1. Introduction
The modern Pacific margin of North America extends for ∼8000 km, from Alaska to Mexico, and is one of the best natural laboratories to study the range of interactions between oceanic and continental plates because of the different tectonic settings that exist along its strike (e.g. Dickinson, Reference Dickinson, Mahlburg Kay, Ramos and Dickinson2009). The Alaskan subduction zone changes southward to the Queen Charlotte transform fault (Fig. 1), which is connected to the south with the Cascadia subduction zone. Further south, subduction is truncated by the San Andreas transform fault and the Gulf of California rift system, which is connected to the Acapulco trench in southern Mexico. Such discontinuities in the lateral extension of the trench are manifest by the development of a disjointed North American volcanic arc consisting of the Aleutian–Alaskan (e.g. Kay & Kay, Reference Kay and Kay1988) and Cascades (e.g. O’Hara et al. Reference O’Hara, Karlstrom and Ramsey2020) arcs, as well as the Trans-Mexican Volcanic Belt (TMVB; Gómez-Tuena et al. Reference Gómez-Tuena, Mori and Straub2018 b; Fig. 1). The complex architecture of the North American Pacific margin is mainly the result of its along-strike variations relative to the convergence vector between the North America and Pacific plates. In effect, the orientation of the latter is highly variable along the North American continental edge (Fig. 1) because of the Pacific–Farallon mid-ocean ridge oblique subduction of the past ∼30 Ma (Dickinson, Reference Dickinson, Mahlburg Kay, Ramos and Dickinson2009; Müller et al. Reference Müller, Zahirovic, Williams, Cannon, Seton, Bower, Tetley, Heine, le Breton, Liu, Russell, Yang, Leonard and Gurnis2019). Considering the likely variability of past convergence vectors (Müller et al. Reference Müller, Zahirovic, Williams, Cannon, Seton, Bower, Tetley, Heine, le Breton, Liu, Russell, Yang, Leonard and Gurnis2019), and that subduction of ocean and aseismic ridges likely occurred repeatedly throughout geological history (Pilger, Reference Pilger1981; Kinoshita, Reference Kinoshita2002; Sisson et al. Reference Sisson, Pavlis, Roeske, Thorkelson, Sisson, Roeske and Pavlis2003; Schoonmaker & Kidd, Reference Schoonmaker and Kidd2006), it seems plausible to assume that many ancient continental margins, including the North American Pacific margin itself, displayed at times a segmented configuration similar to the present one (Fig. 1). However, in most Mesozoic tectonic reconstructions, the Pacific continental edge of North America is envisioned as a continuous convergent margin, along which ongoing subduction occurred for more than ∼150 Ma, between Triassic and Late Cretaceous times (Dickinson & Lawton, Reference Dickinson and Lawton2001; Pindell & Kennan, Reference Pindell, Kennan, James, Lorente and Pindell2009; Riel et al. Reference Riel, Jaillard, Martelat, Guillot and Braun2018). Continuous subduction beneath North America during Mesozoic time should have resulted in the development of a Triassic–Cretaceous arc along its western continental margin. Yet, available geochronological and geochemical data indicate that the central and southern segments of the Mexican Pacific margin were characterized by the absence of an active subduction zone during most of the Triassic period (Kirsch et al. Reference Kirsch, Helbig, Keppie, Murphy, Lee and Solari2014; Centeno-García, Reference Centeno-García2017; Coombs et al. Reference Coombs, Kerr, Pindell, Buchs, Weber, Solari, Martens and Molina Garza2020). Moreover, Early and Middle Jurassic igneous rocks in southern and central Mexico (Barboza-Gudiño et al. Reference Barboza-Gudiño, Zavala-Monsiváis, Castellanos-Rodríguez, Jaime-Rodríguez and Almaraz-Martínez2021) have been tentatively interpreted either as the vestiges of a continental arc (C. Bartolini, unpub. Ph.D. thesis, Univ. Texas at El Paso, 1998; Lawton & Molina Garza, Reference Lawton and Molina Garza2014; Barboza-Gudiño et al. Reference Barboza-Gudiño, Zavala-Monsiváis, Castellanos-Rodríguez, Jaime-Rodríguez and Almaraz-Martínez2021) or a syn-rift igneous province associated with Pangaea break-up (Martini & Ortega-Gutiérrez, Reference Martini and Ortega-Gutiérrez2018; Cavazos-Tovar et al. Reference Cavazos-Tovar, Gómez-Tuena and Parolari2020). A rift setting for these Jurassic rocks would imply that, contrary to what is predicted by most previous reconstructions, there would be no solid evidence of subduction beneath the central and southern segments of the Mexican Pacific margin during a large part of Triassic and Jurassic time. Based on this premise, clarifying the genesis of Jurassic igneous rocks in southern and central Mexico is key to reconstructing the evolution of the southern North American Pacific margin and understanding the processes that shaped it during early Mesozoic time.
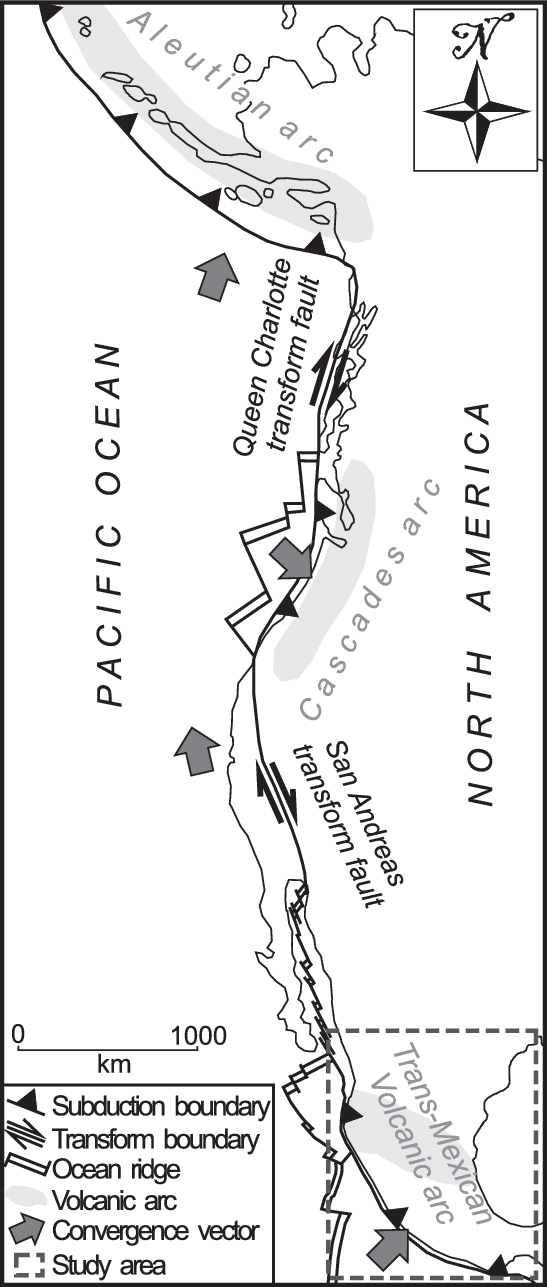
Fig. 1. Modern geotectonic configuration of the North America Pacific margins.
This work reviews the available geochemical data from Early–Middle Jurassic igneous and meta-igneous rocks exposed in southern and central Mexico, to discuss their petrogenesis and test the proposed arc and rift scenarios. After this assessment, we present a new tectonic model describing the evolution of the central and southern segments of the Mexican margin during Early–Middle Jurassic time, and further propose a new hypothesis regarding the onset of subduction in southern Mexico at the end of Jurassic time.
2. Geological background
2.a. Middle–Late Triassic time: the onset of Pangaea break-up
The fragmentation of Pangaea started by Middle–Late Triassic time with the development of a NE–SW-trending rift belt that roughly follows the Ouachita–Marathon–Sonora, Alleghanian and Variscan orogenic belts that formed during Pangaea amalgamation (Bird & Burke, Reference Bird and Burke2006; Fig. 2a). During this early stage of Pangaea break-up, rift basins were infilled by voluminous, Middle–Upper Triassic fluvial to shallow-marine successions that are locally interbedded with and cut by syn-rift volcanic and intrusive rocks (Klitgord et al. Reference Klitgord, Hutchinson, Schouten, Sheridan and Grow1988; Le Roy & Piqué, Reference Le Roy and Piqué2001; Schlische, Reference Schlische, LeTourneau and Olsen2003; Martin-Rojas et al. Reference Martin-Rojas, Somma, Delgado, Estévez, Iannace, Perrone and Zamparelli2009; Frederick et al. Reference Frederick, Blum, Snedden and Fillon2020; Erlich & Pindell, Reference Erlich, Pindell, Davison, Hull and Pindell2021; Fig. 2b). At the same time, the development of continental arc and back-arc assemblages in the Colombian and Venezuelan Andes (Correa-Martínez et al. Reference Correa-Martínez, Rodríguez, Arango, Zapata and Bermúdez2016; van der Lelij et al. Reference van der Lelij, Spikings, Ulianov, Chiaradia and Mora2016; Spikings & Paul, Reference Spikings, Paul, Gómez and Pinilla-Pachon2019), as well as in southwestern USA and northwestern Mexico (Riggs et al. Reference Riggs, Reynolds, Lindner, Howell, Barth, Parker and Walker2013, Reference Riggs, Oberling, Howell, Parker, Barth, Cecil and Martz2016; Lawton et al. Reference Lawton, Urueña, Solari, Terrazas, Juárez-Arriaga, Ortega-Obregón, Ingersoll, Lawton and Graham2018) indicates that subduction was taking place at least beneath some parts of the western Pangaean margin (Fig. 2b). Middle–Upper Triassic arc successions are, however, lacking in central and southern Mexico. Instead, this sector of the Pangaean margin was the site of deposition of turbidite successions (Zacatecas, Taray, Charcas and La Ballena formations, and the Arteaga and El Chilar complexes; Centeno-García, Reference Centeno-García, Anderson, Nourse, McKee and Steiner2005; Ortega-Flores et al. Reference Ortega-Flores, Solari and de Jesús Escalona-Alcázar2016, Reference Ortega-Flores, Solari, Martini, Ortega-Obregón, Martens and Molina-Garza2020, Reference Ortega-Flores, Solari and Martini2021), which have been interpreted as the stratigraphic record of submarine fans (i.e. the Potosí and Tolimán) that were deposited along the continental margin of western equatorial Pangaea and the adjacent Farallon oceanic plate (Silva-Romo et al. Reference Silva-Romo, Arellano-Gil, Mendoza-Rosales and Nieto-Obregón2000, Reference Silva-Romo, Mendoza-Rosales, Campos-Madrigal, Centeno-García and Peralta-Salazar2015; Barboza-Gudiño et al. Reference Barboza-Gudiño, Zavala-Monsiváis, Venegas-Rodríguez and Barajas-Nigoche2010; Ortega-Flores et al. Reference Ortega-Flores, Solari and Martini2021; Fig. 2b). Previous studies pointed out that the youngest detrital zircon U–Pb age populations (∼270–245 Ma) in these Triassic successions are considerably older (∼30–10 m.y.) than the depositional age of the hosting deposits (Barboza-Gudiño et al. Reference Barboza-Gudiño, Zavala-Monsiváis, Venegas-Rodríguez and Barajas-Nigoche2010; Ortega-Flores et al. Reference Ortega-Flores, Solari, Lawton and Ortega-Obregón2014). Based on this evidence, Centeno-García (Reference Centeno-García2017) suggested that the Triassic submarine fan successions were emplaced in the absence of an active magmatic arc. Even though a few subordinate detrital zircon grains of Late Triassic age have been documented in some samples from the Upper Triassic metasedimentary succession (e.g. Barboza-Gudiño et al. Reference Barboza-Gudiño, Zavala-Monsiváis, Venegas-Rodríguez and Barajas-Nigoche2010; Ortega-Flores et al. Reference Ortega-Flores, Solari and Martini2021), the lack of widespread Late Triassic igneous rock exposures likely indicates that these zircons are far-travelled grains derived from distant sources in northwestern Mexico and northwestern South America. Likewise, the minor occurrence of Late Triassic zircon grains in modern fluvial and littoral deposits draining a large sector of southern Mexico (Cavazos-Tovar et al. Reference Cavazos-Tovar, Gómez-Tuena and Parolari2020) documents a clear gap in magmatism and zircon production, and supports the lack of a Late Triassic arc, at least along this sector of the western Pangaean margin.
