NOMENCLATURE
- ANOPP
Aircraft Noise Prediction Program
- CFR
Code of Federal Regulations
- EPNL
Effective Perceived Noise Level
- FAA
Federal Aviation Administration
- FLOPS
Flight Optimization System
- ISA
International Standard Atmosphere
- NASA
National Aeronautics and Space Administration
- NPSS
Numerical Propulsion System Simulation
- VNRS
Variable Noise Reduction System
1.0 INTRODUCTION
Noise standards and recommended practices for new supersonic civil aircraft do not currently exist. Until new regulations are developed, the brief text relevant to supersonic aircraft in Chapter 12 of Ref. 1 states that noise limits given for subsonic aircraft may be used, provisionally, as a guideline. For evaluation purposes, we use subsonic certification procedures to predict noise levels in this article. This is due to the anticipation of the authors – given the guidance in Chapter 12 – that new supersonic jet aircraft will be required to certify under subsonic aircraft regulations.
Applicants seeking a noise-type certificate for new subsonic-jet and large subsonic-transport aircraft are subject currently to the regulations of Chapters 4 and 14 of Ref. 1. The newest noise limits described in Chapter 14 are implemented in phases. While large aircraft (having maximum gross weights over 55 tonnes) are required to meet Chapter 14 limits if the application is received after 2017, smaller aircraft are eligible to certify under older, less demanding noise limits defined by Chapter 4 until 31 December 2020. Further, in the United States and in other countries, flight certification tests may be delayed 5 additional years after the application date. Thus, an opportunity exists for a transport in the smaller business class – weighing less than 55 tonnes – to certify under Chapter 4 noise limits but not begin noise certification tests until 2025. This article documents the study of a notional Mach 1·4 supersonic business jet in this category.
The requirement of supersonic flight leads to significant differences in configuration, aerodynamics and propulsion relative to a subsonic aircraft. A supersonic business jet is likely to have a thin wing with a low aspect ratio and a simple flap system. It is likely to require high take-off speed before sufficient lift is generated to lift off, and even higher airspeeds to climb with significant thrust margin. But normal noise reference procedures require applicants to climb at airspeeds no greater than 20 Kn over than the take-off safety speed (this is the “not greater than V2 plus 20 Kn” requirement defined by 3.6.2(d)(1) of Ref. 1). It is possible the applicant may want to apply for a departure from this reference procedure to ensure adequate climb performance. Provisions for departures from normal procedures are explained in 3.6.1.4 of Ref. 1. A departure from normal procedures is allowed if the applicant can show the aircraft performance characteristics demand it and if the certificating authority approves it.
Another possible departure from normal procedures involves engine take-off power settings. Experience in aircraft sizing analyses suggests that supersonic aircraft are likely to be constrained by a take-off field length requirement. Therefore, high engine power settings are required during the ground roll and climb-out phases of take-off. But engines capable of supersonic cruise tend to have high specific thrust, high exhaust velocity, and high levels of jet noise, making noise certification a challenging prospect. Reducing jet noise at the lateral measurement condition, where engine power is maximum, is particularly challenging (A sketch showing the arrangement of the noise measurement monitors is shown in Fig. 1). Researchers during NASA's Supersonic Cruise Research Program(Reference Grantham and Smith2) proposed a certification noise reduction procedure to address noise at the lateral condition. This procedure since has become known as the auto-throttle or programmed thrust lapse take-off(3).
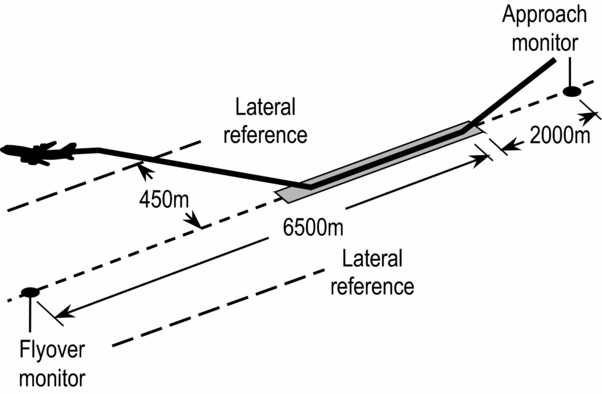
Figure 1. Noise certification monitor arrangement relative to take-off and landing flight paths.
As typically envisioned, an advanced take-off using a programmed thrust lapse begins ordinarily, with maximum thrust applied from brake release through rotation and lift-off. Propulsion noise is highest at maximum thrust, but for observers located laterally across from the aircraft, noise is very efficiently attenuated by ground effects. Lateral attenuation is caused by ground surface absorption, by refraction and scattering effects of the air, and by engine-airframe installation effects. At low elevation angles, these effects can attenuate sound along the lateral sideline by as much as 10 dB(4). The programmed thrust lapse procedure exploits lateral attenuation.
