1. Introduction
Ordovician sedimentary sequences from the Precordilleran basin of western Argentina were penetrated by a series of mafic sills, of several metres thickness, that intruded the sediments early after deposition. At Ancaucha creek, the Yerba Loca Formation exposes pillow lavas, numerous basic intrusive bodies, and the effects of a regional metamorphism that locally reaches greenschist facies (Fig. 1). The igneous rocks are genetically related and represent the uppermost section of an ophiolite belt that crops out discontinuously along the western slope facies of the Precordillera carbonate platform (Haller & Ramos, Reference Haller, Ramos and Editorial1984; Kay, Ramos & Kay, Reference Kay, Ramos, Kay and Editorial1984; Ramos et al. Reference Ramos, Jordan, Allmendinger, Mpodozis, Kay, Cortés and Palma1986, Reference Rejebian, Harris and Huebner2000). In the Western Precordillera, the main deformational event is coeval with a low-grade regional metamorphism that took place in Siluro-Devonian times (Gosen, Reference Gosen1992; Buggisch et al. Reference Buggisch, Gosen, Henjes–Kunst and Krumm1994; Davis et al. Reference Davis, Roeske, McClelland, Snee, Ramos and Keppie1999).

Figure 1. Location and geological map of Ancaucha creek (modified after Albanesi, Ortega & Hünicken, Reference Ortega, Albanesi and Frigerio1995).
Conodont-rich mixed clastic–calcareous turbidites are common in Ancaucha creek and allow for high-resolution biostratigraphy through the sedimentary section. The conodont Colour Alteration Index (CAI) has been used, together with X-ray diffraction analyses of clay minerals as well as petrography, in order to assess the effects of rapid thermal stress produced by basic intrusive rocks and subsequent regional low-grade metamorphism. The mafic sills from Ancaucha creek reach tens of metres in thickness and allow testing of the conodont CAI response to extended thermal aureoles. Previous studies referred to CAI and contact metamorphism constrained to smaller intrusions (e.g. Nicoll, Reference Nicoll1981; Armstrong & Strens, Reference Armstrong, Strens and Austin1987; Burnett, Reference Burnett1988). A theoretical thermal model of the intrusions is contrasted with empirical geological evidence to evaluate the maturity parameters.
2. Geological setting
The Precordillera geological province comprises N–S-trending chains from the external zone of the Andean orogen, between 29 and 33° S (Ramos, Reference Ramos, Clark and Burchfiel1988). It represents a fold-and-thrust belt mostly composed of Cambrian to Carboniferous strata triggered by flat-slab subduction of the Nazca plate in Neogene times (Jordan et al. Reference Jordan, Allmendinger, Damanti and Drake1993; Ramos, Cristallini & Pérez, Reference Ramos, Cristallini and Pérez2002). The Yerba Loca Formation (Furque, Reference Furque1963), Darriwilian–Sandbian in age, crops out in the northern and central parts of the Precordillera terrane, between 29°15′ and 31°30′ S, at the Yerba Loca, La Tranca and El Tigre ranges. This stratigraphic unit is characterized by clastic rocks (sandstones, claystones, calcarenites and spare conglomerates) and concordant to sub-concordant mafic to ultramafic bodies. Due to its complex deformational style, it is difficult to estimate the true thickness (Ramos et al. Reference Ramos, Jordan, Allmendinger, Kay, Cortes, Palma and Editorial1984, Reference Ramos, Jordan, Allmendinger, Mpodozis, Kay, Cortés and Palma1986; Gosen, Reference Gosen1997). However, Furque (Reference Furque1983) suggested a minimum thickness of 1500 m, based on integrated stratigraphic profiles. Neodymium isotopic data from the Yerba Loca Formation suggest an upper crustal and a less evolved source for the sediments (Abre et al. Reference Abre, Zimmermann, Cingolani, Cairncross, Chemale, Bossi, Gaucher and Campal2006), broadly similar to the eastern Precordilleran basins (Gleason et al. Reference Gleason, Finney, Peralta, Gehrels and Marsaglia2007).
Westernmost outcrops of the Yerba Loca Formation (Cuesta del Viento locality) exhibit west-vergent Palaeozoic folds with amplitudes and wavelengths of several hundreds of metres associated with penetrative cleavage planes and sericite growth, which indicates very low-grade metamorphism (Ramos et al. Reference Ramos, Jordan, Allmendinger, Mpodozis, Kay, Cortés and Palma1986; Gosen, Reference Gosen1997). For this section, Kay, Ramos & Kay (Reference Kay, Ramos, Kay and Editorial1984) demonstrated that pillow lavas and columnar jointed basalts are genetically linked to mafic–ultramafic sills that concordantly intrude distal turbidites and pelagic deposits. Folded igneous rocks provide evidence that the low-grade metamorphism and deformational event developed later (Ramos et al. Reference Ramos, Jordan, Allmendinger, Kay, Cortes, Palma and Editorial1984, Reference Ramos, Jordan, Allmendinger, Mpodozis, Kay, Cortés and Palma1986). The emplacement of the mafic bodies had not significantly fractured or folded the sedimentary bedding.
At Cuesta del Viento section (near the study area), the metamorphic character of mafic rocks from the Yerba Loca Formation was analysed by Robinson, Bevins & Rubinstein (Reference Robinson, Bevins and Rubinstein2005), who suggested P–T conditions of about 2–3 kbar and 250–350 °C. These authors identified prehnite, pumpellyite and actinolite, in absence of zeolites and/or laumontite, which points to the higher levels of sub-greenschist facies metamorphism. Whole-rock Sm/Nd isotopic ratios are consistent with a Late Ordovician–early Silurian age for regional deformation and metamorphism (Gleason et al. Reference Gleason, Finney, Peralta, Gehrels and Marsaglia2007). Nevertheless, K/Ar dating and structural relationships of comparable settings from the Western Precordillera suggest a Siluro-Devonian time for the low-grade metamorphism that affected all pre-Carboniferous units (Gosen, Reference Gosen1992; Buggisch et al. Reference Buggisch, Gosen, Henjes–Kunst and Krumm1994; Davis et al. Reference Davis, Roeske, McClelland, Snee, Ramos and Keppie1999). Subsequent Neogene W–E Andean deformation was superposed onto earlier Palaeozoic structures without significantly overprinting them (Gosen, Reference Gosen1997; Alvarez-Marron et al. Reference Alvarez-Marron, Rodriguez-Fernandez, Heredia, Busquets, Colombo and Brown2006).
