Fu et al. Reference Fu, Zhang, Budd, Liu and Pan2014; Paterson et al. Reference Paterson, García-Bellido, Jago, Gehling, Lee and Edgecombe2016). The only other taxon described from the lower Heatherdale Shale is the endemic helcionelloid macromollusc Tribelopoma amygdala Jacquet et al. Reference Jacquet, Jago and Brock2016, which has only been found in the Heatherdale Shale and so has no biostratigraphic signal.

Fig. 4. Isoxy sp. from the lower Heatherdale Shale. (a, b) Compressed mouldic specimens from HS/88.4, 76.6 m above base of HS section (MPAL-0734, MPAL-0735). (c–f) Separated rostral spines recovered from large limestone nodules at spot locality in the lower Heatherdale Shale at Carrickalinga Head (MPAL-0711, MPAL-0712, MPAL-0713, MPAL-0714).
The upper Heatherdale Shale is best represented in the Mount Terrible Gully and Sellick Hill area (Fig. 1), but fossils are generally rare in these mudrocks. Relatively poorly preserved hyolithids, sponges, brachiopods and gastropods have been reported sporadically from the upper Heatherdale Shale (e.g. Abele & McGowran, Reference Abele and McGowran1959), but only a few taxa have been formally described. These include the organic-walled microfossil Sphaerocongregus Moorman, 1974 (Foster et al. Reference Foster, Cernovskis and O’Brien1985) and a few poorly preserved specimens of a conocoryphid trilobite, Atops briandailyi from a locality c. 340 m above the base of the Heatherdale Shale near to the top of the unit (Jago et al. Reference Jago, Daily, Von Der Borch, Cernovskis and Saunders1984; Jenkins & Hasenohr, Reference Jenkins and Hasenohr1989; Jenkins et al. Reference Jenkins, Cooper and Compston2002), close to the dated volcanic tuff layer (see previous section).
4. Materials and methods
Detailed sedimentology and lithofacies assessment of the HS section in Rocky Gully is based on 29 oriented lithological samples collected systematically through the upper Fork Tree Limestone and lower Heatherdale Shale. Lithological samples were cut and polished at Macquarie University and polished slabs were imaged using a high-resolution Cannon photoscanner. Crack-out Isoxys fossils in mudrock were observed under an Olympus microscope, and photographed with a Canon D7 camera with auxiliary light to increase resolution of morphological structures, in the Palaeobiology Laboratories, Macquarie University. Small shelly fossils including bradoriids, Isoxys and phosphatic tubes were acquired by routine etching methods with diluted (10%) acetic acid (Jeppsson et al. Reference Jeppsson, Anehus and Fredholm1999) from limestone nodules collected from lower Heatherdale Shale at a cliff locality near Carrickalinga Head (35° 24’ 13″ S; 138° 19′ 57″ E). Microfossils were picked by hand from the acid-resistant residues, and selected specimens were photographed using a JEOL JSM 7100F-FESEM SEM at Macquarie University.
A total of 34 horizons were sampled for stable isotope analyses (13C and 18O). Carbonate powders for isotopic analyses were drilled from freshly cut rock surfaces, avoiding weathering rinds, veins and other secondary features. Approximately 30 mg of rock powder per sample was drilled using a low-speed Dremel 400-series diamond-tipped drill. All carbon and oxygen isotope samples were processed and analysed at the University of Adelaide. For each sample, 0.8 mg was loaded into Exetainer vials and purged with helium. Samples were reacted with 104% phosphoric acid for c. 1 hour at 70°C. The CO2 produced was then sampled and analysed using a Nu Instruments GasPrep system and Nu Horizon continuous-flow isotope-ratio mass spectrometer (CF-IRMS). The internal laboratory carbonate standards used to correct the data were ANU-P3 calcium carbonate (δ13C = +2.24, δ18O = –0.32), UAC-1 calcium carbonate (δ13C = –15.0, δ18O = –18.4) and IAEA CO-8 calcite (δ13C = –5.76, δ18O = –22.7). ANU-P3 was calibrated and provided by ANU, and the in-house standard UAC-1 was calibrated against the international standards IAEA CO-1, 8 and 9 as well as ANU-P3. Replicate analyses of the standards were generally better than ±0.1 for carbon and oxygen. Carbon and oxygen isotope values are reported in permil (‰) relative to the international standard VPDB. Oxygen isotope values are also reported in permil (‰) relative to the international standards VSMOW.