Immediately after the runway obstacle is cleared (but well before the conventional, pilot-initiated throttle cutback takes place), engine thrust is automatically lowered to reduce lateral sideline noise. The programmed thrust lapse is more gradual than the rather abrupt pilot-initiated throttle cutback occurring later. Ideally, the throttle is reduced as the benefit of lateral attenuation vanishes with increasing altitude. It would likely be implemented over the time required for gear retraction and be completed before the second segment climb begins.
Thus, capitalising on lateral attenuation via a thrust lapse procedure is a clever idea. Lateral noise is abated, but because take-off thrust is at maximum through obstacle clearance, take-off field length does not increase.
However, thrust lapse procedures at low altitude may not be allowed under current regulations. Changes to engine power are not permitted by Section 3.6.2(a) of Ref. 1. This regulation defines minimum safe altitudes (210 to 300 meters, depending on the number of engines), below which engine power setting must remain at maximum. Without permission to operate otherwise, regulations rule out part-power take-offs for noise certification and would seem to disallow thrust lapse procedures at low altitude. And regulating authorities might be reluctant to approve a pilot-initiated procedure that would increase the workload of the flight crew during take-off and reduce the rate of climb.
Still, it is thought that a departure from normal reference procedures could be permitted if computer-controlled automatic throttle scheduling is used, making pilot initiation unnecessary. An automatic digital engine control implementation of a programmed thrust lapse could use an aircraft's weight-on-wheels sensors, airspeed, altimeter, attitude and air temperature indicators, and perhaps airport navigational aids to begin pre-programmed thrust reduction.
Afterburning during take-off to shorten field length was used by the Concorde and has been proposed for some future supersonic transports (e.g., Reference BertonRefs 5, Reference Morgenstern6). However, unlike the Concorde, use of afterburners would need to be restricted to just the ground roll due to noise concerns. Even so, lateral ground attenuation may be insufficient to abate noise generated on the runway unless the degree of reheat is limited. A programmed thrust lapse would need to consist of a “wet-to-dry” conversion as landing gear is retracted. This would require the nozzle throat to close just after lift-off. No take-off afterburning is considered in this study.
Along with an accelerating climb-out, a programmed thrust lapse likewise may require approval for a departure from normal take-off reference procedures defined for subsonic aircraft. This article documents the initial development of a non-proprietary, public-domain analytical NASA model of a supersonic business jet upon which studies can be performed. The accelerating climb-out and the programmed thrust lapse procedure (and their apparent necessity for supersonic transports) are the focus of this study. Our intent is to use the model to study take-off procedures and their influence on certification noise. Approach noise is also predicted, but only insofar as to estimate cumulative noise margin with respect to Chapter 4 limits. Noise abatement in an operational setting beyond noise certification is not addressed.
2.0 ANALYSIS AND DISCUSSION
2.1 Engine analysis
It is unlikely a completely new engine could be developed and ready in time for 2025 flight tests. Instead, it is more likely the low-pressure spool of a contemporary off-the-shelf engine would be redesigned, resulting in a supersonic variant of an existing subsonic turbofan. In this study, an analytical model of a subsonic CFM56-7B is used as the “donor” engine from which the supersonic engine is derived. Interestingly, the original CFM56-2, granted certification in 1979, was itself derived from the GE F101 and 102 used for the supersonic B-1A bomber. So redesigning the low-pressure spool of a CFM56 once again for a supersonic application would bring the engine family full circle.
Because much engine design data are closely held, proprietary and unavailable, any analytic simulation of a CFM56 (outside of CFM International) will necessarily have some inherent inaccuracy. Nevertheless, if data can be obtained from public-domain sources (such as type certificate data sheets, manufacturer-provided operating documents, technical reports and manufacturer's websites), simulations of turbofans developed outside of engine companies can be reasonably accurate. The model of the subsonic CFM56 is created with such information using the Numerical Propulsion System Simulation code (NPSS)(Reference Claus7,8) to predict engine performance. NPSS is an engine-cycle analysis tool developed jointly by NASA and United States aerospace industry. It is currently the accepted, state-of-the-art software for air-breathing engine cycle performance analysis for United States industry, academia, and NASA. The CFM56 model is adapted from work performed under the FAA's Environmental Design Space initiative(Reference Kirby and Mavris9).