Gabbroic sills up to 50 m in thickness intruded the Yerba Loca Formation at Ancaucha creek. The mineralogy of those intrusions has been analysed by Fernández-Noia, Sumay & Meissl (Reference Fernández-Noia, Sumay and Meissl1990). Sedimentary strata adjacent to the basic sills have a notable banded appearance and are composed of interlayered distal mixed-turbidites and argillaceous hemipelagites. A similar arrangement was described by Quartino, Zardini & Amos (Reference Quartino, Zardini and Amos1971) in the Alcaparrosa Formation of the Western Precordillera, and we have observed that situation in the Sierra de la Invernada Formation, Central Precordillera, as well.
At Ancaucha creek, the base of the Yerba Loca Formation is apparently concordant with the Los Sombreros Formation (an olistostromic unit) and yielded conodonts from the Lenodus variabilis Zone (early Darriwilian age) (Albanesi, Ortega & Hünicken, Reference Albanesi, Ortega and Hünicken1995). Blasco & Ramos (Reference Blasco and Ramos1976) recovered a graptolite fauna of early Caradoc age from outcrops of the Yerba Loca Formation at the Cuesta del Viento locality. Subsequent graptolite finds made by Ortega, Brussa & Astini (Reference Ortega, Brussa and Astini1991) correspond to specimens assigned to the Paraglossograptus tentaculatus and Nemagraptus gracilis zones, which have extended the temporal range of the stratigraphic unit from early Darriwilian to early Sandbian ages so far. On the other hand, the Los Sombreros Formation (Cuerda, Cingolani & Varela, Reference Cuerda, Cingolani and Varela1983) represents the basal décollement of the Western Allochthon tectonic unit, which includes the Yerba Loca Formation, and was thrusted over the Central Imbricate system prior to the middle Carboniferous sedimentation (Alvarez–Marron et al. Reference Alvarez-Marron, Rodriguez-Fernandez, Heredia, Busquets, Colombo and Brown2006). Immediately beneath this thrust, Devonian siliciclastic sequences of the Talacasto Formation (Furque, Reference Furque1979) appear intensively folded.
The mafic intrusions of the Yerba Loca Formation produced thermal anomalies in the host rock up to CAI 7, prevailing over the regional CAI 4 (sensu Albanesi, Ortega & Hünicken, Reference Albanesi, Ortega and Hünicken1995). Combined effects of swarm intrusions could have enhanced the geothermal gradient (e.g. Nicoll & Gorter, Reference Nicoll and Gorter1984), giving rise to higher conodont CAIs than expected. Other areas not related to this magmatism achieved maximum temperatures during Siluro-Devonian low-grade metamorphism. For example, stratigraphic units that interfinger the Yerba Loca Formation yielded conodonts with CAI 3; this was particularly so in the Sierra de La Invernada Formation to the south. Additionally, conodonts from the Los Sombreros Formation at Ancaucha creek exhibit a CAI 3.
3. Methods of study
Carbonate–clastic turbidite samples for conodont studies were taken from the Yerba Loca Formation at the Puerta de Ancaucha (30°6.33′ S, 68°52.56′ W; samples labelled to as ‘P’) and the Salto (30°5.68′ S, 68°53.62′ W; ‘A’ and ‘B’) sections (Figs 1, 2). Both outcrops contain mafic sills, of 30 and 50 m thickness, respectively.

Figure 2. Outcrops of distal turbidites–hemipelagites and sills from the Yerba Loca Formation at Ancaucha creek. (a) El Salto section. Hammer is 33 cm long. (b) Puerta de Ancaucha section. Person at lower left corner for scale.
3.a. Conodont colour alteration index
Conodonts are apatitic microfossils that contain trace amounts of organic matter within their structure. The variation of colour in conodont elements exposed to heat has long been known (Ellison, Reference Ellison1944), but it was not explained and quantified until the works of Epstein, Epstein & Harris (Reference Epstein, Epstein and Harris1977) and Rejebian, Harris & Huebner (Reference Rejebian, Harris and Huebner1987). When subjected to heat, conodont elements experience progressive and irreversible chemical transformations of the organic matter included in their phosphatic structure. Unaltered conodonts present a pale yellow and a smooth surface (CAI 1). Gradually increasing temperature results in successive carbonization processes of conodont elements that include the colour sequence light to dark brown (CAI 1.5–4) through black (CAI 5). Subsequent colour changes towards grey (CAI 6), white (CAI 7) and finally translucent (CAI 8) are consequences of oxidation of organic matter, release of constitutional water and recrystallization. As with other organic maturity indices that follow Arrhenius reactions, these processes are directly related to temperature and duration of heating. The different CAI stages, which range in temperature from 50 to > 600 °C, were calibrated with field and laboratory experiments (Epstein, Epstein & Harris, Reference Epstein, Epstein and Harris1977; Rejebian, Harris & Huebner, Reference Rejebian, Harris and Huebner1987).