5. Results
5.a. Lithofacies classification
Lithofacies of the Fork Tree Limestone–Heatherdale Shale transition in Rocky Gully are grouped into three facies following the terminology of Flügel (Reference Flügel2010) (Fig. 2): monomict (diagenetic) limestone breccia (Lithofacies A); interbedded lime mudstone with calcareous shale and calcareous muddy siltstone (Lithofacies B); and mudstone (Lithofacies C). The upper Fork Tree Limestone is dominated by Lithofacies A, whereas the lower portion of the Heatherdale Shale alternates between Lithofacies B1 and B2, which are gradually replaced by Lithofacies C towards the top of the section. In outcrop, the calcareous mudstone (Lithofacies B1) usually weathers to a yellowish/khaki colour that contrasts with the much darker colour of fresh rock. Compared with calcareous mudstone, lime mudstone (Lithofacies B2) is much more resistant to weathering. In general, the section is composed of centimetre- and decimetre-scale parallel beds; however, the breccia within Fork Tree Limestone is massive, non-bedded and lacks visible sedimentary structures. The common occurrence of microfractures and millimetre-sized calcite-filled veins within Lithofacies A and B may indicate some tectonic influence after diagenesis (Jago et al. Reference Jago, Zang, Sun, Brock, Paterson and Skovsted2006; Gehling et al. Reference Gehling, Jago, Brock, Kruse, Betts, Jacquet, Paterson, Droser, García-Bellido, Langsford and Zang2016).
5.a.1. Lithofacies A: Monomict (diagenetic) limestone breccia
Sub-lithofacies A1 (Fig. 3a): This lithofacies includes monomict breccias consisting predominantly of dark-coloured, irregular, and angular to sub-angular micritic limestone clasts (> 65% of total rock composition). Microfractures along clast boundaries are common. Clasts may have boundaries with fine, sharp irregularities, or indistinct boundaries where the clast–matrix boundary is often gradational. Clasts range in size from 2 to 50 mm, with most > 20 mm. The breccia is mainly clast-supported. Matrix consists of a fine, yellow-brown calcisiltite to marly lime mudstone. Calcite silt grains in the matrix are angular to sub-rounded. No fossils were recovered from clasts or matrix.
Sub-lithofacies A2 (Fig. 3b): This lithofacies is similar to A1 as it is dominated by carbonate breccia. However, clast size is much smaller (c. 2–15 mm but usually < 10 mm), and ratio of clasts to matrix is closer to 50:50. Clasts are mainly angular to sub-rounded and are poorly sorted. The breccia is clast-supported and matrix-supported. The matrix consists of brown and brick-red micrite, microspar and calcisiltite. Millimetre-scale euhedral and semi-euhedral pyrite crystals are distributed within both clasts and matrix. Several millimetre-sized calcite veins cross-cut the breccia. Microfractures are common within larger clasts. Abutting clasts often have boundaries that interlock tightly with one another, while clasts suspended within the matrix tend to have gradational boundaries.
5.a.2. Lithofacies B: Interbedded lime mudstone with calcareous shale and calcareous muddy siltstone
Sub-lithofacies B1 lime mudstone (Fig. 3c–e): Grey to dark grey, homogenous micritic limestones. Rare pyrite grains (0.01–0.5 mm, HS/33, HS/58.4, HS/65), phosphatic grains (0.03–1.5 mm, HS/54.4, HS/60.4, HS/61) and fossil fragments (HS/58.4) are occasionally interspersed in the mud matrix. Microfractures and millimetre-sized calcite-filled veins are very common. One sample (Fig. 3e) is composed of mainly lime mud with partly distributed angular, very poorly sorted angular micritic limestone clasts 2–20 mm in size, with a matrix composed of silt-sized and sand-sized calcite grains (Fig. 3e).
Sub-lithofacies B2 calcareous shale (Fig. 3f) and calcareous muddy siltstone (Fig. 3g): These facies are characterized by fissile and finely laminated brown to light-grey mudstone and siltstone. Microfractures are very common with millimetre-sized calcite veins. Fossils and bioturbation are absent, which probably indicates low levels of nutrients and oxygen. The lower Heatherdale Shale is mainly composed of cyclic alternation between sub-lithofacies B1 and B2 with a minor bedding thickness fluctuation.
5.a.3. Lithofacies C: mudstone
The mudstone (Lithofacies C; Fig. 3h) within Heatherdale Shale mainly occurs in the top part of the section (HS/54.4, HS/82.8, HS/88.4). This mudstone is homogenous, yellowish-grey in colour, with rounded and lenticular calcareous nodules ranging from 6 to 15 mm in size. There is no bioturbation or other distinctive primary sedimentary structures, although these deposits do contain rare specimens of slightly mineralized Isoxys (Figs 2, 4a, b).
5.b. New faunal data
The lower Heatherdale Shale is generally poorly fossiliferous. Rare fossils from the Heatherdale Shale section in Rocky Gully consist of indeterminate small shelly fossil fragments (observed within polished slabs at HS/58.4; Figs 2, 3c) and crack-out specimens of Isoxys sp. from HS/88.4, some 63 m above the base of the formation (Figs 2, 4a, b). The Isoxys specimens are from the same locality previously mentioned in the Heatherdale Shale by Paterson et al. (Reference Paterson, Edgecombe, García-Bellido, Jago and Gehling2010), which can now be accurately placed in a stratigraphic context.