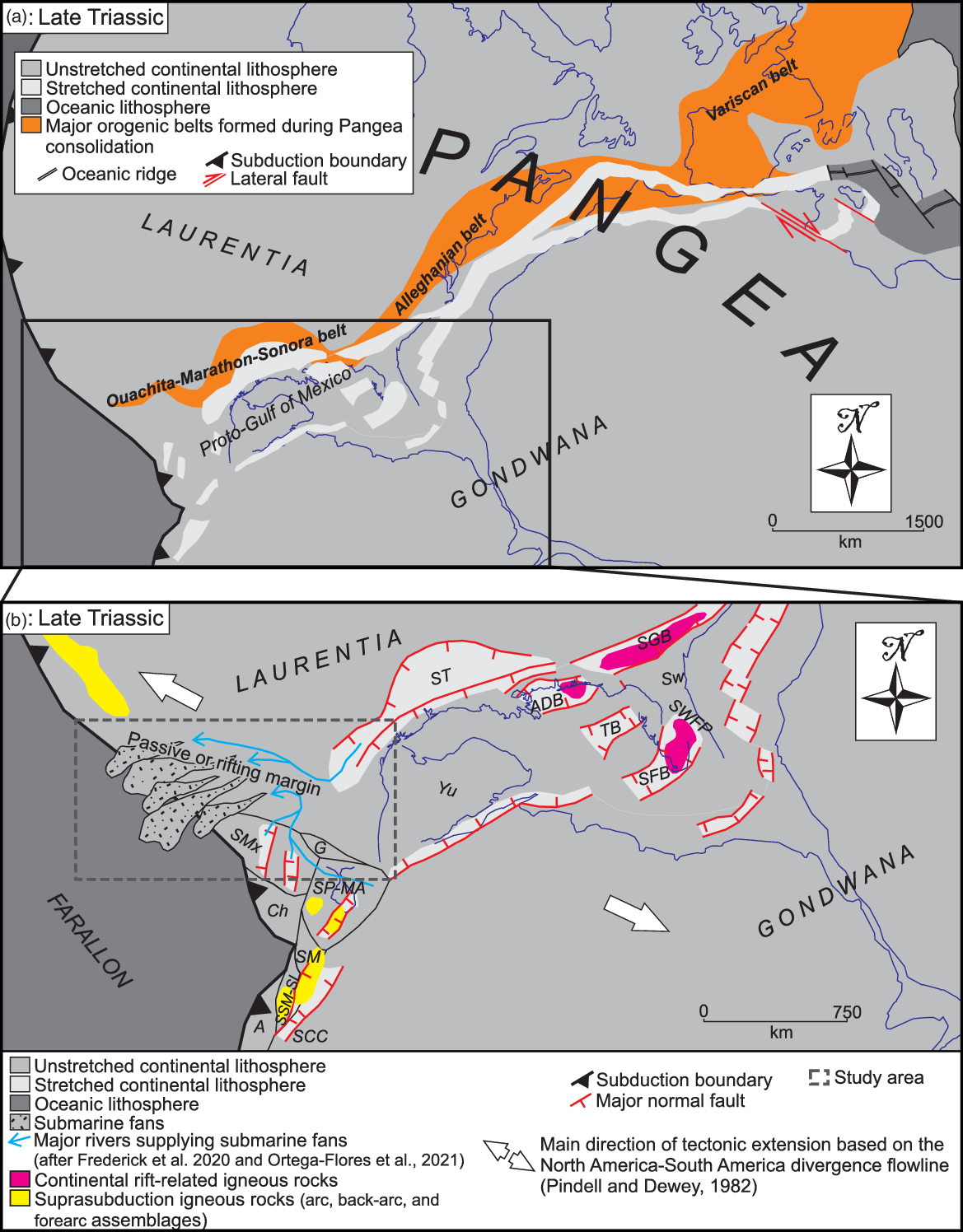
Fig. 2. (a) Reconstruction of the supercontinent Pangaea in Late Triassic time (∼230–210 Ma). The reconstruction is obtained by integrating previous reconstructions and data published by Dickinson & Lawton (Reference Dickinson and Lawton2001), Le Roy & Piqué (Reference Le Roy and Piqué2001), Schettino & Turco, (Reference Schettino and Turco2009), Labails et al. (Reference Labails, Olivet, Aslanian and Roest2010) and Pindell et al. (Reference Pindell, Villagómez, Molina-Garza, Graham, Weber, Davison, Hull and Pindell2021). (b) Geotectonic reconstruction of the western equatorial margin of Pangaea in Late Triassic time (∼230–210 Ma). The reconstruction shows the areas that experienced continental attenuation and the distribution of subduction-related and continental rift-related igneous rocks. The reconstruction of the Florida and Yucatán areas is based on data presented in Erlich & Pindell (Reference Erlich, Pindell, Davison, Hull and Pindell2021) and Frederick et al. (Reference Frederick, Blum, Snedden and Fillon2020). The Mexican sector of the Pangaean margin is reconstructed according to data in Pindell et al. (Reference Pindell, Villagómez, Molina-Garza, Graham, Weber, Davison, Hull and Pindell2021) and Ortega-Flores et al. (Reference Ortega-Flores, Solari and Martini2021). The Colombian sector of the Pangaean margin is according to Bayona et al. (Reference Bayona, Jiménez, Silva, Cardona, Montes, Roncancio and Cordani2010), van der Lelij et al. (Reference van der Lelij, Spikings, Ulianov, Chiaradia and Mora2016), Bayona et al. (Reference Bayona, Bustamante, Nova, Salazar-Franco, Gómez and Pinilla-Pachon2020) and Spikings & Paul (Reference Spikings, Paul, Gómez and Pinilla-Pachon2019). A – Antioquia block; ADB – Apalachicola–Desoto Canyon Basin; Ch – Chortis block; G – Guajira block; SP-MA – Merida Andes–Sierra de Perijá block; SCC – Southern Central Cordillera of Colombia; SFB – South Florida Basin; SGB – South Georgia Basin; SM – Santander Massif block; SMx – South Mexico block; SSM-SL – Sierra de Santa Marta–San Lucas block; ST – South Texas Basin; Sw – Swannee terrane; SWFP – Southwest Florida Mesozoic volcanic province; TB – Tampa Basin; Yu: Yucatán block.
Based on the increasing evidence of the lack of a magmatic arc, Centeno-García (Reference Centeno-García2017) proposed that the central and southern Mexican sector was a passive or rifted margin during Late Triassic time (Fig. 2b). The juxtaposition of this Late Triassic passive or rifted margin with the adjacent convergent boundaries to the north and south requires the existence of major transform faults dissecting the western equatorial margin of Pangaea. However, none of these Late Triassic faults have been documented and no indirect evidence has yet been identified. Moreover, the conjugate for the Mexican rifted margin has not been found, making the passive-rifting margin scenario speculative. In contrast, Vega-Granillo et al. (Reference Vega-Granillo, Sarmiento-Villagrana, Vidal-Solano, Araux-Sánchez and Bourjac-de-Anda2020) recently proposed a model in which the Upper Triassic metasedimentary successions of southern and central Mexico were developed in a more complex tectonic setting, in which an ENE-elongated continental rift basin, roughly separating southern and northern Mexico, interacted with a major subduction boundary that developed along the western margin of Pangaea. However, this scenario requires the development of a Late Triassic extensional arc in southern and central Mexico and, thus, is not supported by available geochronological data that document a gap in the magmatic activity during Late Triassic time. Between Late Triassic and earliest Jurassic times, the submarine fan deposits were folded, sheared and tectonically transported to the NE (Ortega-Flores et al. Reference Ortega-Flores, Solari and de Jesús Escalona-Alcázar2016; Vásquez-Serrano et al. Reference Vásquez-Serrano, Nieto-Samaniego, Alaniz-Álvarez and Rangel-Granados2019; Fig. 3). Shortening of the Triassic succession produced an orogenic wedge (Vásquez-Serrano et al. Reference Vásquez-Serrano, Nieto-Samaniego, Alaniz-Álvarez and Rangel-Granados2019) that presently extends from the trench into the continental interior, with the easternmost exposures almost bounding the western Gulf of Mexico (GM). The total thickness of this orogenic wedge is unknown, although a minimum thickness of ∼7 km is depicted in structural sections by some authors (e.g. Vásquez-Serrano et al. Reference Vásquez-Serrano, Nieto-Samaniego, Alaniz-Álvarez and Rangel-Granados2019). The dynamics and the driving forces of this deformation event are not yet understood. Some authors speculated that shortening of the Upper Triassic submarine deposits was linked to the onset of eastward subduction beneath the North American Pacific margin, or to the westward subduction beneath an exotic Pacific island arc that was subsequently accreted to the North American continental margin (Anderson et al. Reference Anderson, McKee and Jones1990; Centeno-García & Silva-Romo, Reference Centeno-García and Silva-Romo1997; Centeno-García, Reference Centeno-García2017; Vásquez-Serrano et al. Reference Vásquez-Serrano, Nieto-Samaniego, Alaniz-Álvarez and Rangel-Granados2019). A few mafic rocks interpreted as exotic blocks within the sheared and folded submarine fan deposits display geochemical and isotopic signatures of island-arc tholeiites and a high pressure – low temperature paragenesis (5–7 kbar and 200–330 °C, respectively; Talavera-Mendoza, Reference Talavera-Mendoza2000). However, as the age of these metamorphic mafic rocks is unknown, the hypothesis that they are contemporaneous to the Upper Triassic successions of Mexico remains unconfirmed.
