Introduction
Hydrogen cyanide (HCN) is suggested as a central molecule in chemical evolution because it is proposed as a ‘chemical block’ of the three pillars of the prebiotic chemistry (Islam and Powner, Reference Islam and Powner2017). Several experiments have shown that HCN is an important precursor of organic molecules such as carboxylic acids, amino acids, purines, pyrimidines and other carbonyl compounds (Sanchez et al., Reference Sanchez, Ferris and Orgel1967; Draganić and Draganić, Reference Draganić and Draganić1980; Niketić et al., Reference Niketić, Draganić, Nešković, Jovanović and Draganić1983; Ferris and Hagan, Reference Ferris and Hagan1984; Schwartz et al., Reference Schwartz, Voet and Veen1984; Draganić et al., Reference Draganić, Draganić, Azamar, Vujošević, Berber and Negrón-Mendoza1985a; Borquez et al., Reference Borquez, Cleaves, Lazcano and Miller2005; Matthews, Reference Matthews and Seckbach2005; Ruiz-Bermejo et al., Reference Ruiz-Bermejo, Zorzano and Osuna-Esteban2013; Sutherland, Reference Sutherland2016).
However, one of the biggest problems in considering any primitive conditions is the availability of raw material in the environment and its concentration (Miller, Reference Miller1987). These are fundamental criteria to consider any primitive scenario and the kind of reactions that could have happened on the primitive Earth. Regarding this, several mechanisms could have contributed to the inventory of HCN on early Earth by the action of different energy sources (e.g. photolysis, shock waves, electrical discharges of lightning and volcanism; Ferris and Hagan, Reference Ferris and Hagan1984; Holm and Neubeck, Reference Holm and Neubeck2009; Tian et al., Reference Tian, Kasting and Zahnle2011; Parkos et al., Reference Parkos, Pikus, Alexeenko and Melosh2016; Ferus et al., Reference Ferus, Kubelík, Knížek, Pastorek, Sutherland and Civiš2017; Rimmer and Rugheimer, Reference Rimmer and Rugheimer2019). Likewise, the extraterrestrial input from meteorites and comets was possibly an important source of HCN (Matthews and Minard, Reference Matthews and Minard2006; Colín-García et al., Reference Colín-García, Negrón-Mendoza and Ramos-Bernal2009; Mumma and Charnley, Reference Mumma and Charnley2011; Pizzarello, Reference Pizzarello2012). In general, the mechanisms for the synthesis of HCN are based in reactions between an oxidizing agent (e.g. CO, CO2), a source of nitrogen (e.g. NH3, N2) and a reducing agent (e.g. H2, H2O) (for more details, see Ferris and Hagan, Reference Ferris and Hagan1984). In addition, cyanide species in the form of complexes with sulphur/ferrous ions could have been present in the primitive oceans or in the vicinity of hydrothermal systems (Mukhin, Reference Mukhin1974; Arrhenius et al., Reference Arrhenius, Arrhenius and Paplawsky1994; Keefe and Miller, Reference Keefe and Miller1996; Dzombak et al., Reference Dzombak, Ghosh and Wong-Chong2006; Holm and Neubeck, Reference Holm and Neubeck2009).
The concentration of HCN is a critical parameter. High concentrations (>0.1 mol l−1) could have favoured the polymerization of the molecule, while lower concentrations (<0.01 mol l−1) might have favoured its hydrolysis (Sanchez et al., Reference Sanchez, Ferris and Orgel1968; Miyakawa et al., Reference Miyakawa, Cleaves and Miller2002). Besides, these mechanisms are highly dependent on pH.
There is no agreement about the concentration of HCN on early Earth. For instance, it has been proposed that it was possible to reach high concentrations of HCN at the surface of the water (floating HCN patches >1 mol l−1; Fábián et al., Reference Fábián, Szőri and Jedlovszky2014). Other models propose that concentration per se in the primitive oceans was very low (10−10,–13 mol l−1) (Stribling and Miller, Reference Stribling and Miller1987; Miyakawa et al., Reference Miyakawa, Cleaves and Miller2002). Hence, it is necessary to consider primitive environments where the production of HCN was continuous and then chemical reactions could have taken place to increase the chemical complexity. One plausible scenario may be the hydrothermal systems.