The cliff-forming lower member of the Heatherdale Shale is considerably thicker at Carrickalinga Head, reaching a thickness of c. 100 m (Abele & McGowran, Reference Abele and McGowran1959). Large (> 35 cm) ovoid phosphatic-calcareous nodules in the lower Heatherdale Shale occur at Carrickalinga Head (35° 24′ 13″ S; 138° 19′ 57″ E) and were reported to contain ‘Hyolithes’ and large sponge spicules (Abele & McGowran, Reference Abele and McGowran1959, p. 310). New processing of these nodules with acetic acid maceration techniques produced relatively poorly preserved, but identifiable, specimens of the bradoriids Sinskolutella cuspidata Betts et al. Reference Betts, Topper, Valentine, Skovsted, Paterson and Brock2014 (Fig. 5a–f), and an indeterminate phasoiid (Fig. 5g–i). Other taxa recovered from the insoluble residues include the rostral spines of Isoxys sp. (Fig. 4c–f), indeterminate hyolith conchs (Fig. 5n–o), hyolithelminthid tubes (Fig. 5j–m), sponge spicules (Fig. 5r) and other indeterminate small shelly fossils (Fig. 5p, q).

Fig. 5. Small shelly fossils from large limestone nodules in the Heatherdale Shale at spot locality in the cliff face at Carrickalinga Head (see Fig. 1). (a–i) Bradoriid arthropods: (a–f) Sinskolutella cuspidate: (a) lateral view, (c) pillow-like micro-ornamentation across inflated part of shield; (g–i) Phasoiid indet. from MPAL-0719: (i) fine reticulate micro-ornamentation around shield margin; (a, c) MPAL-0715; (b) MPAL-0716; (d, e) MPAL-0717; (f) MPAL-0718. (j–m) Hyolithelminthid tubes (MPAL-0720, MPAL-0721, MPAL-0722). (n–o) Indeterminate hyolith conchs (MPAL-0723). (p, q) Indeterminate small shelly fossils (MPAL-0724). (r) Hexactinellid sponge spicule (MPAL-0725).
5.c. Chemostratigraphy
δ13C chemostratigraphy through the upper Fork Tree Limestone and overlying lower Heatherdale Shale in the HS section captures mostly negative values (Fig. 6). In the cross-plot, a few data points plot separately to the rest of the data (Fig. 7). Five samples (HS/4.7, HS/8.2, HS/14.9, HS/61, HS/73.7) that exhibit unusual δ13C values (e.g. strongly covarying δ13C and δ18O) were omitted from the isotope curves (Figs 6, 7). The samples drilled from the brecciated limestone (HS/4.7, HS/8.2, HS/14.9) exhibit a covariation of δ18O and δ13C values, which may be related to strong brecciation and diagenetic fabrics developed in this part of the section (Figs 3a, 6). δ13C and δ18O signals also covary strongly at HS/61, suggesting diagenetic alteration at this horizon (Fig. 6). The sharp negative δ 13C value of HS/73.7 was also excluded from the curve as it was taken from within a partially brecciated limestone also influenced by diagenesis (Figs 3e, 6).

Fig. 6. Chemostratigraphic curves (δ18O and δ13C) from HS section through the uppermost Fork Tree Limestone and calcareous shales in the lower Heatherdale Shale. δ13C values interpreted to represent positive excursion III (maximum to +0.19‰) on the global chemostratigraphic scale. Orange dots and blue squares represent δ13C and δ18O data, respectively. Omitted samples are indicated in red colour.

Fig. 7. Cross-plot of δ18O‰ and δ13C‰ data for HS section. Red circles represent samples omitted from HS section.
There is an overall trend from strongly negative values in the lower part of the section (–2.50‰ at HS/0) to a weak positive peak (+0.19‰ at HS/40.4), then a steady decline to increasingly negative values toward the top of the section (–4.65‰ at HS/71.4), with a possible trend back to positive in the upper part of the section (–2.42‰ at HS/72.9) (Fig. 6). The δ18O data remain relatively stable at c. –8.00‰ for most of the sampled lower Heatherdale Shale, with a shift to –11.08‰ at the top of the sampled section.