The low-pressure spool of the CFM56-7B is redesigned for a Mach 1·4 cruise application. The booster is discarded (with it, supersonic ram effects would elevate aft stages of the compressor to an excessive temperature), and the fan and low-pressure turbine are redesigned for a higher pressure ratio. Fan performance is modelled using data collected at NASA from the GE57 scale model fan(Reference Suder, Prahst and Thorpe10). The GE57 fan is considered to be perhaps representative of what might be used by an engine manufacturer in a supersonic refan application. It consists of a single stage, has no inlet guide vanes and operates at peak efficiency at a pressure ratio of 2·2. Fan pressure ratio is a design variable strongly influencing engine performance. A high fan pressure ratio is preferred to create exhaust velocity high enough for supersonic flight, while a low fan pressure ratio is required to meet take-off and landing noise requirements. Fan pressure ratio, along with a practical extraction ratio, directly determines the bypass ratio of the engine. This poses conflicting requirements for supersonic engine designers. In this study, an initial mixed-flow engine cycle was studied using unscaled GE57 fan performance data. This led to supercritical nozzle pressure ratios at low altitude and high levels of jet-shock cell noise during take-off. That engine design was deemed untenable and discarded. The current engine cycle in this study uses the GE57 fan data, but with pressure ratios scaled low enough that the nozzle is on the cusp of choke near sea level. With a small amount of engine derating at low altitude and a programmed thrust lapse, jet-shock cell noise is eliminated after lift-off.
Another key design choice is whether to forcibly mix the core and bypass streams or to allow them to remain separate. There are compelling reasons to mix the streams. There is usually an increase in gross thrust when flows are forcibly mixed and exhausted through a common nozzle, with the benefit increasing with increasing core stream temperature. Although the benefit is slight, it can be important to net thrust at high speed when ram drag is high. And the outer mould lines of a single-stream nozzle are preferred over those of a more complex co-annular nozzle if sonic boom reduction is important. In this study, core and bypass streams are forcibly mixed through a lobed mixer. The design extraction ratio is kept near unity so that mixer bypass port and mixer exit Mach numbers are always less than 0·5.
The mixed flow exits through a single-stream convergent-divergent plug nozzle. The centre-body plug and nozzle throat are fixed while the divergent flaps are variable. The plug is important in keeping aft-body boat-tail angles small during supersonic cruise while also lowering jet noise slightly. At low altitudes, the divergent nozzle flaps are closed to a minimum area so that the nozzle exit plane is the throat. Solid models of the CFM56-7B and the derived supersonic turbofan are shown in Fig. 2.
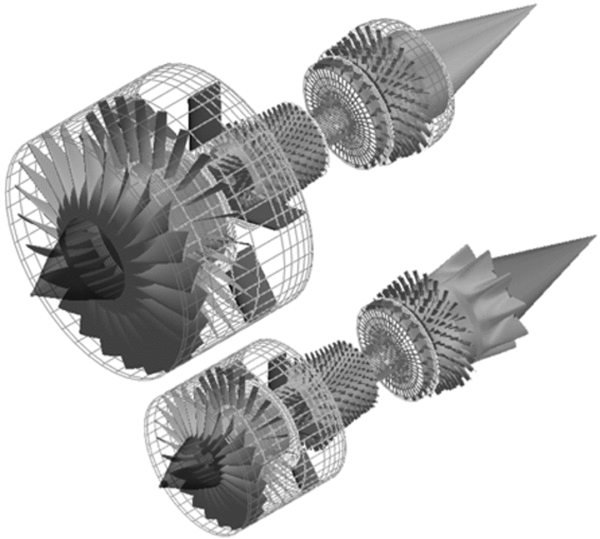
Figure 2. Solid models of the CFM56-7B (top) and notional modified supersonic variant (bottom).
At the cycle design point, the compressor and the high-pressure turbine are held in off-design mode. NPSS performance map scalars of the compressor and high-pressure turbine from the CFM56 donor engine are manually specified. This keeps the core of the supersonic derivative engine identical to the CFM56 core. Bleed flow fractions and core flow passage areas are also held constant. Hot-section temperatures are kept nearly as high as the CFM56 maximum take-off temperatures. But since the supersonic variant would spend several hours at maximum temperature (compared to just a few minutes during take-off for a subsonic turbofan), this becomes a rather important assumption. Maintaining high temperatures is justified by assuming increased hot-section overhaul frequency (not uncommon for a business jet application), new turbine aerofoils with improved materials and coatings, and better cooling effectiveness. A summary of engine performance data is shown in Table 1. Ambient conditions at altitude use International Standard Atmosphere (ISA) conditions, while conditions near sea level use standard hot day (ISA+15°C) conditions. Performance data at sea level are shown after engine derating.
Table 1 Supersonic derivative engine performance summary

And although not studied, a separate flow turbofan may be an interesting alternative engine design. The bypass stream of a co-annular nozzle provides an effective means to reduce the shear velocity of a supersonic core jet. This reduces Mach wave radiation that can be the principal noise source of a supersonic jet. A separate flow turbofan could be an engine architecture that allows fan pressure ratio to be higher while still keeping jet noise at acceptable levels.