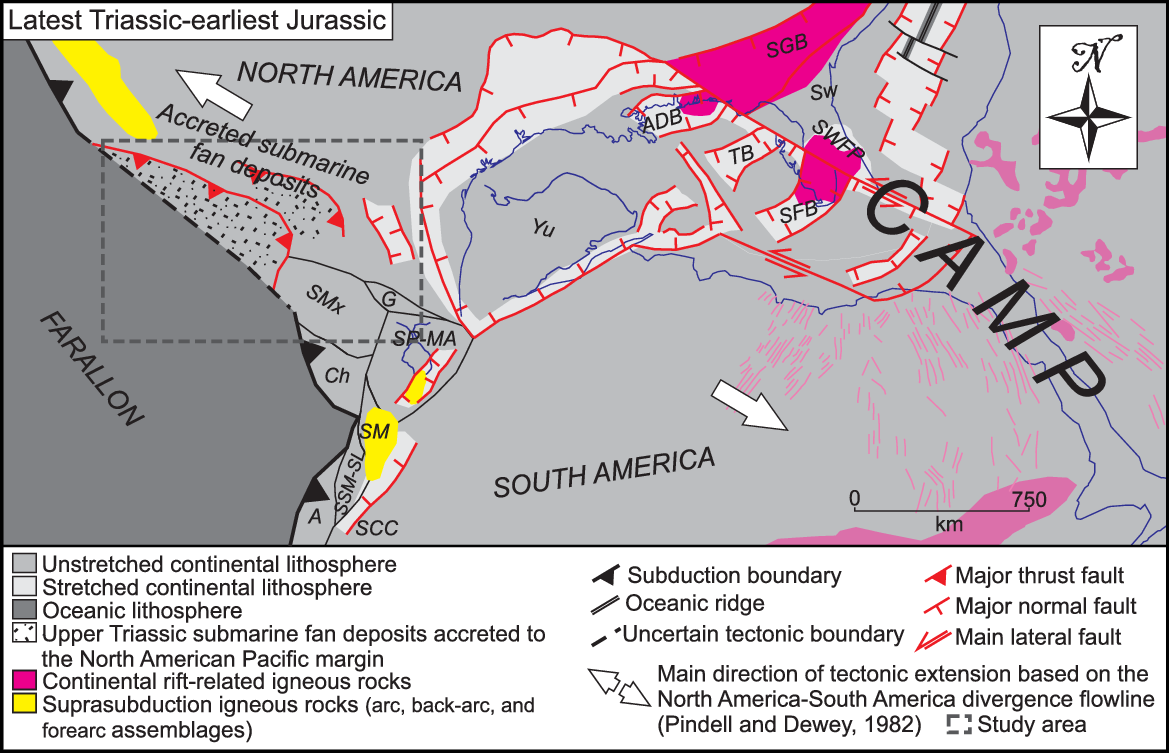
Fig. 3. Geotectonic reconstruction of the North America – South America divergent boundary between latest Triassic and earliest Jurassic time (∼202–200 Ma; based on Boschman et al. Reference Boschman, van Hinsbergen, Torsvik, Spakman and Pindell2014; Marzoli et al. Reference Marzoli, Callegaro, dal Corso, Davies, Chiaradia, Youbi, Bertrand, Reisberg, Merle, Jourdan and Tanner2018; Bayona et al. Reference Bayona, Bustamante, Nova, Salazar-Franco, Gómez and Pinilla-Pachon2020; Erlich & Pindell, Reference Erlich, Pindell, Davison, Hull and Pindell2021 and Pindell et al. Reference Pindell, Villagómez, Molina-Garza, Graham, Weber, Davison, Hull and Pindell2021). The reconstruction shows the areas that experienced continental attenuation and the major normal to lateral faults that accommodated divergence between North and South America. It also shows the extension of the Central Atlantic Magmatic Province (CAMP) associated with the continental rifting, as well as the distribution of the subduction-related magmatism along the Pacific margins of the Americas. A – Antioquia block; ADB – Apalachicola–Desoto Canyon Basin; Ch – Chortis block; G – Guajira block; SP-MA – Merida Andes–Sierra de Perijá block; SCC – Southern Central Cordillera of Colombia; SFB – South Florida Basin; SGB – South Georgia Basin; SM – Santander Massif block; SMx – South Mexico block; SSM-SL – Sierra de Santa Marta–San Lucas block; ST – South Texas Basin; Sw – Swannee terrane; SWFP – Southwest Florida Mesozoic volcanic province; TB – Tampa Basin; Yu – Yucatán block.
2.b. Latest Triassic – earliest Jurassic time: the emplacement of the Central Atlantic Magmatic Province and opening of the Atlantic Ocean
The Triassic–Jurassic boundary is marked by the formation of the first ocean ridge in the proto-Atlantic, between Morocco and Nova Scotia (Sahabi et al. Reference Sahabi, Aslanian and Olivet2004; Schettino & Turco, Reference Schettino and Turco2009; Labails et al. Reference Labails, Olivet, Aslanian and Roest2010; Fig. 3). This magmatism heralded the development of the Central Atlantic Magmatic Province (CAMP; Fig. 3), the most extensive magmatic province in Earth’s history (Marzen et al. Reference Marzen, Shillington, Lizarralde, Knapp, Heffner, Davis and Harder2020), the vestiges of which are presently exposed along eastern North and South America, western Africa and southwestern Europe (e.g. Marzoli et al. Reference Marzoli, Callegaro, dal Corso, Davies, Chiaradia, Youbi, Bertrand, Reisberg, Merle, Jourdan and Tanner2018). The CAMP is dominated by basaltic rocks with ages in the range of ∼202 to 192 Ma (primarily 40Ar/39Ar in plagioclase), with a peak at ∼201 Ma (Marzoli et al. Reference Marzoli, Callegaro, dal Corso, Davies, Chiaradia, Youbi, Bertrand, Reisberg, Merle, Jourdan and Tanner2018). Igneous rocks of the CAMP were originally considered the magmatic manifestation of a deep-mantle plume that potentially contributed to Pangaea break-up (Morgan, Reference Morgan1983; Hill, Reference Hill1991). However, it has been increasingly recognized that the genesis of the CAMP is more likely related to decompression melting of shallow upper mantle during Pangaea break-up due to the moderate rise of mantle temperature that resulted from long-term heat incubation underneath the Pangaea supercontinent (Hole, Reference Hole2015; Marzen et al. Reference Marzen, Shillington, Lizarralde, Knapp, Heffner, Davis and Harder2020), possibly related to the mantle return flow generated by the subduction of cold oceanic plates along the supercontinent’s edges (Heron, Reference Heron, Wilson, Houseman, McCaffrey, Doré and Buiter2019). The formation of such a huge thermal anomaly (the African superplume; Zhong et al. Reference Zhong, Zhang, Li and Roberts2007) likely represented a response to the development and establishment of the present-day degree-2 mantle convection during the formation of the supercontinent, which in turn may have exerted a significant control on the development and migration of Cordilleran arcs during the last ∼180 Ma (Spencer et al. Reference Spencer, Murphy, Hoiland, Johnston, Mitchell and Collins2019).
2.c. Early–Middle Jurassic time: the Nazas igneous province
The progressive break-up of Pangaea between ∼195 and ∼168 Ma was accommodated by normal to lateral faults with different orientations, mostly following previous zones of weakness between terranes (e.g. Erlich & Pindell, Reference Erlich, Pindell, Davison, Hull and Pindell2021; Fig. 4). Based on a multi-stage, global plate-reconstruction model of western equatorial Pangaea, Pindell et al. (Reference Pindell, Weber, Hale-Erlich, Cossey, Bitter, Molina Garza, Graham, Erlich, Martens and Molina-Garza2020) estimated continental extension in the order of 600 km and 400 km in the western and eastern proto-GM, respectively. Crustal thinning during this time interval favoured the emplacement of syn-rift basaltic, rhyolitic and minor andesitic lava flows, dykes and sills in the South Georgia rift basin (Heatherington & Mueller, Reference Heatherington and Mueller1999; Heffner et al. Reference Heffner, Knapp, Akintunde and Knapp2012), the Southwest Florida Mesozoic volcanic province (Heatherington & Mueller, Reference Heatherington and Mueller1991, Reference Heatherington and Mueller2003) and the Apalachicola and South Florida basins (Erlich & Pindell, Reference Erlich, Pindell, Davison, Hull and Pindell2021; Fig. 4). To the west, along the northern and southern margins of the Yucatán block, Early–Middle Jurassic syn-rift igneous rocks have not been observed at the surface or reached by wells. The lack of igneous rocks has motivated many authors to suggest that extension along the northern and southern margins of the Yucatán block was essentially amagmatic (e.g. Frederick et al. Reference Frederick, Blum, Snedden and Fillon2020). However, magnetic and gravity anomalies suggest the existence of a deeply buried volcanic rifted margin in southern Texas (Mickus et al. Reference Mickus, Stern, Keller and Anthony2009; Maus et al. Reference Maus, Barckhausen, Berkenbosch, Bournas, Brozena, Childers, Dostaler, Fairhead, Finn, von Frese, Gaina, Golynsky, Kucks, Lühr, Milligan, Mogren, Müller, Olesen, Pilkington, Saltus, Schreckenberger, Thébault and Tontini2009), but the age of these hypothesized volcanic rocks is unknown.

Fig. 4. Geotectonic reconstruction of the North America – South America divergent boundary during Early–Middle Jurassic time (∼190–170 Ma; based on Boschman et al. Reference Boschman, van Hinsbergen, Torsvik, Spakman and Pindell2014; Bayona et al. Reference Bayona, Bustamante, Nova, Salazar-Franco, Gómez and Pinilla-Pachon2020; Erlich & Pindell, Reference Erlich, Pindell, Davison, Hull and Pindell2021 and Pindell et al. Reference Pindell, Villagómez, Molina-Garza, Graham, Weber, Davison, Hull and Pindell2021). The reconstruction shows the areas that experienced continental attenuation and the distribution of subduction-related igneous rocks (including the Nazas igneous province), as well as of the continental rift-related igneous rocks. A – Antioquia block; ADB – Apalachicola–Desoto Canyon Basin; Ch – Chortis block; G – Guajira block; SP-MA – Merida Andes–Sierra de Perijá block; SCC – Southern Central Cordillera of Colombia; SFB – South Florida Basin; SGB – South Georgia Basin; SM – Santander Massif block; SMx – South Mexico block; SSM-SL – Sierra de Santa Marta–San Lucas block; ST – South Texas Basin; Sw – Swannee terrane; SWFP – Southwest Florida Mesozoic volcanic province; TB – Tampa Basin; Yu – Yucatán block.