6. Discussion
6.a. Depositional environment
Carbonate breccias may be formed by a wide variety of syn- and post-depositional processes (Flügel, Reference Flügel2010), including diagenetic or tectonic processes. Lithofacies A consists of diagenetic breccias likely caused by pressure dissolution of a formerly pure lime mudstone. Poorly stratified, discontinuous layers in Lithofacies A and lack of clear sedimentary structures argue against syn-depositional formation. The tightly interlocking clast boundaries also suggest dissolution as a result of diagenesis (Blendinger, Reference Blendinger2001). These processes also converted grey micrite to the marly yellow-brown and brick-red matrix between the clasts (Fig. 3a). Boundaries between clasts and the matrix are normally gradational, indicating irregular replacement margins and partial dissolution. Microfractures and veins cross-cutting limestone clasts indicate fracturing prior to brecciation and suggest post-diagenetic tectonic activity (Jago et al. Reference Jago, Zang, Sun, Brock, Paterson and Skovsted2006; Gehling et al. Reference Gehling, Jago, Brock, Kruse, Betts, Jacquet, Paterson, Droser, García-Bellido, Langsford and Zang2016). Based on the pure lime mudstone of the clasts, the depositional environment interpretation of Lithofacies A therefore suggests that this material was deposited below storm wave base in a quiet, inner- to mid-shelf environment (Fig. 2). Interbedded fine-grained siliciclastics and carbonates (Lithofacies B) of the lower Heatherdale Shale are poorly fossiliferous and, apart from fine laminations, lack distinctive sedimentary structures. This indicates deposition in a low-energy, inner- to mid-shelf environment below storm wave base (Figs 2, 3). Rare pyritic crystals (0.01–0.5 mm) suggest an episodic oxygen-deficient depositional setting. Pure siliciclastic mudstone (Lithofacies C) at the top of the section indicates a distal, deep-water depositional environment, likely on the outer shelf.
Overall, the depositional environment of the upper Fork Tree Limestone and lower Heatherdale Shale in the HS section represents a transgression whereby inner- to mid-shelf deposits gave way to deeper-water facies with decreasing carbonate and increasing fine siliciclastics (Figs 2, 3). The interbedded carbonate and siliciclastic deposits in Lithofacies B probably represent periodic small fluctuations of sea level in an overall transgressive system tract.
6.b. Integrated chronostratigraphy of the Heatherdale Shale
Betts et al. (Reference Betts, Paterson, Jacquet, Andrew, Hall, Jago, Jagodzinski, Preiss, Crowley, Brougham, Mathewson, García-Bellido, Topper, Skovsted and Brock2018) presented a high-resolution chronostratigraphic scheme for the lower part of the Cambrian System of South Australia based on integrated palaeontological (Betts et al. Reference Betts, Paterson, Jago, Jacquet, Skovsted, Topper and Brock2016, Reference Betts, Paterson, Jago, Jacquet, Skovsted, Topper and Brock2017), chemostratigraphic and radiometric data collected from measured stratigraphic sections in the Arrowie Basin and the lower part of the Normanville Group in the eastern Stansbury Basin. Three new biozones were documented (in ascending order): Kulparina rostrata Zone, Micrina etheridgei Zone and Dailyatia odyssei Zone. Each of these biozones is intimately tied to a robust, synchronous chemostratigraphic curve that affords much more accurate correlation between regional successions, and with the global δ13C curve.
Carbonate development in the Stansbury Basin preceded that in the Arrowie Basin during Cambrian Age 2. Globally important fossils such as the micromollusc Watsonella crosbyi have been recovered from the upper part of the Mount Terrible Formation (Jacquet et al. Reference Jacquet, Brougham, Skovsted, Jago, Laurie, Betts, Topper and Brock2017), and the globally recognized ZHUCE (Zhujiaqing carbon isotope excursion) positive δ13C event has been recorded in the lower Normanville Group (upper Wangkonda and lower Sellick Hill Formation) (Betts et al. Reference Betts, Paterson, Jacquet, Andrew, Hall, Jago, Jagodzinski, Preiss, Crowley, Brougham, Mathewson, García-Bellido, Topper, Skovsted and Brock2018). The positive ZHUCE is followed by the negative SHICE (Shiyantou carbon isotope excursion) within Facies C of the Sellick Hill Formation (Betts et al. Reference Betts, Paterson, Jacquet, Andrew, Hall, Jago, Jagodzinski, Preiss, Crowley, Brougham, Mathewson, García-Bellido, Topper, Skovsted and Brock2018). In both the Arrowie and Stansbury basins, the nadir of the SHICE occurs below the lower boundary of the Kulparina rostrata Zone and, in the Normanville Group, the incoming of the globally widespread tommotiid taxon Sunnaginia imbricata (Betts et al. Reference Betts, Paterson, Jago, Jacquet, Skovsted, Topper and Brock2016; Jacquet et al. Reference Jacquet, Brougham, Skovsted, Jago, Laurie, Betts, Topper and Brock2017). As noted earlier, the upper Sellick Hill Formation and the entire Fork Tree Limestone sit within the Kulparina rostrata Zone, strongly supporting a pre-trilobitic, Terrenuevian Age 2 for all units of the Normanville Group below the Heatherdale Shale (Jacquet et al. Reference Jacquet, Brougham, Skovsted, Jago, Laurie, Betts, Topper and Brock2017; Betts et al. Reference Betts, Paterson, Jacquet, Andrew, Hall, Jago, Jagodzinski, Preiss, Crowley, Brougham, Mathewson, García-Bellido, Topper, Skovsted and Brock2018).