2.1.1 Airframe analysis
Most properly, an aircraft conceptual design should begin with a study to determine basic vehicle performance, payload and mission requirements. Concurrently, an experienced aircraft designer would loft a vehicle and define its geometry well enough to predict its aerodynamics and weight. A performance constraint and sizing analysis would determine optimum vehicle characteristics to perform the desired mission. Airframe and propulsion analysts would team together and iterate the process until closure. Due to time and resource constraints, however, this usual practice is replaced with a simpler approach. The scope of this study is to assess advanced take-off procedures and their impact on noise, and so the focus is on propulsion design, take-off performance and noise predictions. Insight can be gathered with reasonable assumptions for airframe performance. In subsequent phases of this study, more complete vehicle analysis will be made.
A supersonic business jet with a maximum gross weight of 55 t (121,000 lb) is selected for this study for reasons discussed in the introduction. Engines are designed for a Mach 1·4 cruise at 15·2 km. Three engines are necessary to provide sufficient thrust for cruising if the vehicle's lift-to-drag ratio is approximately 10. The engines are assumed to be mounted on top of the wing and fuselage to shield forward-radiating fan noise from observers on the ground during take-off and approach. The nozzle exit planes extend aft of all airframe surfaces; thus fan exit noise, core noise and jet noise – a distributed noise source in any case – do not benefit from wing shielding. A solid model of the vehicle concept is shown in Fig. 3.

Figure 3. Solid model of notional supersonic business tri-jet.
Low-speed aerodynamics are required to compute take-off and approach trajectories necessary for a certification noise analysis. Aerodynamics in the form of scalable lift and drag coefficients are taken from a Boeing study of a Mach 1·6, 82 t supersonic transport(Reference Welge11). Lift and drag coefficients as functions of angle-of-attack are given for three flap settings. It is assumed that if the wing planform and high lift devices of the Boeing vehicle and our reference vehicle are similar, then the low-speed aerodynamics may also be similar. A wing reference area corresponding to a take-off wing loading of 4300 N/m2 is assumed in order to compute actual aerodynamic forces for a trajectory analysis. In later phases of this study, the actual wing loading will result from an aircraft sizing analysis.
2.1.2 Take-off analysis
Take-off and climb at high speed may be preferred for many supersonic aircraft. This preference can be shown for the supersonic business jet in this study by constructing its thrust demand curves. These are shown in Fig. 4 for level and steady flight at maximum weight using take-off flaps at an altitude of 600 meters. Unlike a medium subsonic commercial aircraft of similar weight in these conditions, which may have a minimum drag speed of perhaps 200 Kn, our supersonic business jet's minimum drag speed is predicted to be nearly 300 Kn. Speeds below the minimum drag speed are in the so-called region of reversed command, where to fly more slowly requires more thrust to overcome increasing lift-dependent drag. Flying safely in this region requires adequate thrust margins. If climb-out speeds are limited by 3.6.2(d)(1) of Ref. 1, very high rotation and lift-off speeds would seem to be in order, so that the climb-out would take place at as high a speed as possible. Since modern aircraft tires are rated to approximately 200 Kn, rotation speed in this study is assumed to occur at 190 Kn. This results in a lift-off speed of 196 Kn and a take-off safety speed of 202 Kn.
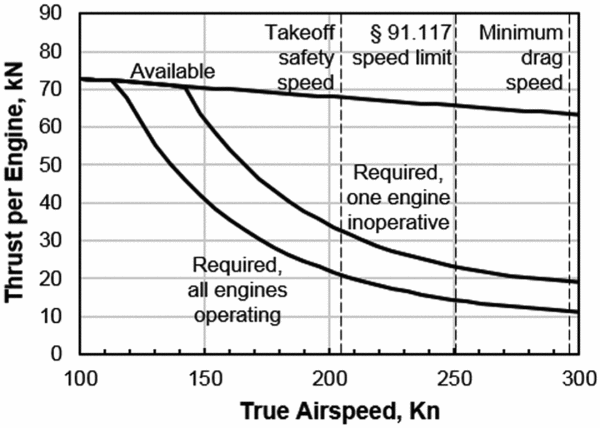
Figure 4. Thrust demand of supersonic business jet for level steady flight at 600 m.
Even so, 202 Kn is still well inside the region of reversed command. This demonstrates the need for high-speed ground rolls (delayed rotations) and quite possibly an accelerating climb-out. Seemingly, an excellent case could be made to permit a departure from the climb-out speed limit requirement defined by 3.6.2(d)(1) of Ref. 1.
In addition, unless permission is granted otherwise, flight in excess of 250 Kn under 10,000 feet is prohibited in the United States (and in many other countries) by the Code of Federal Regulations 14 CFR § 91.117(a). Accelerating climb-outs are studied in this report, but they are limited to speeds under 250 Kn.
2.1.2.1 Standard take-off procedures
A take-off field length calculation in accordance with 14 CFR § 25 is made for this aircraft using NASA's Flight Optimisation System, FLOPS(Reference McCullers12). It is assumed to rotate at an angle-of-attack of 13°. Maximum usable lift coefficient is assumed to be 1·0. For the critical outboard engine failure calculation, engine-out yaw drag is estimated using handbook methods. Balanced field length is calculated to be 2,300 m; however, the take-off field length is, instead, determined by 115% of the all-engines operating field: 2,340 m. In either event, field lengths of this distance should be satisfactory for this class of aircraft, giving credibility to the take-off wing loading assumed earlier. The minimum second segment climb gradient of 2·7% required for tri-jets with one engine inoperative is satisfied.