Several studies using CAI data have focused on contact metamorphism, assessing the effects produced by intrusions over conodont preservation and their host rocks. Short-term high-temperature events do not necessarily produce the normal gradational conodont colour alteration sequence. Usually, contact-metamorphosed conodont elements are not deformed or coarsely recrystallized but have a broader range in CAI values, both locally from sample to sample and even within the same sample. Conodont elements recovered from hydrothermally altered rocks may show similar CAI distributions, but they are generally corroded and present a superficial grey patina produced by oxidation of organic matter (Rejebian, Harris & Huebner, Reference Rejebian, Harris and Huebner1987). In order to test the applicability of the CAI method, Armstrong & Strens (Reference Armstrong, Strens and Austin1987) compared theoretical and experimental data on conodonts that were recovered adjacent to a basaltic dyke, obtaining positive results. Based on conodont CAI, Nicoll (Reference Nicoll1981) recognized that the thermal effects produced by a small volcanic plug in the Canning basin extended nearly one metre into the country rock. In a detailed contribution, Burnett (Reference Burnett1988) documented intensely metamorphosed conodont elements from contact-aureoles of three basic intrusions in northern England, and described how the colour, preservation and chemistry of the microfossils were affected. Following a similar methodology, Königshof (Reference Königshof1991) investigated the close correlation between the conodont CAI increase and the proximity to a granitic intrusion in the Harz Mountains. Other studies were based on CAI values to determine the temperatures of ore-bearing hydrothermal fluids and their host rocks (Sangster, Nowlan & McCracken, Reference Sangster, Nowlan and McCracken1994; Repetski & Narkiewicz, Reference Repetski, Narkiewicz, Górecka, Leach and Kozlowski1996; Cunningham et al. Reference Cunningham, Austin, Naeser, Rye, Ballantyne, Stamm and Barker2004).
The thermal history of rocks located in the transitional interval from diagenesis to low-grade metamorphism has been investigated independently through different criteria (e.g. illite crystallinity, conodont CAI, vitrinite reflectance, fluid inclusion homogenization temperatures) or comparing two or more of these parameters (summarized by Merriman & Frey, Reference Merriman, Frey, Frey and Robinson1999). In particular, the XRD-based illite crystallinity technique introduced by Kübler (Reference Kübler and Neuchâtel1967) (Kübler Index, KI; cf. Guggenheim et al. Reference Guggenheim, Bain, Bergaya, Brigatti, Drits, Eberl, Formoso, Galán, Merriman, Peacor, Stanjek and Watanabe2002) is the most common method for determining grades in pelitic rocks affected by diagenesis and low-temperature metamorphism. In order to distinguish grades of diagenesis and incipient metamorphism in Palaeozoic–Mesozoic terrains from northern Hungary, Kovács & Árkai (Reference Kovács, Árkai and Austin1987) compared illite crystallinity, vitrinite reflectance, conodont CAI and carbonate textural characteristics. A general correlation between KI and CAI values determines that CAI range 4–5.5 reflects anchizone boundaries (García-López et al. Reference García-López, Bastida, Aller and Sanz-López2001).
Forty-five conodont samples (66 kg) from unweathered rocks were processed in 10 % acetic acid solution for a maximum of 48 hours, following the standard etching techniques described by Stone (Reference Stone and Austin1987). Fragmentation of sample rocks was reduced to a minimum, in order not to modify the original taphonomic information. CAI values were determined at the margin or lighter parts of the conodont specimens by direct comparison with a standard CAI set provided by Dr Anita Harris (USGS).
Conodont CAI values are ordinal variables; hence, they cannot be averaged (that is, the activation energy necessary to progress from CAI 2 to 3 is not equivalent to the one from CAI 3 to 4; cf. Villa, Reference Villa2001). Accor-dingly, conodont samples with a range in CAI values were assigned with a modal CAI interval (e.g. CAI 5–6). In contact metamorphic settings, the higher parts of the temperature range of each CAI value reflect the thermal aureole peak (Rejebian, Harris & Huebner, Reference Rejebian, Harris and Huebner1987). Maximum CAI temperatures were adopted after Epstein, Epstein & Harris (Reference Epstein, Epstein and Harris1977) and Rejebian, Harris & Huebner (Reference Rejebian, Harris and Huebner1987), considering that the Ordovician intrusions produced a short-term heating event (Fig. 3).
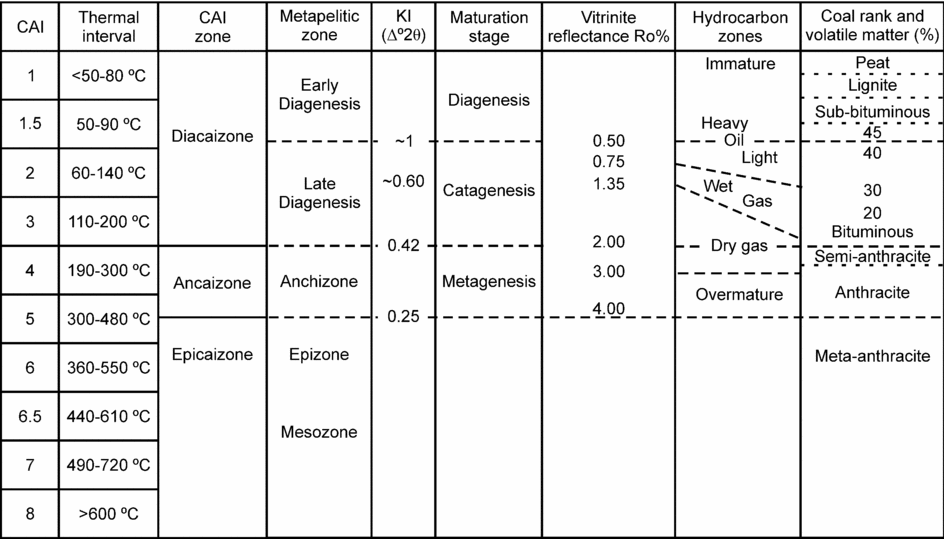
Figure 3. Conodont CAI values contrasted with other low-grade metamorphic indices. Modified after Merriman & Frey (Reference Merriman, Frey, Frey and Robinson1999). Temperature ranges adopted from Epstein, Epstein & Harris (Reference Epstein, Epstein and Harris1977) and Rejebian, Harris & Huebner (Reference Rejebian, Harris and Huebner1987). CAI zone values after García-López et al. (Reference García-López, Bastida, Aller and Sanz-López2001).