Holm and Neubeck (Reference Holm and Neubeck2009) suggested a possible mechanism for HCN production under hydrothermal conditions from CH4, NH3 and other dissolved species (i.e. N2, CO). The main reactions are as follows:
(1) CH4 + NH3 → HCN + 3H2
(2) 2CH4 + N2 → 2HCN + 3H2
(3) CO + NH3 → HCN + H2O
In the same way, based on theoretical models, it has been proposed that HCN can coexist with their precursor molecules (e.g. CO, CO2, H2 and N2) under hydrothermal conditions (Shock, Reference Shock and Holm1992; Schulte and Shock, Reference Schulte and Shock1995; LaRowe and Regnier, Reference LaRowe and Regnier2008). Such species may be available in these environments (Table 1) when the hydrothermal fluids are mixed with seawater. Particularly, alkaline fluids, resulted from the serpentinization processes, could have an essential role in the release of several dissolved species and consequently in the formation of organic compounds (Konn et al., Reference Konn, Charlou, Holm and Mousis2015; McDermott et al., Reference McDermott, Seewald, German and Sylva2015). Brandes et al. (Reference Brandes, Boctor, Cody, Cooper, Hazen and Yoder1998) showed that N2 could be transformed into NO2, NO3 and ammonia in the presence of minerals under hydrothermal conditions. Additionally, abiotic nitrogen reduction could have taken place within primordial hydrothermal vents, supplying some ammonia for the synthesis of C-H-O-N compounds via abiotic processes (Schoonen and Xu, Reference Schoonen and Xu2001).
Table 1. Temperature, pH and dissolved species in fluids of some hydrothermal systems and seawater

Based on: §Charlou et al. (Reference Charlou, Donval, Douville, Jean-Baptiste, Radford-Knoery, Fouquet, Dapoigny and Stievenard2000); *Schrenk et al. (Reference Schrenk, Kelley, Bolton and Baross2004) and references therein.
Consequently, if HCN can be produced under hydrothermal conditions, it is crucial to study its stability and reactivity considering some of the available geochemical variables in hydrothermal systems, such as different pH values, high temperatures and in the presence of inorganic surfaces.
On the one hand, it is well known that both hydrolysis and polymerization of HCN are pH-dependent (Sanchez et al., Reference Sanchez, Ferris and Orgel1967; Ferris and Hagan, Reference Ferris and Hagan1984). Besides, it has been proven that some minerals adsorb HCN and/or increase its hydrolysis. This has been demonstrated theoretically and experimentally using ferrierite (Nanba et al., Reference Nanba, Obuchi, Akaratiwa, Liu, Uchisawa and Kushiyama2000), double-layered hydroxides (Zhao et al., Reference Zhao, Tian, Yan, Zhang and Ning2015), zeolites (Kotdawala et al., Reference Kotdawala, Kazantzis and Thompson2008; Demir et al., Reference Demir, Salmas, Ahunbay and Yurtsever2012), titanium oxides (Ma et al., Reference Ma, Wang, Wang, Ning, Jing and Cheng2017) and serpentinite and clays (Colín-García et al., Reference Colín-García, Ortega-Gutiérrez, Ramos-Bernal and Negrón-Mendoza2010; Colín-García et al., Reference Colín-García, Heredia, Negron-Mendoza, Ortega, Pi and Ramos-Bernal2014). Nonetheless, it is necessary to consider all these variables together.
Here, we present an experimental approach of the HCN thermolysis under a simple hydrothermal scenario. In this study, it was considered that it is possible to find relatively warm fluids, generated by the hydrothermal fluids upwelling in chimneys and their diffusion within the medium. The selected temperature (100°C) could be very likely present surrounding the hot chimneys; since it has been reported a temperature difference (50°C) on fluids in a centimetre scale, result of turbulent mixing and venting activity (Fornari et al., Reference Fornari, Shank, Von Damm, Gregg, Lilley, Levai, Bray, Haymon, Perfit and Lutz1998; Bates et al., Reference Bates, Lee, Tunnicliffe and Lamare2010; Mittelstaedt et al., Reference Mittelstaedt, Escartín, Gracias, Olive, Barreyre, Davaille, Cannat and Garcia2012). In the study of the reactivity and fate of HCN and its decomposition products, it is important to consider some prebiotic scenarios, such as the surroundings of submarine and subaerial hydrothermal systems (Colín-García et al., Reference Colín-García, Villafañe-Barajas, Camprubí, Ortega-Gutiérrez, Colás and Negrón-Mendoza2018), and to explore their potential implications for chemical evolution.
Materials and methods
Synthesis of HCN
HCN solution (0.1 M) was produced in situ by the reaction between KCN and H2SO4. HCN gas was dissolved in Milli Q water into an Ar atmosphere (Azamar and Draganić, Reference Azamar and Draganić1982). The concentration was determined by titration with an aqueous solution of AgNO3 (Draganić et al., Reference Draganić, Draganić, Petković and Nikolić1973).