Next, a take-off calculation for noise certification from a sea level field on a standard acoustic day is made in accordance with the requirements in 3.6.2 of Ref. 1. No climb-out acceleration or programmed thrust lapse is performed. Above the minimum safe altitude, engine power is reduced during a pilot-initiated cutback such that the climb gradient is 0 with one engine inoperative, or 4% with all engines operating. The engine power cutback is designed to reduce noise at the flyover monitor. Many certification applicants vary the time and position of the cutback (using an equivalent procedure) to minimise noise slightly at this location. In this study, however, the cutback is complete 5,200 m from brake release, and the flyover noise is determined entirely by the cutback power noise signature. This take-off is depicted by the solid lines in the plots for steady, non-accelerating climb-outs in Fig. 5. This standard take-off is permissible without departures from normal procedures defined by 3.6.2 of Ref. 1.
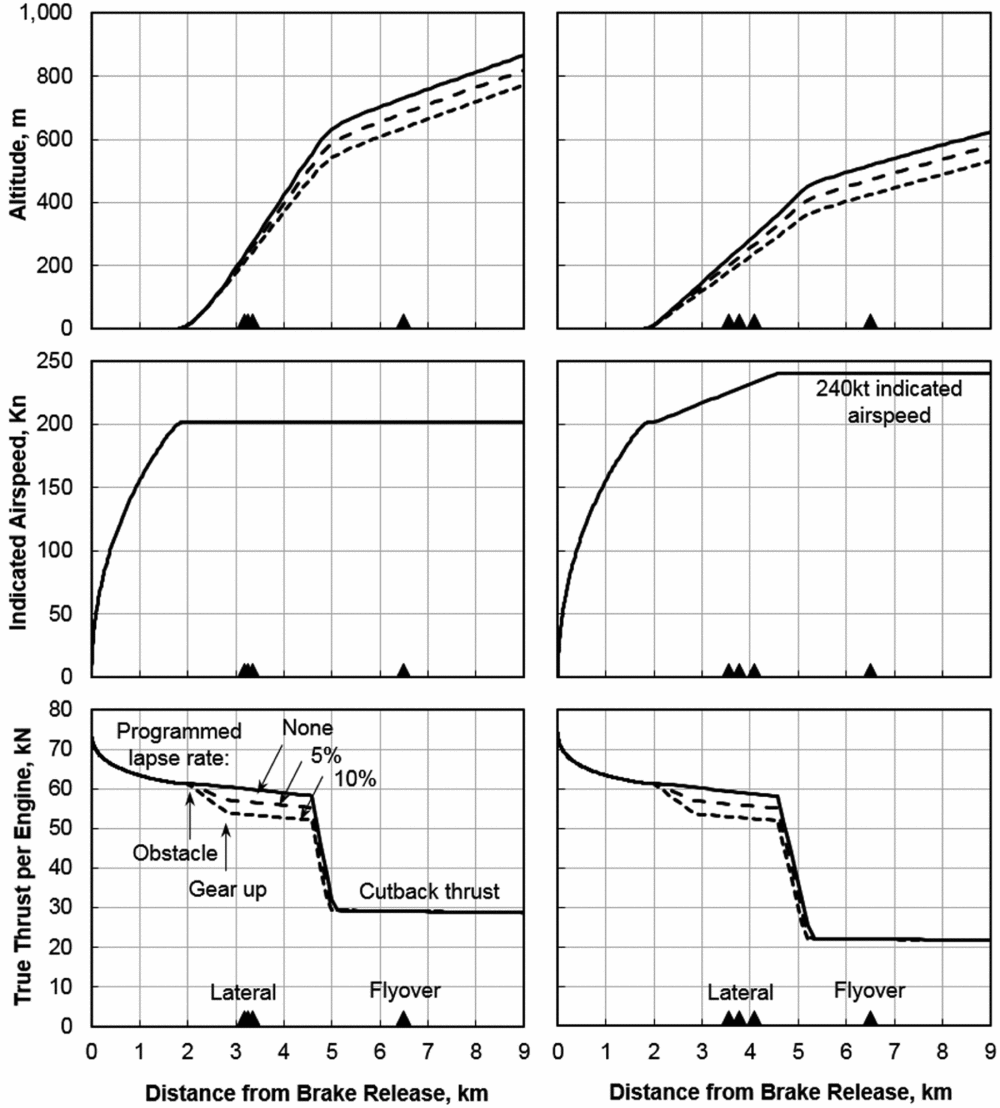
Figure 5. Take-off trajectories with steady climb-out (left) and accelerating climb-out (right), showing influence of programmed thrust lapse rate and noise reduction cutback.