Lower–Middle Jurassic arc and back-arc assemblages are distributed in the Colombian, Venezuelan and Peruvian Andes (van der Lelij et al. Reference van der Lelij, Spikings, Ulianov, Chiaradia and Mora2016; Quandt et al. Reference Quandt, Trumbull, Altenberger, Cardona, Romer, Bayona, Ducea, Valencia, Vásquez, Cortes and Guzman2018; Bayona et al. Reference Bayona, Bustamante, Nova, Salazar-Franco, Gómez and Pinilla-Pachon2020; López-Isaza & Zuluaga, Reference López-Isaza, Zuluaga, Gómez and Pinilla-Pachon2020), as well as in North America across the length of southern California and western Nevada, continuing southwestward into Arizona and northwestern Mexico (Busby-Spera, Reference Busby-Spera1988; Tosdal et al. Reference Tosdal, Haxel and Wright1989; Busby-Spera et al. Reference Busby-Spera, Martinson, Riggs, Schermer, Harwood and Miller1990; Haxel et al. Reference Haxel, Wright, Riggs, Tosdal, May, Anderson, Nourse, McKee and Steiner2005; González-León et al. Reference González-León, Vázquez-Salazar, Navarro, Solari, Nourse, del Rio-Salas, Lozano-Santacruz, Arvizu and Valenzuela Chacón2021; Fig. 4). These assemblages indicate that, during Early and Middle Jurassic time, subduction persisted at least beneath some sectors of the Pacific margin of South and North America. In central and southern Mexico, scattered exposures of Lower–Middle Jurassic volcano-sedimentary successions and rare subvolcanic and intrusive to meta-intrusive rocks are presently distributed between Torreón, Acapulco and Tuxtla Gutiérrez (C. Bartolini, unpub. Ph.D. thesis, Univ. Texas at El Paso, 1998; Campa-Uranga et al. Reference Campa-Uranga, García Díaz and Iriondo2004; Godínez-Urban et al. Reference Godínez-Urban, Lawton, Molina Garza, Iriondo, Weber and López-Martínez2011; Helbig et al. Reference Helbig, Keppie, Murphy and Solari2012; Zavala-Monsiváis et al. Reference Zavala-Monsiváis, Barboza-Gudiño, Velasco-Tapia and García-Arreola2012; Lawton & Molina Garza, Reference Lawton and Molina Garza2014; Barboza-Gudiño et al. Reference Barboza-Gudiño, Zavala-Monsiváis, Castellanos-Rodríguez, Jaime-Rodríguez and Almaraz-Martínez2021; Fig. 5). The volcano-sedimentary successions are dominantly composed of fluvial deposits alternated with felsic pyroclastic rocks and subordinate mafic to intermediate lava flows (C. Bartolini, unpub. Ph.D. thesis, Univ. Texas at El Paso, 1998; Lawton & Molina Garza, Reference Lawton and Molina Garza2014). Subvolcanic and (meta)intrusive bodies are dominantly granitic in composition (López Infanzón, Reference López Infanzón1986; Elías Herrera & Sánchez Zavala, Reference Elías Herrera and Sánchez Zavala1990; Pérez-Gutiérrez et al. Reference Pérez-Gutiérrez, Solari, Gómez-Tuena and Martens2009; Helbig et al. Reference Helbig, Keppie, Murphy and Solari2012; Pompa-Mera et al. Reference Pompa-Mera, Schaaf, Hernández-Treviño, Weber, Solís-Pichardo, Villanueva-Lascurain and Layer2013). Isotopic ages previously reported for these igneous rocks span from ∼230 to ∼70 Ma (Pb-α, Rb–Sr, K–Ar and Ar–Ar; Bartolini et al. Reference Bartolini, Lang, Spell, Bartolini, Buffler and Blickwede2003 and references therein). However, many of these ages contradict solid stratigraphic relationships, suggesting that, after magmatic emplacement, these rocks experienced a complex thermal history (e.g. Zavala-Monsiváis et al. Reference Zavala-Monsiváis, Barboza-Gudiño, Velasco-Tapia and García-Arreola2012). Based on zircon U–Pb ages, almost all authors agree in bracketing the crystallization age of these rocks between ∼191 and ∼165 Ma (Fig. 5 and references therein). However, U–Pb ages on detrital zircon grains from Jurassic fluvial deposits show that the magmatic activity in central and southern Mexico likely spanned ∼35 m.y., from ∼200 to ∼165 Ma (e.g. Lawton & Molina-Garza, Reference Lawton and Molina Garza2014).

Fig. 5. Schematic map showing the distribution of igneous and meta-igneous rocks of Early and Middle Jurassic age in central and southern Mexico. No systematic geochemical variations are observed among data from different localities (see online Supplementary Material Fig. S1: chemical variation of NIP rocks).
These Early and Middle Jurassic igneous rocks exposed in central and southern Mexico are part of the Nazas igneous province (NIP). Most authors (C. Bartolini, unpub. Ph.D. thesis, Univ. Texas at El Paso, 1998; Lawton & Molina-Garza, Reference Lawton and Molina Garza2014; Barboza-Gudiño et al. Reference Barboza-Gudiño, Zavala-Monsiváis, Castellanos-Rodríguez, Jaime-Rodríguez and Almaraz-Martínez2021) interpreted those rocks as the vestiges of a continental arc (hereafter referred to as the Nazas Arc), which is inferred to be the connection between the Cordilleran arc in the southwestern USA and the continental arc of South America (Fig. 4). Based on the abundance of epiclastic deposits interbedded with volcanic rocks and the evidence of progressive exhumation of basement rocks (Rubio-Cisneros & Lawton, Reference Rubio-Cisneros and Lawton2011; Lawton & Molina Garza, Reference Lawton and Molina Garza2014), the Nazas Arc has been interpreted as a continental extensional arc. In the last few years, however, Martini & Ortega-Gutiérrez (Reference Martini and Ortega-Gutiérrez2018) and Cavazos-Tovar et al. (Reference Cavazos-Tovar, Gómez-Tuena and Parolari2020) suggested that the NIP is likely a syn-rift magmatic province that was formed as a result of crustal thinning associated with Pangaea break-up, with negligible subduction influence. This alternative scenario is based on the observation that, at least in central and southern Mexico, these Early and Middle Jurassic igneous rocks were emplaced in continental extensional to transtensional rift basins, the kinematics of which is closely linked to the NW–SE divergence between North and South America and is incompatible with a SE convergence vector along the Pacific margin of North America (Martini & Ortega-Gutiérrez, Reference Martini and Ortega-Gutiérrez2018). Moreover, Cavazos-Tovar et al. (Reference Cavazos-Tovar, Gómez-Tuena and Parolari2020) showed that the overall negative εHf values and the trace-element systematics of Jurassic zircon grains in modern fluvial and littoral deposits associated with the NIP rocks suggest melting of a metasedimentary crustal source in the genesis of these Early–Middle Jurassic magmas. At present, an exhaustive petrogenetic study of the NIP has not been completed; therefore, its origin remains controversial and the timing and mechanism of subduction initiation along the continental margin of Mexico are still under debate.
3. Methods: data treatment
In this work, we present a compilation (121 samples) of whole-rock geochemical data available from the literature for Early and Middle Jurassic igneous and meta-igneous rocks exposed in central and southern Mexico (online Supplementary Material Table S1). We selected only those samples for which the age and stratigraphic position are well known. Accordingly, we do not consider samples from igneous and meta-igneous exposures with a stratigraphic position that has been tentatively defined by some authors, successively challenged by others and presently is not yet exhaustively resolved. We also exclude from our database those samples from volcanic and metavolcanic layers for which the age has been inferred based on a poorly defined maximum depositional age (defined by fewer than three grains with concordant ages overlapping at 2σ) of the interbedded clastic deposits.
Although the amount of data published by previous authors is significant, deciphering the origin of the Early–Middle Jurassic magmatism in Mexico is challenging, primarily owing to the moderate to pervasive weathering of the igneous and meta-igneous rocks (e.g. Barboza-Gudiño et al. Reference Barboza-Gudiño, Zavala-Monsiváis, Castellanos-Rodríguez, Jaime-Rodríguez and Almaraz-Martínez2021). In fact, most of the compiled chemical analyses display high loss on ignition values (LOI >3 wt %; online Supplementary Material Table S1 and Fig. 6). In addition, primary mineral phases are largely replaced by secondary clay minerals, oxides and hydroxides (Fastovsky et al. Reference Fastovsky, Hermes, Strater, Bowring, Clark, Montellano, Hernandez, Anderson, Nourse, McKee and Steiner2005; Barboza-Gudiño et al. Reference Barboza-Gudiño, Zavala-Monsiváis, Venegas-Rodríguez and Barajas-Nigoche2010; Godínez-Urban et al. Reference Godínez-Urban, Lawton, Molina Garza, Iriondo, Weber and López-Martínez2011; Barboza-Gudiño et al. Reference Barboza-Gudiño, Zavala-Monsiváis, Castellanos-Rodríguez, Jaime-Rodríguez and Almaraz-Martínez2021). To minimize the effect of weathering on the petrogenetic interpretation, we filtered the available data by rejecting analyses characterized by high LOI values (>3 wt %) and by using a combination of weathering-related parameters that aim to increase the reliability of the selected analysis. Specifically, we consider two different weathering indexes: the Chemical Index of Alteration (CIA; Nesbitt & Young, Reference Nesbitt and Young1982) and the Weathering (W) index (Ohta & Arai, Reference Ohta and Arai2007). The CIA is expressed by the ratio of immobile (i.e. Al) to major mobile elements (i.e. Na, Ca and K). Accordingly, less weathered rocks show low CIA values (c. 40–50), and higher values (up to 100) are related mainly to the formation of minerals like kaolinite and sericite at the expense of feldspars, as a result of pervasive and/or protracted weathering (Perri, Reference Perri2020). The W index is a geochemical index built on a statistical analysis of major-element behaviour during weathering (Ohta & Arai, Reference Ohta and Arai2007). High values of the W index indicate moderate to pervasively weathered samples, whereas values in the range of 10–20 are common in fresh rocks (Perri, Reference Perri2020). On a global set of data, these indices display a well-developed positive correlation (Perri, Reference Perri2020) and are thus suitable to be used as complementary parameters to evaluate the weathering extent of the selected rock samples. Applied to our data compilation, the CIA and W indexes show that ∼20 % of the geochemical analyses available were performed on moderately to pervasively weathered samples, the composition of which is the result of extensive mobilization of soluble elements (Fig. 6). In this work, these samples (CIA >80; W index >80) were not considered as representative of the original magmatic compositions and discarded.

Fig. 6. Loss on ignition (LOI) and two different weathering indexes of the compiled chemical analysis (see online Supplementary Material Table S1 for data sources). (a) A conspicuous amount of the samples show high values of LOI and (b) moderate to high weathering conditions (alteration fields Perri, Reference Perri2020). Small blue crosses in (b) represent the selected samples once the filter is applied (see Section 3 for details). CIA – Chemical Index of Alteration (Nesbitt & Young, Reference Nesbitt and Young1982); CIA = Al2O3/(Al2O3 + K2O + Na2O + CaO) × 100 (molar amounts); the equations and the steps used to calculate the W index are listed in Ohta & Arai (Reference Ohta and Arai2007).
A critical issue in interpreting the genesis of Early–Middle Jurassic igneous and meta-igneous rocks from central and southern Mexico is that most of the studied intermediate and mafic rocks display high LOI (Fig. 6a). Accordingly, in our filtered selection (57 samples), the composition of these rocks varies from rhyolite to dacite (Fig. 7a, b). However, as the number of samples that were originally classified as mafic represents only 4 % of the available data, we believe that our filtered dataset offers an accurate portrait of the compositional variability of the NIP. Nonetheless, we are aware that our analysis may be biased to the filtered felsic NIP rocks, and that our conclusions may not be simply extrapolated to the altered mafic counterparts. Nonetheless, we show that a careful evaluation of the major and trace elements of the filtered samples, as well as their comparisons with other magmatic suites from well-established tectonic settings, allows us to obtain relevant information on the petrogenesis of the NIP and its potential parental mafic magmas. For the sake of simplicity, in the following discussion we will refer to the NIP rock suite as pertaining to the filtered dataset unless otherwise noted.