Preparation of Mg2+-montmorillonite
Mg-montmorillonite (Mg-Mont) was prepared from SWy-2cNa-Mont Crook County, Wyoming, USA (Na-Mont), according to the following procedure: 40 g of Na-Mont was washed with a saturated solution of MgCl2.6H2O (2 N) for 24 h. The suspension was then centrifuged for 30 min at 20 000 rpm and washed with deionized water by shaking for 24 h. The suspension was centrifuged and lyophilized. This procedure was repeated three times. The dry powder sample was analysed by X-ray diffraction to quantify the differences of the interlamellar space of the clay. It was corroborated that Mg2+ had completely replaced Na+. Mg-Mont was used as magnesium was a common element on early Earth and because it is present in a wide variety of minerals in hydrothermal systems (Holm, Reference Holm2012; Colín-García et al., Reference Colín-García, Heredia, Cordero, Camprubí, Negrón-Mendoza, Ortega-Gutiérrez, Beraldi and Ramos-Bernal2016, Reference Colín-García, Villafañe-Barajas, Camprubí, Ortega-Gutiérrez, Colás and Negrón-Mendoza2018).
Thermolysis
Thermolysis experiments were carried out in a static system at ≈100°C (Fig. 1). Aliquots of the HCN solution (0.1 M, 5 ml) were placed in glass tubes of 8 ml with stopcocks; the headspace was air. A ball flask with wells was filled up with toluene. Toluene was heated until boiling; samples were heated for different periods of time by conduction. All tests were carried out in triplicate. Controls and experiments including Mg-Mont were performed with 100 mg Mg-Mont by 5 ml HCN (0.1 M) at acidic and basic pH. The pH was modified and adjusted to 2 or 8.5, adding drops of HCl or KOH solution (0.1 M), respectively. The greater time exposition to heat for each sample was HCNacid: 141.5 h, HCN/Montacid: 160 h, HCNbasic: 143 h and HCN/Montbasic: 159.5 h. The aqueous phase of the samples was analysed to quantify changes of the organic molecule.
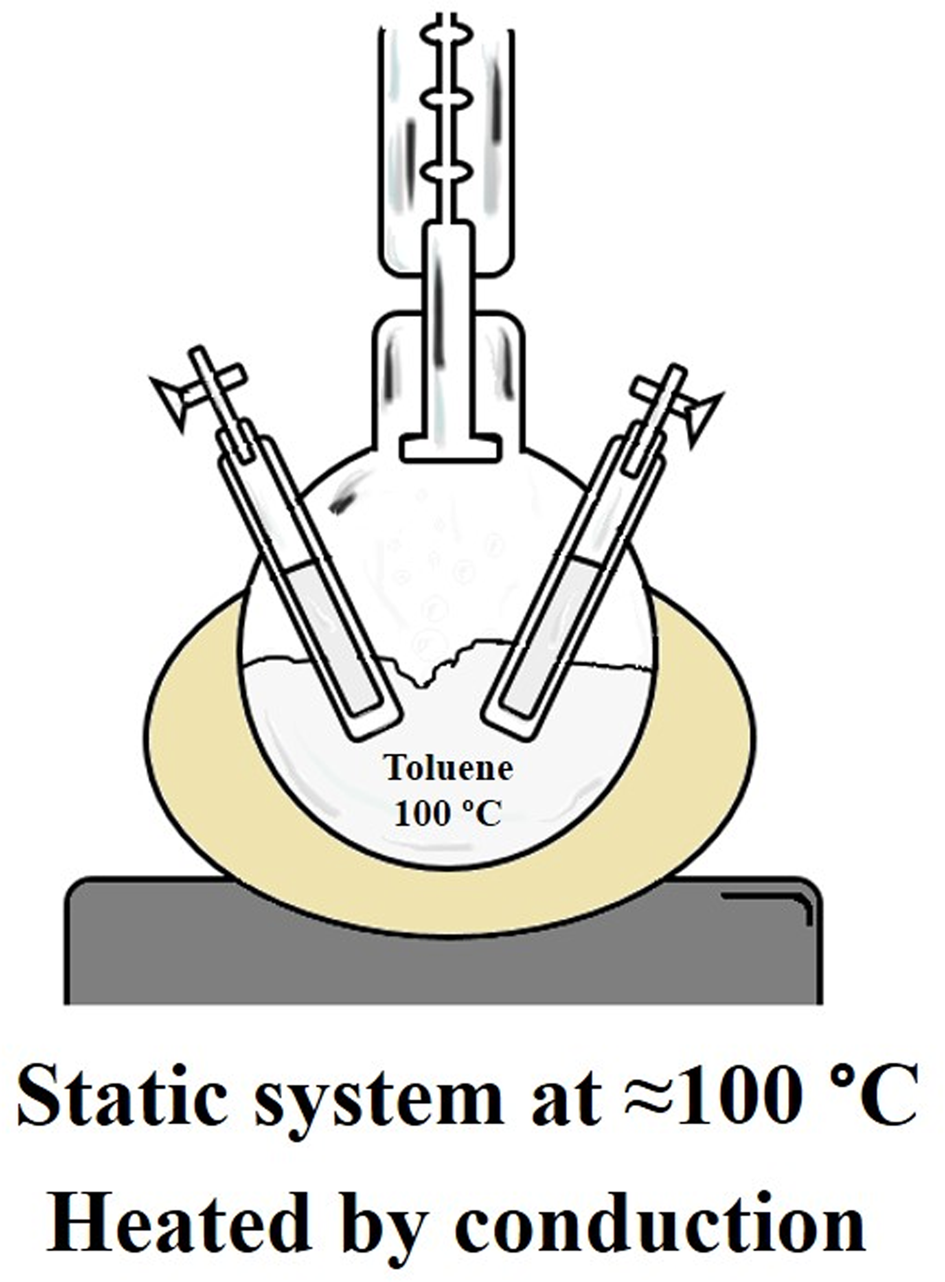
Fig. 1. Static heating system. A ball flask with wells is filled up with toluene; the system is heated until boiling, and samples are heated by conduction.