Betts et al. (Reference Betts, Paterson, Jacquet, Andrew, Hall, Jago, Jagodzinski, Preiss, Crowley, Brougham, Mathewson, García-Bellido, Topper, Skovsted and Brock2018) demonstrated the occurrence of the negative SHICE through the Sellick Hill Formation, with subsequent δ13C values gradually curving back towards 0.0‰ through the upper parts of the section. Although no isotopic data are presented for the Fork Tree Limestone in Betts et al. (Reference Betts, Paterson, Jacquet, Andrew, Hall, Jago, Jagodzinski, Preiss, Crowley, Brougham, Mathewson, García-Bellido, Topper, Skovsted and Brock2018), it is predicted that positive values equivalent to the global ‘II’ occur through the Fork Tree Limestone (Betts et al. Reference Betts, Paterson, Jacquet, Andrew, Hall, Jago, Jagodzinski, Preiss, Crowley, Brougham, Mathewson, García-Bellido, Topper, Skovsted and Brock2018). In the global chemostratigraphic curve, the II δ13C positive is rapidly followed by a negative oscillation (to c. –2‰ δ13C), which subsequently trends back to 0.0‰ (or very weakly positive values) associated with the global III positive δ13C event. This trend closely matches the δ13C data from the HS section where the upper Fork Tree Limestone has negative (–2.50‰) values, but with a gradual positive shift upsection, peaking at +0.19‰ at sample HS/40.4, 21.5 m above the base of the Heatherdale Formation. In the Arrowie Basin, excursions II and III occur in the Wirrapoiwe Limestone (BHG section) and Wilkawillina Limestone (MORO section) (Betts et al. Reference Betts, Paterson, Jacquet, Andrew, Hall, Jago, Jagodzinski, Preiss, Crowley, Brougham, Mathewson, García-Bellido, Topper, Skovsted and Brock2018). The peak δ13C values for excursions II and III in the BHG section are +2.9‰ and –0.3‰, respectively, whereas in the MORO section, excursion II has a peak value of +2.3‰ and III has a peak value of +0.2‰. The presence of a +0.19‰ peak in the HS isotopic curve closely matches the III peak in these sections, suggesting the carbon isotope curve in the lower Heatherdale Shale also represents the III δ13C positive excursion.
The positive excursions II (c. +1.5‰) and III (c. +0.2‰) are also recorded in Siberian sections through the Pestrotsvet Formation (Magaritz et al. Reference Magaritz, Holser and Kirschvink1986; Kirschvink et al. Reference Kirschvink, Magaritz, Ripperdan, Zhuravlev and Rozanov1991; Brasier et al. Reference Brasier, Khomentovsky and Corfield1993; Kouchinsky et al. Reference Kouchinsky, Bengtson, Pavlov, Runnegar, Val’Kov and Young2005), Krasnoporog Formation (Kouchinsky et al. Reference Kouchinsky, Bengtson, Pavlov, Runnegar, Torssander, Young and Ziegler2007) and Emyaksin Formation (Kouchinsky et al. Reference Kouchinsky, Bengtson, Missarzhevsky, Pelechaty, Torssander and Val’Kov2001, Reference Kouchinsky, Bengtson, Clausen and Vendrasco2013), as well as the Lie de Vin Formation in Morocco (Maloof et al. Reference Maloof, Schrag, Crowley and Bowring2005, Reference Maloof, Ramezani, Bowring, Fike, Porter and Mazouad2010). The peak δ13C values for excursions II and III reach up to c. +1 to +2‰ and c. 0.0‰, respectively, worldwide (Betts et al. Reference Betts, Paterson, Jacquet, Andrew, Hall, Jago, Jagodzinski, Preiss, Crowley, Brougham, Mathewson, García-Bellido, Topper, Skovsted and Brock2018). The timing for positive excursions II and III in Morocco are restricted between zircon 206U–238Pb ID-TIMS dates of 523.17 ± 0.16 Ma and 520.93 ± 0.14 Ma, respectively (Maloof et al. Reference Maloof, Ramezani, Bowring, Fike, Porter and Mazouad2010).