Fig. 7. (a) Total alkali versus silica (TAS; Bas et al. Reference Bas, Maitre, Streckeisen and Zanettin1986) classification diagram and major-element compositions of the NIP rocks. Dashed line in (a) indicates the boundary between the alkaline and subalkaline fields according to Irvine & Baragar (Reference Irvine and Baragar1971). (b) Variation diagram intended for geochemical classification of altered and metamorphosed rocks (Winchester & Floyd, Reference Winchester and Floyd1977). Red lines in (c) and (d) are fractional crystallization Rhyolite-MELTS models (Gualda et al. Reference Gualda, Ghiorso, Lemons and Carley2012) of the high Mg no. basaltic-andesite from the Popocatépetl volcano (Sample POS-9 from Straub et al. Reference Straub, Gómez-Tuena, Stuart, Zellmer, Espinasa-Pereña, Cai and Iizuka2011) starting at a pressure of 10 kbar and a temperature of 1210 °C, considering a H2O wt % of 4 and a fixed oxygen fugacity (QFM = 1). Accordingly, the major-element concentrations of the NIP rocks cannot be considered as the results of a process of crystal fractionation of an archetypical primitive arc magma. (e) Diagram showing the aluminium saturation index (ASI). Added for comparison are the compositions of some Mexican arc rocks (Alisitos Arc, Morris et al. Reference Morris, Debari, Busby, Medynski and Jicha2019; Mexican Stratovolcanoes, Parolari et al. Reference Parolari, Gómez-Tuena, Errázuriz-Henao and Cavazos-Tovar2021; NW Mexico, Contreras-López et al. Reference Contreras-López, Delgado-Argote, Weber and Valencia2018, Reference Contreras-López, Delgado-Argote, Weber, Torres-Carrillo, Frei and Gómez-Alvarez2021; Torres-Carrillo et al. Reference Torres-Carrillo, Delgado-Argote, Weber and Contreras-López2020); liquid compositions of a fluid-absent partial melting experiment on metagreywackes (Montel & Vielzeuf, Reference Montel and Vielzeuf1997) and the Ollo de Sapo magmatic suite (García-Arias et al. Reference García-Arias, Díez-Montes, Villaseca and Blanco-Quintero2018).
4. Main geochemical features of the Nazas igneous province
The NIP comprises felsic volcanic and volcaniclastic units, with minor volumes of subvolcanic, intrusive and meta-intrusive rocks of calc-alkaline affinity (C. Bartolini, unpub. Ph.D. thesis, Univ. Texas at El Paso, 1998; Campa-Uranga et al. Reference Campa-Uranga, García Díaz and Iriondo2004; Godínez-Urban et al. Reference Godínez-Urban, Lawton, Molina Garza, Iriondo, Weber and López-Martínez2011; Zavala-Monsiváis et al. Reference Zavala-Monsiváis, Barboza-Gudiño, Velasco-Tapia and García-Arreola2012; Lawton & Molina Garza, Reference Lawton and Molina Garza2014; Barboza-Gudiño et al. Reference Barboza-Gudiño, Zavala-Monsiváis, Castellanos-Rodríguez, Jaime-Rodríguez and Almaraz-Martínez2021). These rocks display a limited compositional range, with high SiO2 (average = 74.3 wt %) and alkali contents (average Na2O + K2O = 7.47 wt %; K2O/Na2O = 6.25), low MgO and FeO (average MgO + FeO = 3.15 wt %), low Ca (average 0.92 wt %) and, with the exception of a few samples (5 out of 57 in our filtered dataset), moderate to strong peraluminous compositions (Fig. 7e). According to the total alkali versus silica (TAS) diagram (Bas et al. Reference Bas, Maitre, Streckeisen and Zanettin1986), and to the SiO2 versus Zr/TiO2 classification diagram (Winchester & Floyd, Reference Winchester and Floyd1977), rocks from the NIP can be classified as subalkaline dacites and rhyolites (Fig. 7a, b).
The rare earth element (REE) concentrations of the NIP rocks are fairly homogeneous, displaying a high content of light REEs (LREE; average LaN = 133.02; ‘N’ values are normalized to CI chondrite from McDonough & Sun, Reference McDonough and Sun1995) and flat heavy REE (HREE) patterns, with moderate LREE/HREE ratios (average LaN/YbN = 8.76; Fig. 8a). Compared to modern and ancient Mexican oceanic and continental arc rocks, samples from the NIP display an overall higher REE fractionation (TMVB average LaN/YbN = 6.29; Alisitos–Guerrero arc average LaN/YbN = 1.15; NW Mexican arc average LaN/YbN = 3.35). Multi-element patterns resemble those of arc rocks (Fig. 8b); however, relative to modern and ancient arcs in Mexico, rocks of the NIP show a higher content of most trace elements, either large ion lithophile elements (LILEs), high field strength elements (HFSEs) or LREEs (Fig. 8b). Most of the NIP rocks also display low Sr concentrations and Sr/Nb ratios, together with negative Eu anomalies (Figs 8, 11).

Fig. 8. (a) Chondrite-normalized rare earth element patterns of the NIP rocks. (b) normal – mid ocean ridge basalt (N-MORB) normalized trace-element patterns of the NIP rocks. Added for comparison are the average compositions of the Mexican arcs and the Ollo de Sapo magmatic suite. Values are normalized to CI chondrite (McDonough & Sun, Reference McDonough and Sun1995) and N-MORB (Sun & McDonough, Reference Sun, McDonough, Saunders and Norry1989).
The isotopic compositions of the whole rocks and zircon grains from the NIP suggest the contribution of an isotopically enriched source. In the Sr–Nd systems, for instance, the NIP rocks from central Mexico (Real de Catorce and Villa Juárez; Barboza-Gudiño et al. Reference Barboza-Gudiño, Zavala-Monsiváis, Castellanos-Rodríguez, Jaime-Rodríguez and Almaraz-Martínez2021) display 87Sr/86Sr and 143Nd/144Nd initial compositions that are more enriched compared to modern and ancient Mexican oceanic and continental arc rocks, even relative to the most enriched compositions of the active continental arc (i.e. the TMVB), and exhibit average continental values (Fig. 9a). The initial εHf(t) compositions of detrital zircons from Lower–Middle Jurassic sedimentary deposits (Ortega-Flores et al. Reference Ortega-Flores, Solari, Martini, Ortega-Obregón, Martens and Molina-Garza2020), from the Cretaceous Alisitos–Guerrero island arc (Contreras-López et al. Reference Contreras-López, Delgado-Argote, Weber and Valencia2018; Montaño-Cortes et al. Reference Montaño-Cortes, Molina-Garza and Iriondo2019) and from modern sediments of western Mexico (Cavazos-Tovar et al. Reference Cavazos-Tovar, Gómez-Tuena and Parolari2020) show that Early–Middle Jurassic zircons related to the NIP display mostly negative εHf(t) values (from −13 to +5), in contrast to the positive values (+2 to +14) that characterize zircons of Cretaceous age, associated with the construction of island arcs (e.g. Centeno-García, Reference Centeno-García2017; Fig. 9b). These low εHf(t) values displayed by zircon grains of Jurassic age (i.e. the ‘Cuale-Macias Suite’ in Cavazos-Tovar et al. Reference Cavazos-Tovar, Gómez-Tuena and Parolari2020), coupled with their marked Eu anomalies, have been associated with the involvement of significant volumes of crustal melts derived from the fusion of fertile continental sedimentary rocks (Cavazos-Tovar et al. Reference Cavazos-Tovar, Gómez-Tuena and Parolari2020).

Fig. 9. (a) Initial bulk rock 143Nd/144Nd versus 87Sr/86Sr of dated NIP rocks (Barboza-Gudiño et al. Reference Barboza-Gudiño, Zavala-Monsiváis, Castellanos-Rodríguez, Jaime-Rodríguez and Almaraz-Martínez2021; we excluded sample VAR 07-16c, which displays an anomalously low 87Sr/86Sr initial ratio, indicative of a later thermal reset), the Alisitos and TMVB arc rocks (Morris et al. Reference Morris, Debari, Busby, Medynski and Jicha2019; Parolari et al. Reference Parolari, Gómez-Tuena, Errázuriz-Henao and Cavazos-Tovar2021), and metamorphic lithologies from the Arteaga Complex (Centeno-Garcia et al. Reference Centeno-García, Ruis, Coney, Patchett and Ortega-Gutiérrez1993; Potra et al. Reference Potra, Hickey-Vargas, Macfarlane and Salters2014; Schaaf et al. Reference Schaaf, Díaz-López, Gutiérrez-Aguilar, Solís-Pichardo, Hernández-Treviño, Arrieta-García, Solari and Ortega-Obregón2020). Arteaga Complex data initial ratios have been recalculated to t = 220 Ma. The averages of river water suspended material (Goldstein & Jacobsen, Reference Goldstein and Jacobsen1988) are also displayed as a proxy of continental compositions. (b) Initial Hf isotopic compositions versus age of detrital zircons associated with the NIP (pre-Oxfordian zircons are a selection of crystals older than 163.5 Ma from the Cuale-Macias suite in Cavazos-Tovar et al. Reference Cavazos-Tovar, Gómez-Tuena and Parolari2020; Ortega-Flores et al. Reference Ortega-Flores, Solari, Martini, Ortega-Obregón, Martens and Molina-Garza2020), and the Zihuatanejo arc (Zihuatanejo suite in Contreras-López et al. Reference Contreras-López, Delgado-Argote, Weber and Valencia2018; Montaño-Cortes et al. Reference Montaño-Cortes, Molina-Garza and Iriondo2019; Cavazos-Tovar et al. Reference Cavazos-Tovar, Gómez-Tuena and Parolari2020). Horizontal error bars represent available 2σ uncertainties of individual U–Pb ages.
5. A critical view of the arc-signature concept: use and misuse
Rocks from the NIP show high concentrations of fluid-mobile elements (e.g. LILEs) and depletions in HFSEs, a feature that has been widely interpreted as an arc signature, specifically the subduction influence on arc magmatism (e.g. Miller et al. Reference Miller, Goldstein and Langmuir1994; Stolper & Newman, Reference Stolper and Newman1994). However, these geochemical attributes are not exclusive to arc products but are found in many kind of rocks, such as most clastic continental and oceanic deposits (Goldschmidt, Reference Goldschmidt1933; Shaw et al. Reference Shaw, Reilly, Muysson, Pattenden and Campbell1967; Rudnick & Gao, Reference Rudnick and Gao2003; Plank, Reference Plank, Holland and Turekian2013) that are commonly derived from arcs, but are evidently not arc rocks and do not necessarily relate directly to a contemporaneous arc setting. Since the continental crust as a whole shows this geochemical signature (e.g. Taylor et al. Reference Taylor, McLennan and McCulloch1983; Rudnick & Gao, Reference Rudnick and Gao2003), it is likely that any rocks derived either from erosion or melting of crustal rocks (e.g. S-type granites), as well as most magma that interacts with the continental crust during its ascent, would adopt its compositions, even if they were formed in a tectonic setting away from the influence of any subduction zone. On the other hand, not all the magmatic rocks that compose modern and ancient arcs exhibit this subduction influence. For instance, many magmas from the TMVB do not show an arc signature, but rather an intraplate-like affinity, although these were undoubtedly emplaced in a continental arc setting (Gómez-Tuena et al. Reference Gómez-Tuena, Mori and Straub2018 b and references therein).
Critically, the assignment of an arc signature is only appropriate when applied to true mantle melts, i.e. magmas that originated from melting of a mantle lithology. Interestingly, a subduction influence has recently been reported in a significant number of mid-ocean ridge basalts (MORB), suggesting that it could be a pervasive feature in some portions of the upper mantle itself, without the need for being associated with an active subduction zone (Yang et al. Reference Yang, Langmuir, Cai, Michael, Goldstein and Chen2021). Moreover, the chemical signal of subduction may also be carried to the deep mantle as evidenced by the isotopic compositions of some intraplate basalts (Hart, Reference Hart1988; Stracke et al. Reference Stracke, Hofmann and Hart2005).
Hence, the arc-like features shown by the NIP are definitively not a strong argument for classifying its tectonic environment as a magmatic arc. More generally, we believe that the concept of arc signature must be handled judiciously, especially when dealing with ancient rocks that do not represent true mantle melts. When the tectonic setting is contested and there are not magmatic rocks that represent true mantle melts, as appears to be the case for the NIP, the discussion of the petrogenesis of a magmatic suite should go beyond the recognition of an arc signature, since it may not represent a discriminant, but rather a compositional attribute of many geological materials. For instance, the examination of the style and extent of trace-element fractionation, the employment of petrological models, the measurement of initial isotopic values of whole rocks and related zircons, as well as the comparison with other well-known magmatic suites can help discern the tectonic setting.