2.1.2.2 Advanced take-off procedures
Noise certification take-offs using programmed thrust lapse rates of 5% and 10% are shown in Fig. 5. Thrust is automatically reduced by digital engine controllers beginning at the runway obstacle and is completed by the start of the second segment climb.
Automatic control of engine thrust has been implemented on several popular civil aircraft, albeit not for noise reduction purposes. Regulations governing automatic take-off thrust control systems were defined under 14 CFR § 25.904 in 1987.
The notion of using programmed thrust lapse as an automatic noise reduction mechanism might be designed as a variable noise reduction system (VNRS) under Ref. 13. Infrequently implemented, a VNRS is a system where noise is abated automatically without flight crew intervention.
Alternately, an examination of the FAA special conditions docket for aircraft having automatic thrust control systems at low altitude is revealing. Digital engine controllers on the Airbus A320 family have an “auto-thrust” feature that automatically increase thrust with no change in power lever position in the event of a stall(14). Also, a special condition(15) has been granted to the Embraer 170/190 family in the event of engine failure, allowing a digital engine controller to automatically increase thrust on the operative engine during a go-around. These examples suggest digitally automated thrust control procedures such as programmed thrust lapse might be implemented for supersonic aircraft on a case-by-case basis using a special conditions exception. In any event, greater clarity may be helpful if automated thrust control is an enabling noise reduction requirement for supersonic transports.
Satisfying minimum climb-gradient requirements may be an important consideration when using a programmed thrust lapse. In the event of an engine failure during climb-out, 14 CFR § 25.121 specifies a minimum climb gradient requirement in the segment defined between the points when gear is retracted and an altitude of 400 ft. This regulation requires the remaining operative engines to be at “the power or thrust available when retraction of the landing gear is begun.” This, by our assumptions, is the maximum take-off thrust prior to the programmed thrust lapse. Thus, an automatic take-off thrust control system would be needed to increase thrust on the remaining engines. Further, time to spool the engines up to maximum power must be considered, and so the amount of programmed thrust lapse could be limited.
Noise certification take-offs using accelerating climbouts are also shown in Fig. 5. There is some flexibility in how an acceleration segment may be performed. Both altitude gain and acceleration in some proportion are possible. In this study, arbitrarily but with some logic, the aircraft simultaneously climbs and accelerates such that an indicated airspeed of 240 Kn is reached at the beginning of the pilot-initiated throttle cutback. This airspeed is slightly less than the maximum 250-Kn limit in the United States and elsewhere. As noted earlier, a high-speed climb-out would require a departure from 3.6.2(d)(1) of Ref. 1, with the justification that it is required to ensure adequate thrust margin and climb performance. In operational practice, high-speed climb-outs are sometimes allowed. Permission may be granted on a case-by-case basis if other aircraft are not in the vicinity. In the future, nearby aircraft may need to give more room to supersonic transports. This practice is already common today, where greater spacing intervals are given to large aircraft due to their wake turbulence.
2.1.2.3 Influence on noise
Advanced take-off procedures have several positive impacts on noise, in addition to reducing lateral noise as noted already. A high-speed climb-out results in less shear between the jet and ambient air, resulting in weaker turbulence and less jet noise. A high-speed climb-out also results in generally lower noise due to shorter event duration. And higher airspeed also creates more lift, allowing a deeper pilot-initiated engine thrust cutback. This last effect is noticeable in Fig. 5. However, either a programmed thrust lapse or an accelerating climbout results in lower climb rates and much lower altitudes over the flyover monitor. The consequence of this is that a programmed thrust lapse tends to lower lateral noise at the expense of flyover noise.
2.1.3 Noise analysis
It is helpful to begin a noise assessment of a conceptual aircraft by considering how loud it can be. Maximum Effective Perceived Noise Levels (EPNLs) for a 55-t (121,000-lb) tri-jet are presented in Table 2 given the language in Annex 16. Maximum lateral, flyover, and approach levels for Chapter 3 are shown. Since supersonic transports are expected to be loudest just after take-off, lateral EPNLs for Chapter 4 and for Chapter 14 are set to the maximum levels permitted. The remaining requirements are split evenly between flyover and approach. Margins naturally would be added to these maximum targets to increase confidence before a product launch decision.
Table 2 Maximum target levels required to meet Chapters 3, 4 and 14 for a 55-t tri-jet, EPNdB

* Maximum permitted lateral noise; remaining requirement split evenly between flyover and approach.