Fossils from the BHG and MORO sections in the Arrowie Basin constrain the III δ13C positive curve to the lower Micrina etheridgei Zone based on the synchronous presence of Askepasma toddense and the accessory taxa Dailyatia ajax and D. macroptera in the MORO section and all three taxa plus Micrina etheridgei in the BHG section. Apart from the published report of one specimen of Micrina etheridgei from a loose sample near the boundary between the Fork Tree Limestone and the lower Heatherdale Shale (Alexander & Gravestock, Reference Alexander and Gravestock1990), the lack of biostratigraphic guide fossils from the lower Heatherdale Shale has hampered detailed correlation. Illustrated rostra of Isoxys? sp. from Curramulka in the western Stansbury Basin (Bengtson et al. Reference Bengtson, Conway Morris, Cooper, Jell and Runnegar1990) are similar in size, shape and form to the specimens of Isoxys rostra recovered from large calcareous nodules in the lower Heatherdale Shale at Carrickalinga Head (Fig. 4c–f). Isoxys rostra from the Heatherdale Shale are also similar to those that occur in the Winnitinny Creek Member of the Wilkawillina Limestone (horizon 866.0 m) within the MORO section, just stratigraphically above the peak of δ13C excursion III (Betts, Reference Betts2012). In the Heatherdale Shale, Isoxys also occurs above δ13C excursion III in the HS section (HS/88.4). Further data regarding taxonomic affinities of Isoxys fossils from mainland South Australia will improve their application to biostratigraphy and correlation.
Sinskolutella cuspidata has been recovered from several sections in the Arrowie Basin (Betts et al. Reference Betts, Paterson, Jago, Jacquet, Skovsted, Topper and Brock2017), and is predominantly restricted to the M. etheridgei Zone. The stratigraphic relationship of the range of S. cuspidata to the δ13C curve is known in the BHG section in the central Flinders Ranges. Here, this taxon overlaps with, and extends stratigraphically above, the III δ13C positive peak and through the overlying CARE (Cambrian arthropod radiation isotope excursion). The fragmentary rostra of Isoxys and the bradoriid taxon Sinskolutella cuspidata in large carbonate nodules in the lower Heatherdale Shale (through which the ‘III’ excursion is observed) supports an upper Terreneuvian, Stage 2 (M. etheridgei Zone) for the sampled succession from the lower Heatherdale Shale (Figs 7, 8) (Peng et al. Reference Peng, Babcock, Cooper, Gradstein, Ogg, Schmitz and Ogg2012; Betts et al. Reference Betts, Paterson, Jacquet, Andrew, Hall, Jago, Jagodzinski, Preiss, Crowley, Brougham, Mathewson, García-Bellido, Topper, Skovsted and Brock2018). The presence of Isoxys sp. from uppermost mudrock (HS/88.4) above the III δ13C isotope event in the HS section (where isotope data are lacking) conforms with the global incoming of arthropods prior to the peak of the CARE event (Zhang et al. Reference Zhang, Ahlberg, Babcock, Choi, Geyer, Gozalo, Hollingsworth, Li, Naimark, Pegel, Steiner, Wotte and Zhang2017), and may also indicate an upper Micrina etheridgei Zone age (Cambrian Stages 2–3) for this part of the section. The lower Heatherdale Shale therefore correlates, at least in part, with the Wirrapowie Limestone and Wilkawillina Limestone in the Arrowie Basin (Fig. 8). The combination of biostratigraphic and chemostratigraphic data consistently suggests a late Terreneuvian to early Epoch 2 of the Cambrian for the lower Heatherdale Shale succession from HS section.