3.b. X-ray diffraction analysis and rock petrography
To complement the CAI method, the insoluble residue released after processing conodont samples was recovered for X-ray diffraction (XRD) analysis. In these samples, the clay mineralogy was studied by XRD using oriented mounts by employing a Philips PW1050 diffractometer (INGEIS, Buenos Aires) with CuKα radiation operated at 50 mA and 30 kV. The < 2 μm size-fraction separated by centrifugation was scanned after air-drying, saturating with ethylene glycol (EG) and heating at 500 °C. The Kübler index was determined following the recommendations of Kisch (Reference Kisch1991). Our data were converted to the CIS scale (Warr & Rice, Reference Warr and Rice1994) using the formula: y = 1.15x − 0.01 (R2 = 0.989). Anchizone limits in the CIS scale are 0.42°/0.25° Δ°2θ. Carbonate petrography was analysed as well. Rock slides were observed under the binocular microscope, and sample textures classified following Kovács & Árkai's (Reference Kovács, Árkai and Austin1987) criteria. In accordance with these authors, increasing deformation produces type A textures: without evidence of changes in the original texture; type B texture: weak preferred orientation and incipient foliation in the matrix; and type C texture: when most of the allochemical components are indistinguishable from the recrystallized matrix. Ca and Mg-carbonate mineralogy was differentiated through alizarin-red staining.
3.c. Modelling cooling of the sills and heating of the host rocks by computer: the program HEAT
In order to test the effects of the Puerta de Ancaucha and El Salto basic bodies on the host rock, we have numerically simulated spatial variations of the thermal aureole with time. The influence of the cooling magma on the intruded sediments was estimated with the HEAT program (v.4.10.0526, http://www.ees1.lanl.gov/Wohletz/Heat.htm), originally written by Wohletz & Heiken (Reference Wohletz and Heiken1992) to model transient thermal regimes in and around magmatic intrusions. An improved code, described in detail by Wohletz, Civetta & Giovanni (Reference Wohletz, Civetta and Giovanni1999), solves heat flow by finite difference solution of energy and momentum conservation equations. Numerical and theoretical bases for solution of the heat flow equation can be consulted in Turcotte & Schubert (Reference Turcotte and Schubert2002).
4. Results
4.a. Conodont biostratigraphy
The conodont collection of the Yerba Loca Formation at Ancaucha creek has been recovered from samples of two sections, Puerta de Ancaucha and El Salto. The 45 calcarenite samples for conodonts yielded about 1300 conodont elements, which are housed in the Museo de Paleontología, Universidad Nacional de Córdoba, under repository code CORD-MP.
The taxonomy of determined species is well known, following descriptions of previous authors, and there is no need for further comments in the present contribution (Fig. 4). Overlapping ranges of these species as recorded in the studied sections indicate the Paroistodus horridus Subzone of the Lenodus variabilis Zone in the biostratigraphic scheme developed at Cerro Potrerillo area for the Argentine Precordillera (Albanesi, Hünicken & Barnes, Reference Albanesi, Hünicken and Barnes1998). The L. variabilis Zone represents the middle Darriwilian age and correlates with the graptolite zones Hustedograptus lentus and Nicholsonograptus fasciculatus of same region (Webby et al. Reference Webby, Cooper, Bergström, Paris, Webby, Paris, Droser and Percival2004). The upper part of the L. variabilis Zone (the Paroistodus horridus Subzone), as previously recognized in the Argentine Precordillera, was correlated with conodont and graptolite zones from reference schemes of diverse regions by Ortega, Albanesi & Frigerio (Reference Ortega, Albanesi and Frigerio2007).

Figure 4. Conodont elements from the Lenodus variabilis Zone (Paroistodus horridus Subzone). (a) Paroistodus horridus (Barnes & Poplawski), M element, lateral view, sample A12, ×50, CORD-MP 11238, CAI 5.5. (b) Periodon macrodentata (Graves & Ellison), Pa element, lateral view, sample P10, ×55, CORD-MP 11239, CAI 5.5. (c) Drepanodus reclinatus (Lindström), S element, lateral view, sample A12, ×22, CORD-MP 11240, CAI 5. (d) Dzikodus tablepointensis (Stouge), P element, upper view, sample P12, ×60, CORD-MP 11241, CAI 5. (e) Protopanderodus gradatus Serpagli, S element, lateral view, sample A9, ×36, CORD-MP 11242, CAI 5. (f) Coelocerodontus bicostatus van Wamel, lateral view, sample P10, ×65, CORD-MP 11243. (g) Costiconus costatus (Dzik), S element, lateral view, sample P10, ×70, CORD-MP 11244, CAI 6. (h) Baltoniodus clavatus