6. Petrogenesis of the NIP
The main goal of this study is to discuss whether the NIP magmatic event was related to the development of a Jurassic arc or whether it represents the igneous manifestation of a continental rift associated with the long-term regional extension triggered by Pangaea’s break-up. We compared the chemical features of the NIP with other well-studied magmatic suites (data sources are in the caption of Fig. 7), represented by two emblematic Mexican magmatic arcs, the Alisito–Guerrero island arc and the TMVB continental arc, together with the Jurassic to Cretaceous arc complexes in Central and Southern Baja California and the felsic rocks from the Ollo de Sapo magmatic suite. The latter are located in northern Spain and are thought to represent almost pure upper-crustal sediment melts generated by tectonic extension in an intracontinental or back-arc rift setting related to the Palaeozoic opening of the Rheic ocean (Montes et al. Reference Montes, Catalán and Mulas2010; Villaseca et al. Reference Villaseca, Merino, Oyarzun, Orejana, Pérez-Soba and Chicharro2014; Díaz-Alvarado et al. Reference Díaz-Alvarado, Fernández, Chichorro, Castro and Pereira2016). Moreover, since felsic rocks like those of the NIP may represent derivative liquids of a parental mafic melt, we also performed fractional crystallization models on both major and trace elements to address whether this assumption is feasible and to constrain the eventual chemical features of this putative mafic source. Finally, to test whether the felsic nature of the NIP magmas represents a primary feature, and not the secondary product of mafic melts, we also discuss the composition of NIP rocks in light of the results from experimental studies on sediment melting.
The pervasive occurrence of felsic and strongly peraluminous magmas, coupled with the lack of intermediate and mafic products, represents an outstanding feature of the NIP. Though these kinds of melts are not abundant on Earth, they have been reported in many orogenic (e.g. Harris et al. Reference Harris, Pearce, Tindle, Harris, Pearce and Tindle1986; Harris & Massey, Reference Harris and Massey1994; Nabelek, Reference Nabelek, Janoušek, Bonin, Collins, Farina and Bowden2020) and extensional settings, both arc and non-arc related (e.g. Kanaris-Sotiriou et al. Reference Kanaris-Sotiriou, Morton and Taylor1993; Bryan et al. Reference Bryan, Ewart, Stephens, Parianos and Downes2000; García-Arias et al. Reference García-Arias, Díez-Montes, Villaseca and Blanco-Quintero2018). Conversely, volcanic arcs mainly comprise mafic and intermediate, metaluminous, calc-alkaline rocks (e.g. Gill, Reference Gill1981). While felsic magmas are indeed present in arc settings, they are volumetrically subordinate to basalts and andesites, with peraluminous rhyolites being an uncommon feature of subduction-related magmatism (Turner & Langmuir, Reference Turner and Langmuir2015). Simple Rhyolite-MELTS (Gualda et al. Reference Gualda, Ghiorso, Lemons and Carley2012) modelling results indicate that the major-element concentrations of the NIP rocks are inconsistent with protracted fractional crystallization of a putative parental arc magma (Fig. 7c, d; more details in the figure caption), and likewise that felsic rocks from the NIP do not gain their peraluminosity through a process of crystal fractionation. Rhyolite-MELTS also provides the bulk composition and the amount of crystallized phases at each crystallization step. Therefore, using a compilation of suitable partition coefficients (Kds; online Supplementary Material Table S2), consistent with the changes in major-element composition of the liquid at each step, it was possible to calculate the trace-element concentrations of the crystallizing magma. The results (red lines of Fig. 10) clearly show that the high concentrations of many trace elements exhibited by the NIP magmas are not predicted by crystal fractionation models of a mafic arc melt, even at high silica contents and fractionation level (SiO2 ∼75 % and X ∼74 %; model constraints in the caption of Fig. 10). Still, the concentrations of several incompatible elements, which are expected to increase steadily during crystal fractionation, are scattered and poorly related to the SiO2 concentration (Fig. 10). This evidence, together with the peraluminous trend of the NIP magmas (Fig. 7e), suggests that fractionation of a parental arc magma is unlikely to have generated compositions like those of the NIP.
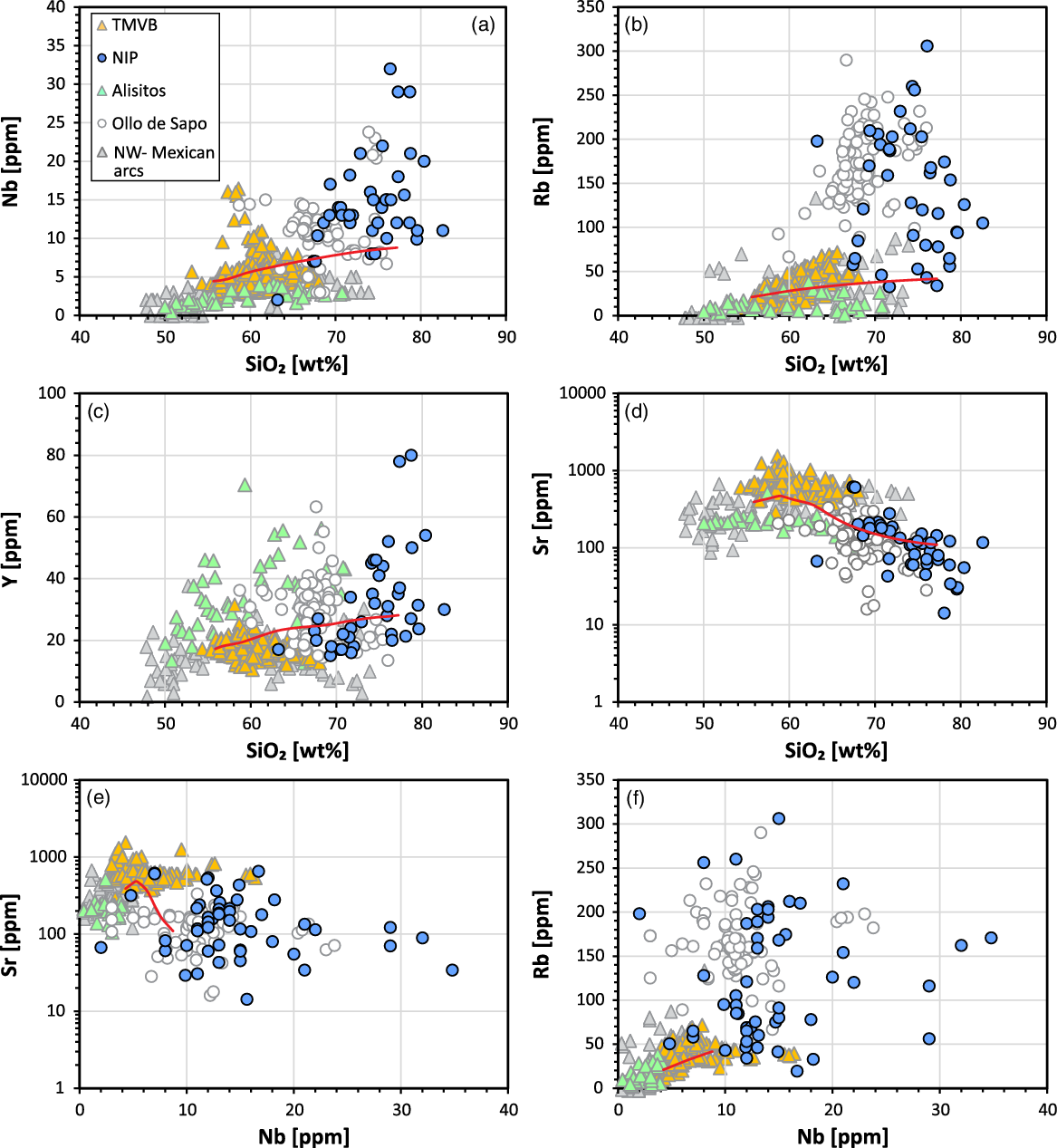
Fig. 10. Concentrations of some incompatible trace elements of the NIP rocks. Compared to arc products, NIP rocks show scattered and generally higher values, similar to the Ollo de Sapo magmatic suite. Red lines represent fractional crystallization paths calculated from the results of the Rhyolite-MELTS experiment described in the caption of Figure 7. Fractional crystallization models for trace elements are calculated considering the variable proportions of crystallizing minerals at different liquid compositions, accordingly to the results of the Rhyolite-MELTS experiment. Individual mineral partition coefficient (Kd) values and sources are reported in online Supplementary Material Table S2.
Compared to intraplate and MOR basalts, arc magmas are notably enriched in Sr and depleted in Nb, as a result of the subduction influence (e.g. Stolper & Newman, Reference Stolper and Newman1994). As rocks from the TMVB and Alisitos–Guerrero arc show an overall high Sr concentration at variable Nb values (Fig. 10e), and given that concentrations of these same elements in the NIP magmas are not the result of fractional crystallization of a parental arc liquid, the Sr/Nb ratio can potentially be used to separate arc magmatic sources from non-arc ones. In addition, considering that Rb and Nb are highly incompatible elements, the Rb/Nb ratio is expected to be almost invariant during processes of crystal fractionation (Fig. 10f) and should reflect the primitive ratio of the magmatic source. Remarkably, in Figure 11, magmas of the NIP and the Mexican arcs form two distinct clusters, implying unrelated magmatic sources. On one hand, the low Sr/Nb ratios displayed by the NIP rocks reinforce the notion of a non-arc genesis. On the other, since intraplate basalts are characterized by very low Rb/Nb ratios (<1; Sun & McDonough, Reference Sun, McDonough, Saunders and Norry1989) and because of the overall invariance of this ratio during fractional crystallization, the data also discard an origin from fractionation of a parental intraplate basalt. Interestingly, the felsic magmas of the Ollo de Sapo suite overlap the NIP compositional cluster, strongly suggesting a similar magmatic origin.
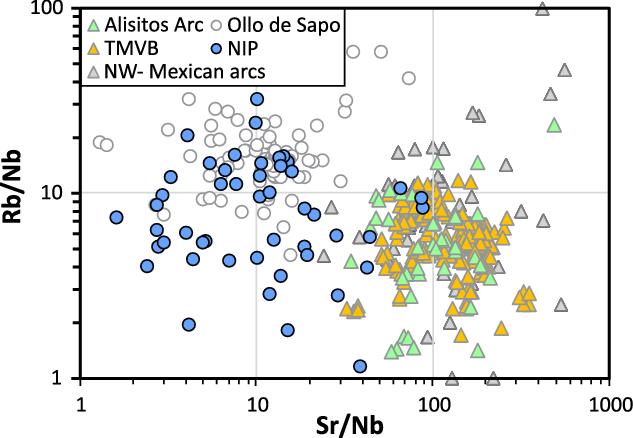
Fig. 11. Rb/Nb versus Sr/Nb variation diagram showing compositional differences between NIP rocks and Mexican arc rocks, suggesting a different magmatic evolution from a separate source.
Experimental studies demonstrate that peraluminosity and high SiO2 contents are common features in pure sediment melts (SiO2 ≥70 %; Patiño Douce, Reference Patiño Douce, Castro, Fernández and Vigneresse2000), over a wide range of pressure–temperature (P–T) and protolith compositions under fluid-absent condition (e.g. Montel & Vielzeuf, Reference Montel and Vielzeuf1997). Since these features are ubiquitous in nearly all Early–Middle Jurassic magmas of southern and central Mexico, we now investigate the involvement of a sedimentary source in the genesis of the NIP.