** Chapter 14 requires a margin of not less than 1 EPNdB below Chapter 3 limits at each point.
Noise certification predictions are made using NASA's Aircraft Noise Prediction Program (ANOPP)(Reference Gillian16,Reference Zorumski17) . Engine-state data computed by NPSS are fed into ANOPP as functions of flight speed, altitude and engine power setting. Jet noise, fan noise, fan treatment suppression, core noise and several airframe sources are predicted using the methods of Stone(Reference Stone, Krejsa, Clark and Berton18), General Electric(Reference Kontos, Janardan and Gliebe19,Reference Kontos, Kraft and Gliebe20) . Emmerling(Reference Emmerling, Kazin and Matta21) and Fink(Reference Fink22). respectively, using best practices. Selecting methods for accurate prediction of source noise levels is essential. Each of these methods is considered to be the best available at NASA for system noise prediction(Reference Dahl23). The methods used in this study are developed around semi-empirical mathematical frameworks that follow physics-based aeroacoustic scaling relationships. The frameworks are empirically calibrated with experimental data. Stone's jet noise prediction method, for example, is developed using anechoic free-jet acoustic data from a variety of nozzle test articles. It uses a physics-based, Bayesian-style regression of jet acoustic data that spans various nozzle flow and geometry regimes.
Fan noise is more difficult to predict accurately, since it is quite dependent on the particulars of fan design. Several calibrations of ANOPP's fan noise prediction framework have been made for various families of fans. The General Electric calibration used in this study reflects their experience base with large commercial turbofans in service just prior to 1996. Acoustic liners are assumed present in much of the interior of the inlet and bypass exhaust ducts. An empirical model developed by General Electric is used to predict liner performance. The method predicts liner suppression spectra based on gross duct geometry inputs, and it is also representative of commercial liners in service in the 1990s.
Though fan noise is modelled perhaps with the least certainty, it is less important to model precisely than jet noise, which dominates the noise signature of the aircraft. Further, with engines mounted above the vehicle, inlet-radiated fan noise is very effectively shielded from observers on the ground. Shielding (also referred to as barrier attenuation or insertion loss) is an acoustic diffraction phenomenon where sound waves are attenuated when propagated past an impermeable barrier placed between the noise source and an observer. In this study, a simple empirical diffraction model based on optical diffraction theory is used. The model was originally proposed by Maekawa(Reference Maekawa24) and is reproduced in many foundational acoustic textbooks. Shielding is particularly efficient when the observer is located in the “shadow region” where the noise source is obscured. The delta wing (see Fig. 3) provides excellent shielding of forward-radiated fan inlet noise. All other sources are not shielded. Jet noise is a distributed source generated downstream throughout the axial exhaust plume. Core noise is predominantly aft-radiating and is assumed to radiate through the exhaust. Fan exit noise also escapes through the nozzle but it is attenuated by treatment in the bypass duct.
These noise sources are analytically flown along the trajectories described in the previous section and propagated to noise certification monitors on the ground. The source levels are computed at half-second intervals using engine-state data at the correct flight condition and engine power. This is particularly important in modelling noise during the programmed thrust lapse procedure. Noise propagation effects include spherical spreading, Doppler shift and convective amplification, atmospheric absorption(25), ground reflections(Reference Chien and Soroka26) based on data for grass-covered ground(Reference Embleton, Piercy and Daigle27), and lateral ground attenuation(28). Modelling ground reflections and lateral attenuation properly is important to determine the influence of the programmed thrust lapse procedure on lateral noise. The results of this study are relevant only to a noise certification test environment where terrain is relatively flat, and where ground preparation, atmospheric and weather conditions are strictly prescribed(13). Applying results of this study to more complex surroundings found in operational settings should be done with caution.
Certification noise predictions are shown in Table 3 and are compared to published noise type certificate data of subsonic aircraft in Fig. 6. Predictions are made for steady and accelerating climb-outs and with programmed thrust lapse rates of 5% and 10%. Plotted in Fig. 6 are published data of over 11,000 Chapter 4 aircraft types. The standard take-off (solid symbol) uses no advanced take-off procedures and satisfies the requirements of Annex 16 without departures from 3.6.2. The advanced take-off (open symbol) uses a 10% programmed thrust lapse rate and an accelerating climb-out.
Table 3 Certification noise predictions, EPNdB

* “Trading” is necessary to meet Chapter 3 limits.

Figure 6. EPNL predictions compared to Chapter 4 data, showing influence of advanced take-off procedures.
Lateral EPNL is primarily a function of jet noise, without significant influence from vehicle performance. Lateral noise tends to peak wherever the aircraft reaches an altitude of about 300 m, where ground attenuation effects vanish but before the aircraft gains more altitude. Thus, vehicle aerodynamics play only a small role in lateral noise level.
Approach and flyover EPNLs, however, are strongly influenced by vehicle aerodynamics and performance. Approach noise levels tend to increase if high-lift wing devices do not improve lift adequately. In this study, it was necessary to add drag to the approach aerodynamics of Reference WelgeRef. 11 (perhaps in the form of speed brakes) to limit approach speed to 160 Kn. The simple addition of drag devices to keep approach speed acceptable is undesirable since thrust must increase to overcome drag while holding the 3° glide slope required by 3.6.3(a) of Ref. 1. It is far better, instead, to improve lift, or approach noise will increase. But thin supersonic wings may not be able to accommodate efficient, more complex high-lift devices. Even so, approach noise is predicted to be similar to published data for aircraft in this weight class.