Stouge & Bagnoli, Pb element, lower view, sample P12, ×25, CORD-MP 11246, CAI 5.5. (i) Drepanoistodus forceps (Lindström), M element, lateral view, sample P10, ×80, CORD-MP 11247, CAI 6. (j) Lenodus variabilis (Sergeeva), Pa element, upper view, sample P10, ×95, CORD-MP 11248, CAI 5. (k) Dzikodus tablepointensis (Stouge), Pa element, upper view, sample P10, ×65, CORD-MP 11256, CAI 5.5. (l) Parapanderodus elegans Stouge, S element, lateral view, sample A12, ×80, CORD-MP 11254, CAI 6. (m) Baltoniodus clavatus Stouge & Bagnoli, M element, lateral view, sample P10, ×60, CORD-MP 11249, CAI 6. (n) Spinodus spinatus (Hadding), S element, lateral view, sample P10, ×45, CORD-MP 11245, CAI 5.5. (o) Periodon macrodentata (Graves & Ellison), Pb element, lateral view, sample P9, ×48, CORD-MP 11252, CAI 6. (p) Protopanderodus gradatus Serpagli, S element, lateral view, sample P12, ×33, CORD-MP 11253, CAI 5. (q) Coelocerodontus trigonius Ethington, lateral view, sample P12, ×33, CORD-MP 11251. (r) Paraprioniodus costatus (Mound), S element, lateral view, sample P4, ×65, CORD-MP 11255, CAI 6. (s) Periodon macrodentata (Graves & Ellison), Pa element, lateral view, sample A12, ×85, CORD-MP 11250, CAI 6. (t) Juanognathus serpaglii Stouge, S element, anterior view, sample P10, ×72, CORD-MP 11258, CAI 6. (u) Periodon macrodentata (Graves & Ellison), M element, lateral view, sample P3, ×56, CORD-MP 11259, CAI 6.5. (v) Polonodus magnum Albanesi, Pa element (juvenile), upper view, sample P12, ×40, CORD-MP 11354, CAI 6.5. (w) Dzikodus tablepointensis (Stouge), Pb element, upper view, sample P4, ×45, CORD-MP 11260, CAI 6.5. (x) Protopanderodus gradatus Serpagli, M element, lateral view, sample A3, ×60, CORD-MP 11257, CAI 6.5. (y) Lenodus variabilis (Sergeeva), Pa element, upper view, sample POCC, ×100, CORD-MP 11262, CAI 7. (z) Parapaltodus simplicissimus Stouge, S element, lateral view, sample POCC, ×100, CORD-MP 11263, CAI 7.5. (aa) Semiacontiodus potrerillensis Albanesi, S element, lateral view, sample A12, ×62, CORD-MP 11355, CAI 6.5. (ab) Spinodus spinatus (Hadding), S element, lateral view, sample POCC, ×45, CORD-MP 11265, CAI 7. (ac) Drepanoistodus basiovalis (Sergeeva), S element, lateral view, sample A4, ×60, CORD-MP 11261, CAI 6.5. (ad) Drepanoistodus forceps (Sergeeva), S element, lateral view, sample POCC, ×40, CORD-MP 11264 CAI 7.5. (ae) Spinodus spinatus (Hadding), S element, lateral view, sample P6, ×90, CORD-MP 11266, CAI 8. (af) Paroistodus horridus (Barnes & Poplawski), S element, lateral view, sample POCC, ×98, CORD-MP 11267, CAI 8. Illustrated specimens are optical pictures (stereomicroscope Nikon SMZ 800, S4).
4.b. CAI and microstructure of conodonts
The studied specimens exhibit variable taphonomic characteristics that, in most cases, depend directly on the distance to the intrusions (Table 1, Figs 4, 5). Conodonts recovered from unweathered or unaltered rocks are pale yellow to light amber and present a smooth lustre. Grained and pitted surfaces develop in response to etching and corrosion (Epstein, Epstein & Harris, Reference Epstein, Epstein and Harris1977). In close proximity to the Puerta de Ancaucha basic sill, conodonts exhibit a sugary texture. At 0.35–12.2 m away from the intrusive bodies, texture changes from sugary to increasingly vitreous. On the other hand, all conodont samples from the El Salto section present a uniformly distributed sugary character that reflects a higher grade of corrosion.
Table 1. Conodont samples distribution with CAI values and key textural observations
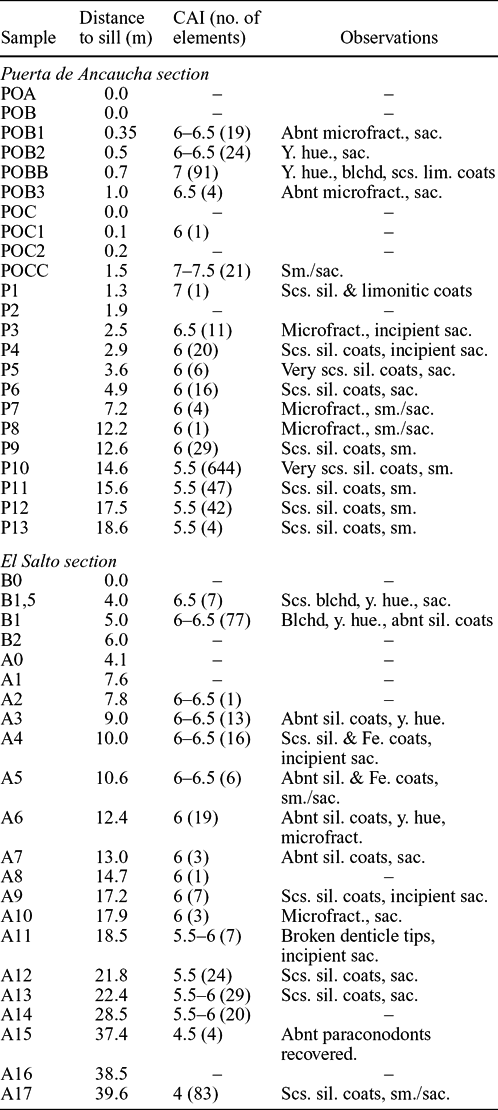
Abnt – abundant, scs. – scarce, microfract. – microfractures, y. – yellow, Fe. coats – ferric oxides coatings, sil. coats – siliceous coatings, blchd – bleached, sac. – sacaroid bright, sm. – smooth bright.

Figure 5. Line-art drawings of the Puerta de Ancaucha studied section. Conodont samples with petrographic slides are indicated by black squares. The inset figure shows detailed sampling through the contact zone.
Conodont samples from both sections lack any patinas. Samples POBB, B1.5 and B1, located at 0.70 m, 4 m and 5 m, respectively, from the intrusion contacts, are bleached and abnormally corroded. Usually, these conodonts present subcircular dissolution sags aligned through microfissures; dispersed corrosion features are less common. In addition, several specimens from samples B1.5, B1 and A6 taken at 4 m, 5 m and 12.4 m, respectively, from the contacts, contain a yellow hue, indicative of staining effects by trace amounts of iron (cf. Königshof, Reference Königshof2003). In the Puerta de Ancaucha section, only 35 % of sample POB2 exhibits a yellow hue.