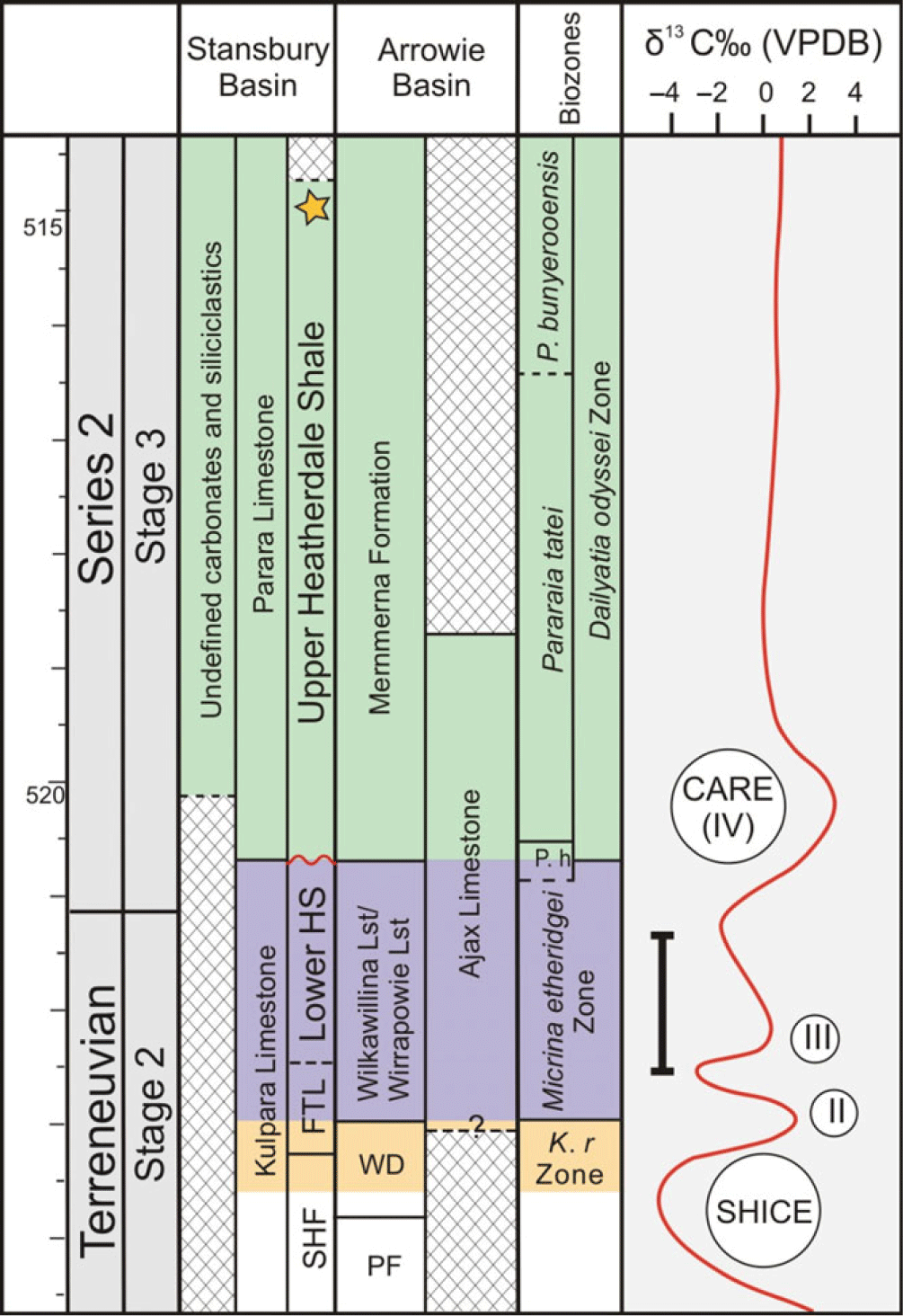
Fig. 8. Regional correlation chart showing lower Cambrian stratigraphic succession against the shelly fossil biozones in the Stansbury and Arrowie Basins (modified from Peng et al. Reference Peng, Babcock, Cooper, Gradstein, Ogg, Schmitz and Ogg2012; Betts et al. Reference Betts, Paterson, Jacquet, Andrew, Hall, Jago, Jagodzinski, Preiss, Crowley, Brougham, Mathewson, García-Bellido, Topper, Skovsted and Brock2018). Yellow star indicates the latest refined CA-TIMS geochronological date (514.98 ± 0.22 Ma) for tuff bed from top of the Heatherdale Shale Formation. The chemostratigraphic succession studied in this paper is indicated by a bold line by the left side of global δ13C excursion. Note the expanded lower boundary of the lower Heatherdale Shale.
The siliciclastic upper member of the Heatherdale Shale is interpreted to have been deposited in an oxygen-deprived outer-shelf setting. It is vastly different to the well-oxygenated, shallow-water carbonate depositional environments that prevailed in the Arrowie Basin at similar times. In contrast to the rich faunas from the Arrowie Basin, fossils from the upper Heatherdale Shale are much rarer. However, the presence of A. briandailyi from the upper Heatherdale Shale has been used by Jenkins et al. (Reference Jenkins, Cooper and Compston2002) to argue for potential correlation to the Pararaia janeae trilobite Zone in the Flinders Ranges based on the presence of a presumably closely related species, Atops rupertensis, in the upper Mernmerna Formation in the Arrowie Basin (Jell et al. Reference Jell, Jago and Gehling1992). However, Atops briandailyi is not part of the Pararaia janeae assemblage and none of the key taxa from the eponymous zone are known in the Heatherdale Shale. Atops briandailyi is more likely to be an older, perhaps ancestral, species that gave rise to A. rupertensis, which occurs in the P. janeae Zone. This is a more parsimonious suggestion given that the near-synchronous volcanic tuff horizon in the lower Mernmerna Formation in the Bunkers Range is well constrained biostratigraphically within the Pararaia bunyerooensis trilobite Zone (Paterson & Brock, Reference Paterson and Brock2007) and the Dailyatia odyssei small shelly fossil zone (Betts et al. Reference Betts, Paterson, Jago, Jacquet, Skovsted, Topper and Brock2017, Reference Betts, Paterson, Jacquet, Andrew, Hall, Jago, Jagodzinski, Preiss, Crowley, Brougham, Mathewson, García-Bellido, Topper, Skovsted and Brock2018). However, the numerical date for the tuff horizon (514.98 ± 0.22 Ma) in the uppermost Heatherdale Shale occurs c. 270 m above the Isoxys-bearing horizon in the HS section, suggesting that this stratigraphic interval (above the HS section) spans the P. huoi, P. tatei and P. bunyerooensis trilobite Zones and most of the Dailyatia odyssei shelly fossil Zone (Betts et al. Reference Betts, Paterson, Jago, Jacquet, Skovsted, Topper and Brock2016, Reference Betts, Paterson, Jacquet, Andrew, Hall, Jago, Jagodzinski, Preiss, Crowley, Brougham, Mathewson, García-Bellido, Topper, Skovsted and Brock2018).