Peraluminous crustal melts are believed to originate through dehydration-melting reactions under fluid-absent or fluid-deficient conditions (e.g. Thompson, Reference Thompson1982; Clemens et al. Reference Clemens, Stevens and Bryan2020). Muscovite, biotite and hornblende are the most common hydrous minerals that undergo those reactions (e.g. Patiño Douce, Reference Patiño Douce, Castro, Fernández and Vigneresse2000). The incongruent breakdown of these minerals, in fact, provides alkali and water to the newly forming melt, while MgO, FeO, Al2O3 and CaO are preferentially stored in more refractory peritectic mineral species (Patiño Douce, Reference Patiño Douce, Castro, Fernández and Vigneresse2000). In this way, crustal melts are characterized by low contents of these latter elements, while, in contrast, high concentrations of these same elements are a common attribute of most mantle-derived melts, regardless of the tectonic setting (e.g. Gill, Reference Gill1981; Baker et al. Reference Baker, Hirschmann, Ghiorso and Stolper1995). The major-element contents of the NIP rocks entirely overlap the compositions of experimental melts of a metasedimentary source (Fig. 7).
The low CaO contents of the NIP magmas suggest that their potential residual assemblage was composed of plagioclase and/or Ca-garnet in different proportions (Montel & Vielzeuf, Reference Montel and Vielzeuf1997; Patiño Douce, Reference Patiño Douce, Castro, Fernández and Vigneresse2000). However, since the HREEs are rarely fractionated, and most of the NIP magmas show a strong Eu negative anomaly coupled with low Sr/Nb ratios (Figs 8a, 11), it is likely that the unmolten solid contained abundant plagioclase as the main Ca-rich phase. Residual plagioclase has been widely observed in low-pressure experimental melts of sandstones and pelites (Montel & Vielzeuf, Reference Montel and Vielzeuf1997; Patiño Douce, Reference Patiño Douce, Castro, Fernández and Vigneresse2000; Clemens et al. Reference Clemens, Stevens and Bryan2020). At higher pressure (>1 GPa), or in the presence of aqueous fluids accompanying melting, plagioclase becomes unstable, with Ca being mainly incorporated into grossular and diopside (Patiño Douce & Beard, Reference Patiño Douce and Beard1996; Patiño Douce, Reference Patiño Douce, Castro, Fernández and Vigneresse2000; Brown, Reference Brown2013). Moreover, the high K2O/Na2O ratio of the NIP magmas (average 6.25) suggests that the main source of these melts is likely a felsic sedimentary or metasedimentary rock, as melting of mafic rocks (i.e. basalts and amphibolites) produces trondhjemitic melts that are strongly depleted in K relative to melts derived from mica-rich sources (Rapp et al. Reference Rapp, Watson and Miller1991; Rapp & Watson, Reference Rapp and Watson1995; Patiño Douce, Reference Patiño Douce, Castro, Fernández and Vigneresse2000). The high K2O/Na2O ratios of the NIP magmas also suggests fluid-absent conditions during partial melting, since H2O fluxing of (meta)sedimentary rocks would produce melts that are noticeably enriched in Na2O/K2O (Collins et al. Reference Collins, Murphy, Johnson and Huang2020), a feature that is further enhanced when melting occurs at high P (Patiño Douce & Beard, Reference Patiño Douce and Beard1996; Patiño Douce & Harris, Reference Patiño Douce and Harris1998). The geochemical evidence thus suggests shallow melting (<0.8–1.0 GPa) of a felsic (meta)sedimentary source in the absence of free fluids.
The enriched isotopic composition of the NIP rocks supports the involvement of a sedimentary or metasedimentary protolith in the genesis of this magmatic province (Fig. 9a). According to the geological record, Upper Triassic submarine fan deposits (Zacatecas and La Ballena formations, Arteaga and El Chilar complexes; Centeno-García, Reference Centeno-García, Anderson, Nourse, McKee and Steiner2005; Ortega-Flores et al. Reference Ortega-Flores, Solari, Lawton and Ortega-Obregón2014) accreted to the western equatorial margin of Pangaea are the most suitable candidate to represent the source of the NIP rocks. These metasedimentary rocks are mainly metapelite and metasandstone showing enriched isotopic compositions (Centeno-Garcia et al. Reference Centeno-García, Ruis, Coney, Patchett and Ortega-Gutiérrez1993) that are similar to those of the NIP (Fig. 9a). Likewise, the overall negative εHf(t) shown by the magmatic zircons related to the NIP supports this hypothesis, indicating the involvement of an enriched source (Fig. 9b). Also, many rocks from the NIP display a significant amount of inherited zircon grains (Godínez-Urban et al. Reference Godínez-Urban, Lawton, Molina Garza, Iriondo, Weber and López-Martínez2011; Zavala-Monsiváis et al. Reference Zavala-Monsiváis, Barboza-Gudiño, Velasco-Tapia and García-Arreola2012; R. F. Durán-Aguilar, unpub. Master’s thesis, Univ. Nacional Autónoma de México, 2013; Silva-Romo et al. Reference Silva-Romo, Mendoza-Rosales, Campos-Madrigal, Centeno-García and Peralta-Salazar2015; Barboza-Gudiño et al. Reference Barboza-Gudiño, Zavala-Monsiváis, Castellanos-Rodríguez, Jaime-Rodríguez and Almaraz-Martínez2021) with ages that span between Triassic and Proterozoic times (Fig. 12a). They show age groups that are virtually identical to the ones of the Upper Triassic metasedimentary rocks (Fig. 12b), implying that these lithologies are a viable (meta)sedimentary source for the NIP.

Fig. 12. (a) Kernel density distribution (KDE; Vermeesch, Reference Vermeesch2018) of inherited zircons found in magmatic rocks from the NIP (Godínez-Urban et al. Reference Godínez-Urban, Lawton, Molina Garza, Iriondo, Weber and López-Martínez2011; Zavala Monsiváis et al. Reference Zavala-Monsiváis, Barboza-Gudiño, Velasco-Tapia and García-Arreola2012; R. F. Durán-Aguilar, unpub. Master’s thesis, Univ. Nacional Autónoma de México, 2013; Silva-Romo et al. Reference Silva-Romo, Mendoza-Rosales, Campos-Madrigal, Centeno-García and Peralta-Salazar2015; Barboza-Gudiño et al. Reference Barboza-Gudiño, Zavala-Monsiváis, Castellanos-Rodríguez, Jaime-Rodríguez and Almaraz-Martínez2021) and (b) detrital zircons from the Upper Triassic metasedimentary rock sequences of southern Mexico (Martini et al. Reference Martini, Ferrari, López-Martínez, Cerca-Martínez, Valencia, Serrano-Duran, Mahlburg Kay, Ramos and Dickinson2009; Ortega-Flores et al. Reference Ortega-Flores, Solari and de Jesús Escalona-Alcázar2016; Ortega-Flores et al. Reference Ortega-Flores, Solari, Martini, Ortega-Obregón, Martens and Molina-Garza2020). Yellow boxes are a magnification of the zircon frequency distribution centred on the Permian (275 Ma).
The geochemical and isotopic evidence presented above suggests that the NIP is likely the result of fluid-absent melting of these Triassic metasedimentary rocks. However, melts produced experimentally from dehydration melting of micaceous metamorphic rocks yield SiO2 contents ≥70 wt % (Patiño Douce, Reference Patiño Douce, Castro, Fernández and Vigneresse2000). Since at least some samples from the NIP have lower values (Fig. 7a, b), it is likely that a mafic mantle-related component is also involved in the genesis of these magmas. This is also suggested by the positive εNd initial values displayed by some NIP rocks (Barboza-Gudiño et al. Reference Barboza-Gudiño, Zavala-Monsiváis, Castellanos-Rodríguez, Jaime-Rodríguez and Almaraz-Martínez2021) and the positive εHf(t) values in a few Early–Middle Jurassic zircons from modern sediments (Cavazos-Tovar et al. Reference Cavazos-Tovar, Gómez-Tuena and Parolari2020). Although mafic and ultramafic rocks are not present in our set of NIP rocks, we speculate on the nature of this mafic component.
For instance, the average zircon saturation temperature (TzircSat; Watson & Harrison, Reference Watson and Harrison1983) calculated for Early–Middle Jurassic zircons found in modern sediments (Cavazos-Tovar et al. (Reference Cavazos-Tovar, Gómez-Tuena and Parolari2020), here used as a proxy for zircons crystallized from the NIP magmas, indicates that the magmatic temperature was below 824 ± 44 °C (1σ) to preserve inherited zircon grains from being completely resorbed. At the same time, the predicted temperature for the crystallization of NIP magmatic zircon is 735 ± 52 °C (1σ). This has been calculated using the Ti-in-zircon thermometer (TzircTi; Log(T(in zircon) = (6.01 ± 0.03) – ((503 ± 30)/T(K)); Watson et al. Reference Watson, Wark and Thomas2006; details about the Ti measurements of zircons are reported in Cavazos-Tovar et al. Reference Cavazos-Tovar, Gómez-Tuena and Parolari2020) on pre-Oxfordian zircons (Fig. 9b). The results allow the inference of the temperature of formation of the NIP magmas to be at least 735 °C, but not markedly higher than 825 °C, and definitely below the ∼1030 °C, which is the liquidus temperature calculated for an average composition of the NIP magmas using the Rhyolite-MELTS software (Gualda et al. Reference Gualda, Ghiorso, Lemons and Carley2012). If we consider a (meta)pelitic source for the NIP magmas, then pressure must not exceed ∼0.8 GPa to maintain a garnet-free residue in this temperature interval (e.g. Brown, Reference Brown2013), in agreement with the overall low LaN/YbN exhibited by the NIP rocks (Fig. 8a). At these conditions, melting is predicted to start with the breakdown of muscovite to form K-feldspar, at ∼700–750 °C, followed by the progressive dissolution of quartz and feldspar, with biotite as the main ferromagnesian residual phase below ∼850–900 °C (e.g. Brown, Reference Brown2013). At higher temperatures, melting continues with the breakdown of biotite, leaving a refractory residue composed of orthopyroxene and cordierite. However, considering the average TzircSat temperature of 824 ± 44 °C (1σ) estimated for the NIP magmas, we believe that a temperature of magma generation below 850–900 °C is in line with the widespread occurrence of inherited zircons in these rocks. The amount of melt predicted by experimental modelling should be ∼10–30 % at these P–T conditions (Montel & Vielzeuf, Reference Montel and Vielzeuf1997), which is above the physical threshold to reach the melt connectivity transition allowing the melt to migrate (Rosenberg & Handy, Reference Rosenberg and Handy2005). Such P–T conditions cannot be attained in a crust characterized by a normal geothermal gradient of 25–30 °C km−1; therefore, an additional source of heat is needed in order to melt sediments at such a shallow depth (≤ 25 km). Accordingly, we considered that the underplating of basalts could be a viable mechanism to provide the necessary amount of heat to melt the (meta)sedimentary source of the NIP. Because of the geochemical features of the NIP magmas, and the fact that continental primitive arc magmas (i.e. orogenic andesites) might be not sufficiently hot to trigger crustal melting (∼100 to 250 °C less compared to ocean-island-basalt-like basalts; Díaz-Bravo et al. Reference Díaz-Bravo, Gómez-Tuena, Ortega-Obregón and Pérez-Arvizu2014; Zellmer et al. Reference Zellmer, Pistone, Iizuka, Andrews, Gómez-Tuena, Straub and Cottrell2016; Gómez-Tuena et al. Reference Gómez-Tuena, Cavazos-Tovar, Parolari, Straub and Espinasa-Pereña2018 a), we tentatively suggest that the heat source was represented by the injection of intraplate basalts in an attenuated continental crust. The absence of such basalts in the NIP, however, is problematic. A possible explanation for the lack of these mafic rocks could be the modest volume of basalt production during the NIP event, compared to the spatial extent of Upper Triassic metasedimentary sequences in southern and central Mexico, which potentially represented very fertile (e.g. fusible) rocks. In such a scenario, it would be very difficult for a basalt to reach the surface without assimilating some amount of metasedimentary host rocks. Alternatively, the felsic NIP magmas could have formed a rheological barrier, trapping the mafic rocks at depth (e.g. Murphy, Reference Murphy2020). In any case, the majority of these mafic magmas would have likely crystallized at depth as a consequence of heat exchange with the metasedimentary layer that produced the NIP felsic melts. Even in the case that some of the NIP parental magmas reached the surface, they would likely have experienced some degree of crustal contamination and achieved intermediate compositions. A small production of mantle melting is also supported by the relatively small volumes of NIP rocks that are currently preserved in the stratigraphic record of southern and central Mexico.