Although tectonic deformation in the Yerba Loca Formation is complex and largely distributed, no ductile or brittle deformation patterns in conodonts (cf. Rejebian, Harris & Huebner, Reference Rejebian, Harris and Huebner1987; Königshof, Reference Königshof2003) from Ancaucha are observed. Microfractures are sparse, and are apparently responsible for the lack of apices in coniform and ramiform elements. These occurred as a conjugated family oblique to the apices and/or parallel to the elements base.
Usually, conodont surfaces are free of mineral overgrowths, limited to scarce clusters of quartz, unidentified silicates and ferric oxides. CAI values are quite uniform within the same samples; no more than 0.5 degree of dispersion is observed. On rare occasions, very delicate or robust conodonts that provided anomalous values are disregarded in our analysis. Evidence of CAI decrement is shown by plotting values of conodont samples against distance to the intrusion (Fig. 5).
The regional CAI background of the Yerba Loca Formation is observed at the Pantanito section (30°5.28′ S, 68°54.45′ W), where well-preserved conodonts exhibit a uniform CAI 3. This is the lowest CAI value recorded from the Yerba Loca Formation, and it can be considered as the background value.
Hydrothermalism distorts CAI patterns, which delimit the thermal aureoles produced by intrusions. Under the presence of oxidizing hydrothermal solutions, conodonts generally become corroded and coarsely recrystallized (Rejebian, Harris & Huebner, Reference Rejebian, Harris and Huebner1987; Königshof, Reference Königshof2003), obliterating the original CAI value up to a few metres from the intrusion. Consequently, bleached elements with high CAI values were not included in the calculations.
4.c. Petrography
As described by Fernández-Noia, Sumay & Meissl (Reference Fernández-Noia, Sumay and Meissl1990), the dark-green to black igneous bodies with sharp contacts from Ancaucha are gabbroic sills. Under the microscope, these rocks display a diabasic texture, with clinopyroxenes, orthopyroxenes and Ca-rich plagioclase as major minerals. Apatite inclusions and opaque minerals (ilmenite, magnetite and pyrite) are present in lower quantities. Low-grade metamorphism is responsible for the alteration of mafic minerals and feldspar to serpentinite, chlorite, minor biotite and quartz (cf. Robinson, Bevins & Rubinstein, Reference Robinson, Bevins and Rubinstein2005).
On the other hand, seventeen conodont samples from both measured sections at Ancaucha were analysed using optical microscopy (Fig. 6). The fine-grained nature of the altered rocks precludes accurate determination of the paragenetic sequence of minerals. Distal turbidite sets are here composed of meta-calcisiltites, claystones and chert that seldom exceed 20 cm in thickness, and many measure less than 7 cm. The meta-calcisiltites are massive to well-bedded, very fine- to coarse-grained rocks that generally contain small amounts of quartz (probably detrital and recrystallized from diagenetic chert), biotite, chlorite and muscovite. Traces of opaque minerals and brown oxides are distributed throughout studied sections, preferably concentrated in sparry-filled veinlets and often accompanied by scarce chlorite.

Figure 6. Macro- and microphotographs of described rocks from Puerta de Ancaucha and El Salto sections. (a) Detailed view of the sedimentary banding enhanced in proximity to the El Salto sill. Hammer is 33 cm long. (b) Textural relationships among Garnet + Zoisite + Quartz fracture fill and later scapolite replacement, sample POA. (c) Annealing of calcite grains and incipient polygonal fabric. Microstylolites also visible, sample POBB. (d) Patchy recrystallization of very fine silt to pseudosparite, sample A17.
Sample POA was recovered from the host rock, just on the contact with the Puerta de Ancaucha basic sill. It exhibits a porphyroblastic texture composed of subidioblastic Ca-rich garnet and zoisite of up to 0.16 mm in width embedded in a fine-grained carbonate matrix, with scarce subangular detritic quartz fragments. Locally within this slide, garnet and zoisite porphyroblasts display σ-structures enveloped by elongated calcitic crystals, which in turn define discontinuous foliation bands in the matrix (C-type texture of Kóvac & Árkai, Reference Kovács, Árkai and Austin1987). A later metasomatic event is probably indicated by growth of scapolite, quartz and rare titanite crystals. Deformed domains are bounded by en echelon faults, ultimately filled with garnet, zoisite and quartz (Fig. 6b). The brittle deformation can be traced up to a mesoscopic scale, particularly in proximity to the intrusive bodies, where the host rock is intensively cracked. Nevertheless, non-coaxial microscopic deformation was observed only in sample POA.
Thermal influence from gabbroic sills is recorded several metres away from the contact. Samples POB1 and P5 from Puerta de Ancaucha, and A5 from El Salto, were taken 0.35 m, 3.6 m and 10.6 m away from the intrusions, respectively. These samples are inequigranular hypidiotopic, medium to coarse, recrystallized calcisiltites. Subhedral garnet porphyroblasts with scarce quartz, zoisite and scapolite arise as subordinate phases, commonly as isolated grains within the calcite matrix. In particular, sample A5 contains rare euhedral vesuvianite and patchy darker zones as relicts of the fine-grained calcitic silt. It is worth mentioning that these samples produced conodonts. None of other studied samples exhibit contact metamorphism/metasomatic mineral associations, but show a variable degree of recrystallization and neomorphism, with incipient polygonal fabrics and annealing of carbonate grains (Fig. 6c). Many of the rocks retain sedimentary features such as bedding, cross-stratification and detritic clasts. Very fine calcisiltites present patchy recrystallized areas, filled with neomorphic sparite (pseudospar) (Fig. 6d). Mottled patterns indicate shallow and deep burial diagenetic environments, probably related to relative small quantities of organic carbon and/or clay minerals, which inhibit or prevent crystal coarsening (Robinson, Reference Robinson1971; Flügel, Reference Flügel2004). All samples except for POA exhibit a B-type texture, according to Kóvacs & Árkai's (Reference Kovács, Árkai and Austin1987) criteria, that is, weak preferred orientation and incipient foliation in the matrix.