Analysis of samples
The treated samples were analysed by different analytical techniques:
Gas chromatography (GC)
The remnant HCN was followed by GC (Varian Series 2400 with flame ionization detector) on a stainless-steel column (1/8 inch and 4 m length) packed with Chromosorb-102 (100/120 mesh). The carrier gas was N2 with a flow rate of 30 ml min−1. The temperature regime was 60–250°C, with a heating rate of 6°C min−1. The detector temperature was 200°C. A volume of 2 μl was injected.
GC-MS
The GC-MS analysis was done in a 6859 network GC system coupled to a 5975 VL MSD with triple-axis detector operating in electron-impact mode at 70 eV (Agilent). HP-5 MS columns (30 m × 0.25 mm i.d. × 0.25 μm film thickness) were used; the analysis was carried out using the method developed by Ruiz-Bermejo et al. (Reference Ruiz-Bermejo, de la Fuente, Rogero, Menor-Salván, Osuna-Esteban and Martín-Gago2012) to detect polar organic compounds. After thermolysis, samples were freeze-dried to remove H2O. Then, 100 μl of BSTFA + TMCS [N,O bis(trimethylsilyl)trifluoroacetamide with Me3SiCL, Thermo Scientific Co, Waltham, MA, USA] was added to dry material and heated for 3 h at 80°C. Next, 2 μl of derivatized sample was injected (injector temperature 220°C, detector temperature 300°C and flow rate 1.1 ml min−1; He as a carrier gas).
Ultra-high performance liquid chromatography (UHPLC)
The aqueous component was analysed by UHPLC (UltiMate 3000 UHPLC chromatograph, Thermo Scientific Co) with a UV-Vis detector (Dionex UltiMate 3000 VWD). For carboxylic acids, we used an Alltech anion exclusion column (7.8 × 300 mm) and 1.5 mmol−1 of H2SO4 as a mobile phase, with a flow rate of 0.6 ml min−1. The detection wavelength (λ) was λ = 210 nm. For diaminomaleonitrile (DAMN) detection, a Waters Cortex 2.7 μm, 4.6 × 150 mm column was used; the mobile phase was (A) ammonium acetate 0.1 mol l−1 and (B) methanol:water 50:50, with a 90 A:10 B ratio and a flow rate of 0.5 ml min−1. The detection wavelength was λ = 293 nm.
UV-Vis spectroscopy
Urea was identified using the DAM method. The detection wavelength was λ = 526 nm (Negrón-Mendoza et al., Reference Negrón-Mendoza, Chacón, Perezgasga and Torres1986).
Results and discussion
Thermolysis
Figure 2 shows the thermolysis of HCN at acidic (2) and basic (8.5) pH, as well as in the presence of Mg-Mont. According to the species distribution diagram of HCN (Fig. 3), at pH = 2, HCN is the dominant species. However, at pH = 8.5, there is a considerable amount of cyanide (~20%). The availability of cyanide ions is crucial because, as we will discuss below, it is directly related to the polymerization process.

Fig. 2. Thermolysis of HCN at 100°C. Under acid conditions (pH = 2) the HCN remains relatively stable, but in the presence of Mg-Mont, the decomposition increases. At basic pH values (pH = 8.5) and in the absence of clay, the transformation of HCN is more efficient.

Fig. 3. Species distribution diagram for HCN.