7. Tectonic model
Our new interpretation of the NIP challenges previously published Early–Middle Jurassic tectonic scenarios for southern Mexico. Most models for Early and Middle Jurassic time imply that subduction was continuous along the Pacific margin of North and South America (Fig. 4). The chemical composition of the NIP rocks, however, questions the interpretation of this province as a continental magmatic arc. Instead, data here presented suggest that the NIP is likely related to sediment melting in a tectonic setting that is not necessarily associated with subduction. These sediment melts could have formed during a regional-scale extensional event that affected wide portions of the present-day southern United States, Mexico and northwestern South America (Fig. 13) and that is well documented in central and southern Mexico by the development of several Early and Middle Jurassic extensional to transtensional sedimentary basins (Goldhammer, Reference Goldhammer, Bartolini, Wilson and Lawton1999; Martini & Ortega-Gutiérrez, Reference Martini and Ortega-Gutiérrez2018). This regional-scale extension began in Middle Triassic time in the proto-GM and continued in central and southern Mexico culminating with the GM opening (e.g. Erlich & Pindell, Reference Erlich, Pindell, Davison, Hull and Pindell2021; Pindell et al. Reference Pindell, Villagómez, Molina-Garza, Graham, Weber, Davison, Hull and Pindell2021). In this context, the NIP may represent a syn-rift igneous province associated with the continental attenuation that preceded the opening of the GM, which is not necessarily related to the Pacific tectonic evolution of North America, but rather a consequence of Pangaea fragmentation.

Fig. 13. Geotectonic reconstruction of the North America – South America divergent boundary during Early–Middle Jurassic time (∼190–170 Ma; after Boschman et al. Reference Boschman, van Hinsbergen, Torsvik, Spakman and Pindell2014; Bayona et al. Reference Bayona, Bustamante, Nova, Salazar-Franco, Gómez and Pinilla-Pachon2020; Erlich & Pindell, Reference Erlich, Pindell, Davison, Hull and Pindell2021 and Pindell et al. Reference Pindell, Villagómez, Molina-Garza, Graham, Weber, Davison, Hull and Pindell2021). The reconstruction shows the novel interpretation of the Nazas province as a magmatic province related to the continental rift between North and South America. In this new scenario, we propose that the Mexican segment of the North American margin was a transform boundary.
Indeed, the complex and non-linear spatial distribution of the volcanic centres of the NIP is difficult to interpret as a back-arc suite either, as it should exhibit an alignment (sub-)parallel to the palaeo-trench, and because no coeval arc rocks of the same age have been reported in central and southern Mexico. Moreover, the mere existence of the Upper Triassic metasedimentary successions negates the back-arc setting hypothesis for the NIP. In effect, Upper Triassic successions were accreted to the Pacific margin of Mexico before the end of Triassic time and constituted the western continental edge of southernmost North America by the beginning of Jurassic time. If a hypothetical Early–Middle Jurassic arc existed, then it must have been subducted along the adjacent trench, together with the currently exposed Upper Triassic successions that would have formed part of the fore-arc region.
Based on our reinterpretation of the NIP, we propose the existence of a Triassic–Middle Jurassic transform fault, here named the Trans-America-transform boundary, that connected the North and South American subduction zones (Fig. 13). Evidence of sinistral block motion along the Mexican continental margin during at least Early and Middle Jurassic time is provided by the occurrence of kilometre-scale, WNW-trending, brittle to brittle-ductile left-lateral faults, which controlled the architecture and evolution of major transtensional basins in southern Mexico (Martiny et al. Reference Martiny, Morán-Zenteno, Tolson, Silva-Romo and López-Martnez2012; Zepeda-Martínez et al. Reference Zepeda-Martínez, Martini, Solari and Mendoza-Rosales2021). Therefore, these major left-lateral faults may be interpreted as the inboard expression of the Trans-America-transform boundary along the Mexican continental margin. Although this structure has not been directly documented, the geological evidence of such a boundary may have been erased by a complex history punctuated by distinct tectonic events such as subduction erosion and terrane accretion (Morán-Zenteno et al. Reference Morán-Zenteno, Corona-Chavez and Tolson1996; Scholl & von Huene, Reference Scholl, von Huene, Hatcher, Carlson, McBride and Martínez Catalán2007; Keppie et al. Reference Keppie, Hynes, Lee and Norman2012; Martini et al. Reference Martini, Fitz, Solari, Camprubí, Hudleston, Lawton, Tolson, Centeno-García, Aranda-Gómez, Tolson and Molina-Garza2012, Reference Martini, Solari and Camprubí2013; Centeno-García, Reference Centeno-García2017; Parolari et al. Reference Parolari, Gómez-Tuena, Cavazos-Tovar and Hernández-Quevedo2018; Cavazos-Tovar et al. Reference Cavazos-Tovar, Gómez-Tuena and Parolari2020).
8. Tectonic implications
The reinterpretation of the NIP as a result of rift-related magmatism offers a new perspective on the Jurassic tectonic evolution of central and southern Mexico. The first and most obvious implication is that subduction initiation beneath the southern and central segments of the Mexican continental margin must be younger than Middle Jurassic time. As a consequence, during Early–Middle Jurassic time, most of the Mexican sector of North America may not represent a convergent margin but instead could be envisioned as a passive or conservative one. Yet, the hypothesis of a conservative margin appears to be more likely for several reasons. An Early–Middle Jurassic Mexican passive margin would have implied the existence of two transform faults that connected this part of the margin with the subduction zones directly northward and southward. However, there is no geological evidence that such lithospheric structures were active during Early and Middle Jurassic time in Mexico. Moreover, numerical models indicate that passive margins are too stable for subduction initiation (Nikolaeva et al. Reference Nikolaeva, Gerya and Marques2011; Stern & Gerya, Reference Stern and Gerya2018) and, besides, there is no documented example of a passive margin collapsing into a subduction zone in Mesozoic and Cenozoic times (Stern & Gerya, Reference Stern and Gerya2018; Arculus et al. Reference Arculus, Gurnis, Ishizuka, Reagan, Pearce and Sutherland2019). Conversely, during Cenozoic time many subduction zones of the western Pacific developed on former transform boundaries as a response to changes in plate motions (Arculus et al. Reference Arculus, Gurnis, Ishizuka, Reagan, Pearce and Sutherland2019 and references therein). Numerical models also support this hypothesis, predicting that conservative margins represent the most favourable sites where new subduction zones are expected to arise (Stern & Gerya, Reference Stern and Gerya2018 and references therein). In the case of Mexico, the scenario that we propose for Early–Middle Jurassic time would meet the two fundamental requirements for subduction initiation (Stern & Gerya, Reference Stern and Gerya2018): (1) the presence of a long (>100 km) lithospheric weakness, i.e. the Trans-America-transform proposed in this work (Fig. 13) and (2) the existence of a density contrast between the oceanic Farallon plate and the North America continent.
The predicted time for the onset of subduction in southern and central Mexico roughly coincides with the beginning of the GM opening (∼167–163 Ma; Cantú-Chapa, Reference Cantú-Chapa, Bartolini and Román Ramos2009; Pindell et al. Reference Pindell, Villagómez, Molina-Garza, Graham, Weber, Davison, Hull and Pindell2021). In our view, this event may represent a cause–effect relationship. Specifically, the production of new oceanic crust in the GM may have produced enough tectonic force to initiate subduction in Mexico, converting the proposed Late Triassic–Middle Jurassic conservative margin into a subduction zone by the end of Middle Jurassic or the beginning of Late Jurassic times. The early manifestation of arc magmatism in Baja California (∼165–160 Ma; Kimbrough & Moore, Reference Kimbrough, Moore, Johnson, Paterson, Fletcher, Girty, Kimbrough and Martín-Barajas2003; Contreras-López et al. Reference Contreras-López, Delgado-Argote, Weber, Torres-Carrillo, Frei and Gómez-Alvarez2021) also supports the onset of subduction by the end of Middle Jurassic time along the central and southern sectors of the Mexican margin, consistent with the proposed time lapse between the subduction initiation and the onset of arc magmatism (∼7 Ma; Arculus et al. Reference Arculus, Gurnis, Ishizuka, Reagan, Pearce and Sutherland2019). In this view, we suggest that the onset of subduction in southern and central Mexico during late Middle Jurassic time was triggered by changes in plate motions related to the opening of the GM, as a result of the Atlantic-related extensional tectonic regime associated with Pangaea break-up.
To conclude, in the same way that the present-day architecture of the North American margin is composed of a complex mosaic of subduction zones connected together by transform faults (Fig. 1), mainly as a result of the Farallon–Pacific oceanic ridges subduction (Plafker & Berg, Reference Plafker and Berg1994; Atwater & Stock, Reference Atwater and Stock1998; Aragón et al. Reference Aragón, D’Eramo, Cuffaro, Doglioni, Ficini, Pinotti, Nacif, Demartis, Hernando and Fuentes2020), we consider that, during Jurassic time, the western American margin was probably composed of both convergent and conservative sectors. Though we cannot prove that subduction of one or more oceanic ridges was going on during Early–Middle Jurassic time, deep seismic tomographic images of the mantle below the Atlantic Ocean shows a number of anomalous low-velocity zones that have been interpreted as the remnants of ancient subducted Farallon-related slabs (van der Meer et al. Reference van der Meer, van Hinsbergen and Spakman2018) This suggests that, during Mesozoic time, subduction was not truly continuous along the western margin of North America, and that the subduction of more than one oceanic plate represents a feasible scenario.
Supplementary material
To view supplementary material for this article, please visit https://doi.org/10.1017/S0016756822000061
Acknowledgements
This work is part of M. Parolari postdoctoral research supported by ‘Programa de Becas Posdoctorales de la UNAM (Universidad Nacional Autónoma de México)’, Mexico. Productive discussions with Laura Mori were helpful in the development of this research. The manuscript benefited greatly from the thoughtful reviews of Brendan Murphy, Nancy Riggs, Iain Neill and two anonymous reviewers.
Declaration of interest
The authors declare that they have no known competing financial interests or personal relationships that could have appeared to influence the work reported in this paper.