4.d. Clay mineralogy
The clay mineral assemblage identified in most of the 12 analysed samples is composed of chlorite ± illite ± smectite ± I/S mixed-layer. However, chlorite was absent in sample POC, which was only composed of I/S and smectite. Chlorite was identified for its rational basal reflections at 14.1, 7.1, 4.73 and 2.83 Å in the pattern of the air-dried oriented mount; these do not show significant changes after EG treatment. In most of the samples, chlorite reflections remain unchanged after heating, although a slight decrease in intensity was observed in some cases. Illite basal reflections (10.1, 5.05, 3.33 and 2.50 Å) remain almost unchanged after EG and heating treatments. Smectite was identified for its shift towards ~ 16–17 Å after EG treatment, and the collapse to 10 Å in the pattern after heating. Illite/smectite mixed-layer was identified in several samples for a peak at 12.5–13.2 Å in the air-dried pattern that shifts towards 13.5–14 Å in the XRD pattern of the EG-treated specimen, which, in turn, collapsed to 10 Å after heating. CIS could only be determined in three samples, in which illite was present in appreciable amounts; the values obtained (0.27–0.32) indicate anchizone metamorphism.
Traces of dolomite, manganese calcite and magnesium calcite were identified in the fine fractions analysed, although samples were treated with acetic acid–sodium acetate buffer prior to clay analysis.
4.e. Theoretical model of magma cooling and the thermal regime of the host rocks
The depth of intrusions below the water–sediment interface was inferred to be in the order of 1 km on the basis of Upper Ordovician stratigraphic reconstructions and rock petrography. Different thicknesses of intrusions (sills of 30, 40 and 50 m thick) were tested; no significant differences were observed in maximum temperatures reached by the surrounding sediments. For example, at 30 m away from the contact, modelled maximum temperatures were similar, despite the different intrusion thicknesses. Consequently, it is possible to consider the thermal effects produced by both intrusions as analogous. Hence, the palaeothermometric data obtained from both profiles were integrated into a single ideal section. The thermal anomaly produced by the intrusions almost immediately overprinted the surrounding sediments, but required several years to expand tens of metres away. After ten years, elevated temperatures decline to near background conditions.
Thermal modelling parameters included a surface temperature of 0 °C and a geothermal gradient of 30 °C km−1. The emplacement of the molten layer was considered to be instantaneous and to have occurred at a constant temperature of 1100 °C, in accordance with measured temperatures in active volcanic areas and experimental research (e.g. Pinkerton, James & Jones, Reference Pinkerton, James and Jones2002; Yoder & Tilley, Reference Yoder and Tilley1962). It was also assumed that the intrusion had a thermal conductivity of 2.6 W m−1K−1, a heat capacity of 1150 J kg−1K−1, and that it experienced no further movement, nor loss or gain of mass (e.g. Suchý et al. Reference Suchý, Šafanda, Sýkorová, Stejskal, Machovič and Melka2004). In our models of pure conductive heat transfer, a typical value of 1 W m−1K−1 was assigned to the host rock, considering unconsolidated, water-saturated claystones (Galushkin, Reference Galushkin1997). Analysis of heat transfer by fluid flow was estimated in three ways: (a) by enhancing the thermal conductivity of the surrounding sediments up to 5 W m−1K−1 (cf. Galushkin, Reference Galushkin1997); (b) taking into account a maximum sediment porosity of 20 % to allow fluid convection; and (c) combining mentioned alternatives. Following all of these specifications, the palaeotemperatures determined through the conodont CAI and the cooling curves modelled for different time intervals are presented in Figure 7.

Figure 7. Conodont CAI temperatures and thermal decrement curves for the Ancaucha sills.
5. Discussion
Conodont textures indicate a hydrothermal activity that was restricted to a small volume of rock close to the intrusions at Ancaucha creek. Apart from the bleaching of specimens in samples POBB, B1.5 and B1, no other conodont textural evidence (e.g. patinas, coarse recrystallization) or high variability of CAI values within samples supports fluid circulation (cf. Rejebian, Harris & Huebner, Reference Rejebian, Harris and Huebner1987; Harris et al. Reference Harris, Rexroad, Lierman and Rosemary1990). Moreover, only four conodont samples provided elements with yellow hues. Staining effects in these conodonts are probably related to complex geometrical pathways for fluid flow (cf. Nabelek, Reference Nabelek2002). Bituminous staining is implausible, given that the close proximity to the intrusions provided enough heat to volatilize the carbonaceous components (Table 1). Considering a linear CAI temperature decrement and pure conductive heat transfer, the thermal anomaly extends to up to 2.5 times the thickness of intrusions (Fig. 7). Conodont CAI values across both profiles suggest that the contact aureoles from the intrusions prevailed over the superimposed regional very low-grade metamorphism.
From seventeen analysed slides, five samples within 10 m of the intrusions displayed metasomatic associated minerals (e.g. garnet, zoisite). Opaque minerals as trace components are distributed not only in these samples but in all samples from both profiles. The B- and C-type meta-calcisiltitic textures determined in the host rock indicate anchizone to epizone domains (Kovács & Árkai, Reference Kovács, Árkai and Austin1987). The occurrence of C-type texture adjoining the mafic bodies is connected to forceful intrusion mechanisms. A few centimetres from the contact, the C-type texture is replaced by the B-type, which is found throughout the host rock. Burnett (Reference Burnett1988) documented altered carbonate textures up to 4 m from the contact of the 30 m wide Holy Island Dyke. The great extension of the B-type texture domain, which occurs throughout the study area, and the conodont CAI 3–4, as regional background, suggest that most carbonate textural alterations were produced within the anchizone domain.