It is possible to identify some trends about the behaviour of HCN in both acidic and basic conditions (Fig. 2). It is noticeable that in acidic pH, the decomposition of HCN is low, but the presence of Mg-Mont increases its decomposition. Some experiments have shown that the half-life time for the hydrolysis of HCN is only a few minutes (Sanchez et al., Reference Sanchez, Ferris and Orgel1968; Stribling and Miller, Reference Stribling and Miller1987; Miller and Bada, Reference Miller and Bada1988). However, Schäfer and Bonn (Reference Schäfer and Bonn2000) demonstrated that hydrolysis of HCN(g), as a function of temperature and in the presence of H2O and N2, is relatively slow until 700°C. It is well understood that hydrolysis of HCN yields formamide and, eventually, formic acid (Sanchez et al., Reference Sanchez, Ferris and Orgel1967; Ferris et al., Reference Ferris, Donner and Lobo1973; Schwartz et al., Reference Schwartz, Voet and Veen1984; Miyakawa et al., Reference Miyakawa, Cleaves and Miller2002; Borquez et al., Reference Borquez, Cleaves, Lazcano and Miller2005).
Some experiments have used inorganic surfaces to investigate the hydrolysis of HCN and suggested that this could be the result of the participation of acid sites at catalysts (Nanba et al., Reference Nanba, Obuchi, Akaratiwa, Liu, Uchisawa and Kushiyama2000; Ma et al., Reference Ma, Wang, Wang, Ning, Jing and Cheng2017). The catalytic activity of clay minerals may result from Brønsted acidity, Lewis acidity, presence of redox-active species and/or introduction of catalytic species along the crystal structure (Adams and McCabe, Reference Adams and McCabe2006). In addition, the acidity at the surface of Mg-Mont may be the result of terminal hydroxyl groups, the bridging of oxygens and exchangeable cations. Likewise, Mg2+ has greater polarizing ability and, in consequence, it can dissociate the water in the interlamellar channel, releasing a proton to the medium (Frenkel, Reference Frenkel1974; Nikalje et al., Reference Nikalje, Phukan and Sudalai2000). In addition, the interaction of HCN with the Brønsted acid sites by hydrogen bonding in the lattice of clay would increase the hydrolysis of HCN (Cruz, Reference Cruz1974; Jamis et al., Reference Jamis, Drljaca, Spiccia and Smith1995). Although one experimental report suggested that HCN at acidic conditions may be sorbed onto montmorillonite (Colín-García et al., Reference Colín-García, Heredia, Negron-Mendoza, Ortega, Pi and Ramos-Bernal2014), the HCN:Mont relationship in our experiments suggests that hydrolysis is the predominant mechanism, rather than sorption.
At basic pH, the decomposition of HCN is lower in the presence of clay. Although HCN hydrolysis at basic pH is possible, it has been demonstrated that polymerization until tetramer, DAMN, is the dominant mechanism (Miyakawa et al., Reference Miyakawa, Cleaves and Miller2002; Borquez et al., Reference Borquez, Cleaves, Lazcano and Miller2005).
Ferris et al. (Reference Ferris, Edelson, Mount and Sullivan1979) showed that in the presence of montmorillonite, the oligomerization of HCN is inhibited, suggesting an interaction with HCN oligomers, and not with HCN itself. In other words, they found that clay catalysed the decomposition of tetramer, DAMN, to form diiminosuccinonitrile (DISN); consequently, the degradation of DAMN involves the release of HCN to the medium. According to this hypothesis, our results are congruent with the greater concentration of free HCN in the presence of clay (Fig. 2). The decomposition of tetramer could be the result of oxidation reactions, mediated by the hydrolysis process and/or the presence of Fe3+ in the clay lattice (Ferris et al., Reference Ferris, Hagan, Alwis and McCrea1982).
In summary, the results suggest that the hydrolysis process, at acidic pH and at 100°C, is the dominant mechanism of transformation of HCN. However, at basic pH and at 100°C, the polymerization of HCN is the dominant reaction (Fig. 2). In addition, the change in colour (yellow to brown) of basic samples, after treatment, is evidence of the polymerization process. The presence of Mg-Mont has two effects: on the one hand, it increases the hydrolysis of HCN (at acidic pH) and, on the other, it inhibits the oligomerization of HCN at basic pH. In order to evaluate the effect of pH on HCN-thermal products, several analytic studies were performed to identify organic molecules in the aqueous component.
Products of HCN thermolysis
Some intermediate species with great prebiotic importance can be formed from HCN under several experimental conditions (Ruiz-Bermejo et al., Reference Ruiz-Bermejo, Zorzano and Osuna-Esteban2013). In this work, only the aqueous phase from HCN thermolysis, the supernatant, was analysed. The organic richness of this aqueous phase will determine the best geochemical conditions in which the products of HCN thermolysis can undergo subsequent reactions in a dynamic environment. The nature, availability and reactivity of the organic molecules dissolved in the medium will determine the importance of HCN on hydrothermal environments. The supernatants of each experiment, with the greater time exposition to heat, were analysed by different qualitative methods.