7. Conclusion
The recent ID TIMS date from the tuff in the upper Heatherdale Shale (514.98 ± 0.22 Ma) is a critical chronostratigraphic tie-point for lower Cambrian strata in the Stansbury Basin, South Australia. However, establishing the relative age of adjacent units has been challenging, primarily due to rare fossil occurrences and carbonate-poor siliciclastics (particularly in the upper parts of the Heatherdale Shale) preventing high-resolution biostratigraphic and chemostratigraphic work. Here we present the first targeted study integrating sedimentological, palaeontological and chemostratgraphic data from the upper Fork Tree Limestone and lower Heatherdale Shale, embedding this succession within the recently established regional chronostratigraphic scheme (Betts et al. Reference Betts, Paterson, Jacquet, Andrew, Hall, Jago, Jagodzinski, Preiss, Crowley, Brougham, Mathewson, García-Bellido, Topper, Skovsted and Brock2018). Lithostratigraphic work identified three distinct lithofacies through the HS section: A, monomict (diagenetic) limestone breccia; B, interbedded lime mudstone with calcareous shale and calcareous muddy siltstone; and C, mudstone revealing a deepening-upwards environment, from inner- to mid-shelf to outer-shelf depositional environments. Compared with the Fork Tree Limestone, deeper palaeoenvironments of the lower Heatherdale Shale were likely stagnant and/or anoxic as evinced by pyritic shales, lack of fauna and bioturbation, and fine-grained deposits, which is also supported by former studies (Jenkins & Hasenohr, Reference Jenkins and Hasenohr1989; Gravestock et al. Reference Gravestock, Alexander, Demidenko, Esakove, Holmer, Jago, Lin, Melnikova, Rozanov, Ushatinskaya, Zang, Zhegallo and Zhuravlev2001; Jago et al. Reference Jago, Zang, Sun, Brock, Paterson and Skovsted2006; Jacquet et al. Reference Jacquet, Jago and Brock2016).
δ13C and δ18O curves from the Fork Tree Limestone and Heatherdale Shale in the HS section reveal the weakly positive III excursion (peak δ13C value +0.19‰) that occurs before the CARE δ13C positive excursion worldwide. δ13C data in combination with the bradoriid Sinskolutella cuspidata and Isoxys (fragmentary rostra and compressed, slightly mineralized examples) support a late Terreneuvian, Age 2 for the sampled succession of HS section at Rocky Gully. This corresponds to the M. etheridgei Zone, and correlates this unit with the Wirrapowie Limestone and Wilkawillina Limestone in Arrowie Basin. New data presented here demonstrate that the boundary between the Fork Tree Limestone and the overlying Heatherdale Shale is considerably older than previously estimated. This integrated study has vastly improved regional chronostratigraphic correlation and better constrained the depositional setting of this important lower Cambrian succession in the Stansbury Basin, South Australia.
Acknowledgements
We thank Tom Bradley for assisting with fieldwork. Manal Bebbington (Macquarie University) helped with rock cutting. Tony Hall (University of Adelaide) performed isotope analyses. Thanks to Yanlong Chen and Yue Liang (Northwest University) for their comments and suggestions. We thank the editor Bas Van de Schootbrugge and two anonymous referees for their constructive suggestions and comments. This work was supported by Macquarie University Research Fellowships (MQRF 2019–2022 to ZLZ); 1000 Talent Shaanxi Province Fellowship (to GAB); iMQRES from Macquarie University (to BL); National Natural Science Foundation of China (NSFC 41425008, 41720104002, 41621003, 41890844); Strategic Priority Research Program of the Chinese Academy of Sciences (XDB26000000); and the Ministry of Science and 111 project of Ministry of Education of China (D17013). FC warmly acknowledges the China Scholarship Council (CSC 201806970026) and Department of Geology, Northwest University for 20 months of research as an exchange PhD student with GAB at Macquarie University.
Conflict of interest
None.