During take-off, the engine power setting for the noise reduction cutback is determined by climb rate, which in turn is also a strong function of vehicle aerodynamics. Although it may appear from these results that flyover margin is sufficient, that margin could erode quickly if levels are underpredicted.
Thus, the approach and flyover EPNLs in this assessment likely have the most uncertainty due to the lack of rigour in our analysis of high lift devices and our assumptions of engine placement and wing shielding. Lateral noise predictions, therefore, are emphasised. Fortunately, advanced take-off procedures and their influence on lateral noise are the focus of this study. A sensitivity study to system uncertainties is planned in a later phase of this work.
Because these results are determined from a variety of largely unknown variables, a Monte Carlo uncertainty analysis is planned and is expected to provide insight into the system model. Normally deterministic, the benchmark noise model will be transformed into a stochastic model by replacing portions of its input data with continuous random values. A vector of input variables representing modelling unknowns will be randomly permuted using probability distributions centred around the model's nominal values. The input variables subject to randomisation will be chosen by a top-down decomposition of the system noise problem. Results of the uncertainty simulation are planned for publication in a later article.
Lateral EPNLs have the narrowest predicted noise margins. Indeed, the lateral EPNL for the standard take-off is not predicted to qualify for Chapter 4. It does, however, qualify for Chapter 3 (despite exceeding the Chapter 3 lateral limit) by virtue of “trading,” a practice once allowed under Chapter 3 certification. Trading allowed applicants to exchange an exceedance at one or two points if the exceedance(s) were offset by a corresponding lower amount at another point or points.
2.1.4 Implications of advanced take-off procedures
Noise at the lateral condition can be made perhaps acceptable for Chapter 4 certification with a programmed thrust lapse or with a thrust lapse combined with an accelerating climbout, albeit with narrow lateral margins. Other source noise reduction technologies mature enough for a near-term supersonic transport could also be used to improve margin. But using a programmed thrust lapse to reduce lateral noise is not without consequences. Lowering thrust adversely affects the aircraft's ability to climb and forces the aircraft to pass over the flyover noise measurement point at a lower altitude than if a standard take-off had been performed. For this reason, the programmed thrust lapse procedure, despite its lateral noise reduction advantage, is in conflict with keeping flyover noise levels low. This can be seen from the noise “footprints” (i.e., isopleths of constant noise level) plotted in Fig. 7. When a programmed thrust lapse is used to reduce lateral noise, footprints extend further downrange into the community. The 81 EPNdB contours shown in the figure are arbitrary. There is no particular significance to showing that level over any other, other than it aptly illustrates the influence of the programmed thrust lapse downrange from an airport.

Figure 7. Take-off EPNL footprints showing influence of programmed thrust lapse: steady climb-out (top), accelerating climb-out (bottom). Plan dimensions in kilometres.
The benefit of an accelerating climb-out is clear. It is helpful in ensuring adequate climb-out thrust margin for supersonic transports, and it has the positive side effect of reducing noise. But given the trade between lateral and flyover noise when a programmed thrust lapse is used, a fair question to ask is whether it should be implemented at all. In most instances, more people live downrange of airports than alongside airport runways. The programmed thrust lapse procedure is likely to subject more people to noise. It also sacrifices altitude or airspeed (or both, depending on how the climb-out is conducted), at a time when they are most welcome. And developing simplifying equivalent procedures for noise certification flight tests seems difficult. The concept exploits the interaction between lateral attenuation – vanishing with increasing altitude – with a reduction in thrust. Mimicking the behaviour of the take-off while accurately representing the complex acoustics of the problem may be challenging.
Yet the issue of satisfying the maximum lateral noise level merits further discussion. One possibility is to simply relax the maximum lateral limit for future supersonic aircraft. Another possibility might be to revive the practice of trading. Since there appears to be margin at the other two conditions (at least in this study), supersonic aircraft might be permitted to exceed the lateral limit by exchanging margin.
3.0 CONCLUSIONS
Analytical models of a near-term supersonic business jet are developed and used to study advanced take-off noise-reduction procedures. Two advanced take-off procedures – accelerating climb-out and programmed thrust lapse – and their apparent necessity for supersonic transports are evaluated in this study. Results show these procedures are helpful in reducing lateral noise but may require departures from normal reference procedures defined by regulations. An accelerating climb-out is shown to reduce both lateral and flyover noise, but a programmed thrust lapse is shown to reduce lateral noise at the expense of flyover noise.
ACKNOWLEDGEMENTS
Thanks to NASA's Commercial Supersonic Technology Project for supporting this study.