Aldehydes
Only in the acidic sample heated without clay, a small peak associated with formaldehyde (HCHO) was detected by GC, both by retention time and co-injection of the standard (Fig. 4). Although the decomposition of formic acid – formed by hydrolysis of HCN – under hydrothermal conditions has as major products CO2 and H2 (Yu and Savage, Reference Yu and Savage1998), it has been suggested that formaldehyde can also be a reaction product (Nelson and Engelder, Reference Nelson and Engelder1925). In addition, the production of formaldehyde from the radiolysis of HCN has been reported (Negrón-Mendoza et al., Reference Negrón-Mendoza, Draganić, Navarro-González and Draganić1983). Even if derivatization by Brady's method (in which carbonyl groups are identified by the formation of 2,4-dinitrophenylhydrazones) (Shriner et al., Reference Shriner, Fuson, Reynold, Curtin, David and Dominguez1982) was performed for all samples, none of them showed positive results; this may be associated with a very low concentration of produced aldehydes in the samples.

Fig. 4. Gas chromatogram of volatile products from the sample of HCN thermolysis at acidic pH without Mg-Mont. The formation of formaldehyde (HCHO) is shown.
Urea
Several researches have shown that urea is a product of HCN oligomerization. Specifically, it may be a product of oxidation/hydrolysis of DAMN (Ferris et al., Reference Ferris, Donner and Lobo1973, Reference Ferris, Wos, Ryan, Lobo and Donner1974b; Ferris and Ryan, Reference Ferris and Ryan1973; Ferris and Edelson, Reference Ferris and Edelson1978; Ferris and Hagan, Reference Ferris and Hagan1984). Our experiments showed that urea is formed only by thermolysis of slightly basic HCN solutions (pH = 8.5) and even in the presence of Mg-Mont (Fig. 5). In addition, urea concentration was greater in the sample without clay. This is consistent with the HCN polymerization mechanism previously mentioned. In other words, and in agreement with Fig. 2, HCN polymerizes better in the absence of clay mineral; and, in consequence, the formation of the tetramer should be higher. Hence, the oxidation/hydrolysis reaction of DAMN at high temperatures led to the formation of an important amount of free urea (≈40% higher concentration than the sample with mineral).

Fig. 5. Urea is a product of the thermolysis of HCN under slightly basic solutions. The spectrum UV-Vis shows the specific absorption band of the complex formed by the reaction of DAM.
Urea has been considered an important prebiotic molecule due to its participation in synthesis reactions of pyrimidines (Robertson and Miller, Reference Robertson and Miller1995; Menor-Salván et al., Reference Menor-Salván, Ruiz-Bermejo, Guzmán, Osuna-Esteban and Veintemillas-Verdaguer2009), and its role in hydrothermal scenarios was highlighted (Holm, Reference Holm1992). Likewise, it has also been found in HCN-radiolysis experiments (Draganić et al., Reference Draganić, Draganić, Azamar, Vujošević, Berber and Negrón-Mendoza1985a, Reference Draganić, Vujošević, Negrón-Mendoza, Azamar and Draganićb; Vujošević et al., Reference Vujošević, Negrón-Mendoza and Draganić1990; Colín-García et al., Reference Colín-García, Negrón-Mendoza and Ramos-Bernal2009).
Carboxylic acids
The formation of some carboxylic acids by thermolysis of HCN was confirmed (Fig. 6). Among the main carboxylic acids identified are: oxalic, maleic, glycolic and formic acid, which were also identified by GC-MS. The formation of formic acid corroborates that, at pH = 2, hydrolysis is the main reaction. In addition, the peaks of oxalic and maleic acid may be a result of the decomposition of formic acid along thermolysis; in other words, they are secondary products.

Fig. 6. UHPLC chromatograms of carboxylic acids formed by HCN-thermolysis. Under basic conditions, the formation of carboxylic acids is higher than at acidic pH. Legend: (1) oxalic acid, (2) maleic acid, (3) not characterized, (4) glycolic acid, (5) formic acid, (6) not characterized and (7) HCN.
The samples under basic pH show more production of carboxylic acids than in acidic conditions. The main reaction involves the formation of oxalic acid, which could be the result of oxidation of DAMN and the reaction between DISN with water and other nucleophiles (Ferris et al., Reference Ferris, Hagan, Alwis and McCrea1982; Schwartz et al., Reference Schwartz, Voet and Veen1984). Additionally, the presence of Mg-Mont seems to affect the reaction products (a predominant unidentified peak, with R t~8.5 min, was detected on the sample without mineral). Moreover, the sample with clay showed a higher remnant of HCN, which is consistent with Fig. 2. Clay mineral inhibits the polymerization process, and hence, a higher amount of free HCN is available. Negrón-Mendoza et al. (Reference Negrón-Mendoza, Ramos-Bernal, Cruz and Juárez2001) reported that the presence of Na-Mont in HCN radiolysis significantly affects the amount and diversity of carboxylic acids formed.