The association of clay minerals determined in most of the analysed samples is complex and probably derives from several superimposed processes. The widespread occurrence of smectite and I/S, in some cases coexisting with illite, even in samples with KI values corresponding to anchizone metamorphism, can only be interpreted as non-equilibrium clay mineral assemblages. The I/S mixed-layers are typical of diagenesis and weak anchizone, whereas smectite is characteristic of early to late diagenesis with a stability range up to 180 °C. The few samples in which KI could be measured were taken tens of metres away from the sills (18.6, 37.4 and 39.6 metres, respectively). These KI values are in the range of anchizone-grade metamorphism in accordance with regional background CAI values. These values thus do not seem to have recorded the thermal aureole produced by the intrusion; on the contrary, they probably reflect the temperatures prevailing during regional metamorphism. In order to understand these results we have to consider that the KI method is a measure of the reaction progress within the prograde illite–muscovite series, which is a kinetically controlled and irreversible transformation (Warr, Reference Warr1996). For this reason, the KI index is predictably less sensitive than organic matter maturation indexes (e.g. CAI, vitrinite reflectance) to short-term metamorphic heating. Moreover, it has long been recognized that organic matter reacts much more sensitively to rises in temperature than minerals do (Teichmüller, Reference Teichmüller and Frey1987).
It is worth noting that illite is not present in the samples taken at short distances from the sills, which in turn contain smectite. This distribution of smectite represents a clear indication of the later origin of this mineral, which would be unstable during the heating event produced by the intrusion, but also under the temperatures in the range of 200–300 °C characteristic of the regional anchizone metamorphism. It has been suggested that pure smectite, in contrast with I/S (< 50 % I), is not a common prograde phase in sedimentary rocks, except in bentonites, as most of the successions have experienced some degree of low-temperature metamorphism leading to the formation of illite layers from the smectite precursor, thus giving rise to I/S mixed-layers (S. Hillier, pers. comm. in Nieto et al. Reference Nieto, Mata, Bauluz, Giorgetti, Árkai and Peacor2005). This applies to the Yerba Loca Formation which, according to the regional background CAI value, underwent anchizone metamorphism. Consequently, smectite and I/S are inferred to result from retrograde diagenesis processes (cf. Nieto & Peacor, Reference Nieto and Peacor1993).
Our numerical modelling for pure conductive heat transfer predicts a thermal aureole narrower than the one depicted by empirical data from conodont CAI, as controlled by energy laws of conservation. However, deduced contact zone temperatures from both methods are quite similar (> 600 °C). This is supported by numerical models and organic metamorphism studies published elsewhere (e.g. Burnett, Reference Burnett1988; Galushkin, Reference Galushkin1997; Suchý et al. Reference Suchý, Šafanda, Sýkorová, Stejskal, Machovič and Melka2004). Most conodonts from Ancaucha creek show high CAI values but no apparent signs of hydrothermalism. This is in agreement with the poorly altered carbonate rock fabric. In particular, CAI values of 6 or greater produced during hydrothermal alteration are not related to temperature–time conditions. Instead, these values are prone to reflect maximum temperatures in contact-metamorphic settings (Rejebian, Harris & Huebner, Reference Rejebian, Harris and Huebner1987).
The CAI 4 from sample A17 falls within background values and it could be reflecting either contact or regional metamorphism. However, because a KI of 0.30, indicative of anchizone metamorphism, was measured for this sample, it is possible to conclude that maximum temperatures from regional metamorphism did not exceed 300 °C. Thus, recorded conodont CAIs from 7 to 4 are reflecting at least a temperature fall from 630 to 300 °C across 39.6 m, approximately, in the host rock.
What do high conodont CAIs indicate within the zone influenced by intrusions? Generally, mafic intrusions crystallize without releasing large amounts of volatiles. At Ancaucha creek, the fine-grained nature of the siliciclastic rocks probably buffered fluid circulation during heating and devolatilization of the mafic sills. However, higher vapour pressures induced calcite recrystallization, which in turn enhanced permeability and porosity. This fact, alternatively, favo-ured transient material transport into the wall rock and metasomatic alteration, while carbonates acted as pathways for metasomatic fluids into the sedimentary sequence. The fine-grained siliciclastic deposits proximate to the intrusives were silicified instead. Apparently, the contact metamorphic mineral parageneses were inhibited by the small size of the basic bodies intruding shallow crustal levels, whereas the conodont CAI reflected temperatures transmitted by the advancing metasomatic front. These CAI values probably indicate oxidation of the organic matter within the conodont structure by the high temperatures of the system (> 300 °C). This situation could be compared to that in seafloor spreading ridges, where hydrothermal alteration reactions of the organic matter are primarily reductive (Simoneit, Reference Simoneit and Parnell1994).
6. Conclusions
The basic intrusions in Ancaucha creek, Western Precordillera, which were affected by the Siluro-Devonian very low-grade metamorphism, are dated as post-Darriwilian (Da2) by conodonts recorded from the host rock (P. horridus Subzone of the L. variabilis Zone).
KI as well as regional background CAI indicate a low–medium anchizone domain for this regional event at Ancaucha creek.
A gradient of the conodont CAI is apparent in plotted values against distance to the intrusions. The distribution of conodont CAI values defines a metasomatic contact aureole that prevailed over the later very low-grade metamorphic event.
The characteristic banded bedding observed across the host rock was caused by hydrothermal activity and preferential silicification of the siliciclastic fraction in the sedimentary sequence.
The conodont CAI temperatures extrapolation at the contact zone is in accordance with theoretical temperatures obtained from numerical modelling (> 600 °C).
The metasomatism at Ancaucha creek produced CAI values of 4–7 with little conodont textural alteration (e.g. staining effects, coarse recrystallization, patinas and yellow hues), although locally, the conodont elements were bleached and highly corroded.
Acknowledgements
The senior author undertook this study as part of his doctoral thesis in the Universidad Nacional de Córdoba, Argentina. We are grateful to R. Lira (Univ. Nac. Córdoba) for his assistance with mineral determinations and helpful criticism. E. Baldo and S. Verdecchía (Univ. Nac. Córdoba) are thanked for their help with optical petrography. K. Wohletz (Los Alamos National Laboratory, USA) provided insightful discussions to interpret the theoretical model. We thank P. Smith and an anonymous reviewer, who helped us to improve the manuscript. This research was funded by SECYT-UNC-2006/07 and CONICET 2006/7 grants to G. L. Albanesi, G. G. Voldman and G. Ortega.