Other options to explain the formation of carboxylic acids may be by reactions between aldehydes (e.g. formaldehyde) and the cyanide ion through the formation of cyanohydrins as intermediates; as well as the formation of other nitriles (e.g. cyanate, cyanogen, cyanoacetylene, glyconitrile), which are produced from decomposition (i.e. hydrolysis, oxidation) of HCN (Kemp and Kohnstam, Reference Kemp and Kohnstam1956; Brotherton and Lynn, Reference Brotherton and Lynn1959; Ferris et al., Reference Ferris, Sanchez and Orgel1968; Wang et al., Reference Wang, Lee, Beach and Margerum1987).
Diaminomaleonitrile
Among oligomers of HCN, DAMN has drawn great attention because it has been pointed out as a gateway for the synthesis of purine and pyrimidines (Zubay, Reference Zubay2000). The analysis of the aqueous phase of heated samples shows that DAMN is present only in the basic solutions (Fig. 7), as previously mentioned (Miyakawa et al., Reference Miyakawa, Cleaves and Miller2002; Borquez et al., Reference Borquez, Cleaves, Lazcano and Miller2005). Even though Yuasa and Ishigami (Reference Yuasa and Ishigami1977) showed that DAMN is also formed in the presence of Mg oxides, which suggests that divalent metals would favour the HCN oligomerization, the presence of a clay mineral seems to inhibit the synthesis of DAMN (the sample with clay showed a considerably lower amount of this oligomer). As mentioned above, the decomposition of DAMN may occur by its hydrolysis and/or oxidation by Fe3+ in the clay lattice (Begland et al., Reference Begland, Hartter, Jones, Sam, Sheppard, Webster and Weigert1974; Ferris et al., Reference Ferris, Hagan, Alwis and McCrea1982). Another possibility is that the lower amount of free DAMN in the sample with Mg-Mont may be the result of surface retention of this oligomer, as this has been reported to happen with other surfaces (Thissen et al., Reference Thissen, Koegler, Salwiczek, Easton, Qu, Lithgow and Evans2015; Toh et al., Reference Toh, Evans, Thissen, Voelcker, d'Ischia and Ball2019). This is an important result because, although the HCN thermolysis under alkaline conditions yields DAMN, eventually, its interaction with the available clays in the medium would constrain the oligomerization process.

Fig. 7. UHPLC chromatogram of HCN thermolysis samples. Only in basic medium did the HCN thermolysis yield DAMN. However, Mg-Mont may inhibit the polymerization of HCN.
CG-MS analysis
To corroborate some of the species that we characterized and some free amino acids, a derivatization of samples, without the common hydrolysis procedure, was performed. Hydrolysis can affect the original amount and nature of the soluble fraction, and this procedure is focused on HCN-derived polymers (Ruiz-Bermejo et al., Reference Ruiz-Bermejo, Zorzano and Osuna-Esteban2013). As we mentioned above, this study is only focused on the soluble fraction of samples.
Glycine and alanine traces were detected only in the acidic sample without clay by GC-MS (data not shown). In both basic samples, it was also possible to corroborate the presence of urea, oxalic and glycolic acid by GC-MS. In addition, intermediate species such as carbamic acid, ethanolamine, glycerol, succinic and propanoic acid were detected in basic solution fractions. It should be mentioned that, in general, free amino acids are not detected in our HCN experiments. Only after acid hydrolysis of HCN oligomers, synthesized either by thermal energy or ionizing radiation, are a significant amount of amino acids released (Ferris et al., Reference Ferris, Wos, Nooner and Oró1974a; Draganić et al., Reference Draganić, Draganić, Azamar, Vujošević, Berber and Negrón-Mendoza1985a, Reference Draganić, Vujošević, Negrón-Mendoza, Azamar and Draganićb; Vujošević et al., Reference Vujošević, Negrón-Mendoza and Draganić1990; Ruiz-Bermejo et al., Reference Ruiz-Bermejo, Zorzano and Osuna-Esteban2013; Marín-Yaseli et al., Reference Marín-Yaseli, González-Toril, Mompeán and Ruiz-Bermejo2016). The formation of free amino acids can be explained by common Strecker synthesis among HCN, aldehydes and ammonia. Some authors have reported the viability of this mechanism under submarine conditions (Schulte and Shock, Reference Schulte and Shock1995; Andersson and Holm, Reference Andersson and Holm2000). Other pathways for amino acid production [like the synthesis from ethanolamine (detected in this study by GC-MS) using metal powder and Friedel-Crafts reactions catalysed by minerals] have been proposed under alkaline hydrothermal scenarios (Zhang et al., Reference Zhang, Tian, Gao, Han, Su, Wang and Feng2017; Ménez et al., Reference Ménez, Pisapia, Andreani, Jamme, Vanbellingen, Brunelle, Richard, Dumas and Réfrégiers2018).
In summary, the thermolysis of acidic samples shows that the formation of formic acid by hydrolysis of HCN is the predominant mechanism. In addition, the presence of Mg-Mont increases the transformation of HCN. The formation of formaldehyde and small carboxylic acids, in acidic conditions, may well be the result of decomposition of formic acid. Differently, the thermolysis at basic conditions showed a higher formation of organic molecules. In the case of the sample without clay, the amount of recovered HCN was lower with the consequent high formation of DAMN. Likewise, semi-quantitative analysis showed that a high amount of urea and oxalic acid was present. Finally, the higher amount of free HCN and the lower amount of urea and DAMN in the sample with Mg-Mont corroborate that clay inhibits the oligomerization of HCN at basic conditions.
Relevance for chemical evolution
Our experiments showed that, even considering a simple hydrothermal simulation, HCN thermolysis may be an important starting point for the production of several organic molecules. If the synthesis of HCN under hydrothermal conditions is continuous, these systems could have had a considerable role as ‘abiotic reactors and chemical evolution niches’, as they were originally proposed (Corliss et al., Reference Corliss, Baross and Hoffman1980; Baross and Hoffman, Reference Baross and Hoffman1985). It is important to highlight that geochemical conditions of the environment are crucial because they are directly related to the formation/destruction mechanisms of more complex organic compounds. Although some experiments suggest that both HCN and some thermolysis products are unstable under hydrothermal conditions (Sanchez et al., Reference Sanchez, Ferris and Orgel1968; Stribling and Miller, Reference Stribling and Miller1987; Miller and Bada, Reference Miller and Bada1988; Holm, Reference Holm1992; Yu and Savage, Reference Yu and Savage1998; Cleaves II, Reference Cleaves2008), it is necessary to take into account several points that, according to our criteria, could be underestimated.
The first is that hydrothermal systems are very complex environments with many geochemical variables, and hence, they are more than high-pressure and high-temperature environments. In this way, new approaches are considering the role of several combined parameters (i.e. pH, redox state, dissolved gases and/or mineral surfaces) on the stability of compounds. In general, these new approaches have shown that the fate and stability of organic molecules are intrinsically related to these variables (Holm and Andersson, Reference Holm and Andersson2005; Colín-García et al., Reference Colín-García, Heredia, Cordero, Camprubí, Negrón-Mendoza, Ortega-Gutiérrez, Beraldi and Ramos-Bernal2016, Reference Colín-García, Villafañe-Barajas, Camprubí, Ortega-Gutiérrez, Colás and Negrón-Mendoza2018).
Additionally, the chemical species are not isolated. In other words, the availability of different species in the same environment could favour the subsequent reactions. For instance, the presence of aldehydes and ammonia would favour the polymerization of HCN (Voet and Schwartz, Reference Voet and Schwartz1983).
Additionally, the molecules are not exposed to the highest temperature all the time. At the moment that the hydrothermal fluids are mixed with seawater, new temperature and/or pH gradients can harbour more suitable conditions (Chevaldonné et al., Reference Chevaldonné, Desbruyères and Haître1991; Bates et al., Reference Bates, Lee, Tunnicliffe and Lamare2010) and favour the stability and polymerization of organic molecules (Ogasawara et al., Reference Ogasawara, Yoshida, Imai, Honda, Hatori and Matsuno2000; Ogata et al., Reference Ogata, Imai, Honda, Hatori and Matsuno2000; Islam et al., Reference Islam, Kaneko and Kobayashi2003).
This approximation about the role of the HCN thermolysis process in the formation of organic molecules suggests that surroundings of hydrothermal environments (<100°C), such as sub-aerial alkaline environments, could have been an import source of free organic compounds on early Earth. It is necessary to design and carry out more complex experiments that would consider coupled variables present in hydrothermal systems to gain a detailed understanding of the behaviour of organic molecules under several geochemical conditions.
Acknowledgements
SAVB acknowledges Posgrado en Ciencias de la Tierra UNAM, CONACyT (Ph.D. grant 697442 and the financial support for a research stay grant) and the Laboratorio de Evolucion Quimica (ICN-UNAM) for the use of its facilities. ANM acknowledges PAPIIT (IN110919). M.R-B. used the research facilities of the Centro de Astrobiología (CAB), and was supported by the Instituto Nacional de Técnica Aeroespacial ‘Esteban Terradas' (INTA) and by the Spanish Ministerio de Ciencia, Innovación y Universidades ESP2017-89053-C2-2-P. M.C-G acknowledges the financial support from the CONACyT A1-S-24341 and DGAPA-PAPIIT IA203217 research grants, and from the Instituto de Geología (UNAM). The authors are indebted to Claudia Camargo, Jorge A. Cruz Castañeda and Adriana Meléndez-López for their technical assistance.