1. Introduction
Rocks of the Trinity Peninsula Group occur widely in the northern Antarctic Peninsula, and the South Shetland and South Orkney islands. They are the substrate for the Mesozoic–Cenozoic-age arcs of the Antarctic Peninsula (Smellie, Reference Smellie, Thomson, Crame and Thomson1991; Willan, Reference Willan2003) and form the Trinity Peninsula Group Province of Vaughan & Storey (Reference Vaughan and Storey2000). Metamorphic rocks occurring as xenoliths in granite are thought to represent rocks that underlie the group and show links to Patagonia (Hervé et al. Reference Hervé, Lobato, Ugalde and Pankhurst1996). The group is considered to include the Miers Bluff Formation of the South Shetland Islands (Hervé et al. Reference Hervé, Faundez, Brix and Fanning2006) and the Greywacke-Shale Formation of the South Orkney Islands (Trouw et al. Reference Trouw, Passchier, Simoes, Andreis and Valeriano1997). All these units have a similar association of facies and similar provenance (Andreis, Ribeiro & Trouw, Reference Andreis, Ribeiro, Trouw and Ricci1997) but better dating of sedimentation and deformation events suggests that they be better regarded as two or more tectonosedimentary tracts. The rocks typically comprise successions that have been regarded as turbidites from middle and lower submarine fan settings and have been variously interpreted as a fore-arc basin (Dalziel, Reference Dalziel1984; Storey & Garrett, Reference Storey and Garrett1985) or a slope basin in the upper part of an accretionary complex (Smellie, Roberts & Hirons, Reference Smellie, Roberts and Hirons1996). Despite these differences, there appears to be a consensus that the rocks evolved on the Pacific margin of the Gondwana continent in a subduction-driven setting (e.g. Willan, Reference Willan2003), though not necessarily in their current location (Vaughan & Livermore, Reference Vaughan, Livermore, Vaughan, Leat and Pankhurst2005). Age control for the Trinity Peninsula Group is not strong. The only palaeontological age constraint comes from the South Orkney Islands where Triassic microfossils are reported (Dalziel et al. Reference Dalziel, Elliot, Jones, Thomson, Thomson, Wells and Zinsmeister1981) and where the upper age limit is constrained by subduction-related deformation and metamorphism of Early Jurassic age (Flowerdew, Daly & Riley, Reference Flowerdew, Daly, Riley, Cooper and Raymond2007). Similarly, Lower Jurassic alluvial fan deposits of the Botany Bay Group (Hunter et al. Reference Hunter, Cantrill, Flowerdew and Millar2005) rest unconformably on the turbidites at Hope Bay, and Triassic fossils occur within an allochthonous sedimentary olistolith at Cape Legoupil (Thompson, Reference Thomson1975) (Fig. 1). On the other hand, 40K–39Ar geochronology suggested some parts of the group could be of Early Permian or Carboniferous age (Smellie & Millar, Reference Smellie and Millar1995). Detrital zircon geochronology results from the northern Antarctic Peninsula (Millar et al. Reference Millar, Vaughan, Flowerdew, Fanning, Trouw, Bradshaw and Futterer2003) and more detailed data in Barbeau et al. (Reference Barbeau, Davis, Murray, Valencia, Gehrels, Zahid and Gombosi2010), show strong Permian peaks and suggest Permian or Triassic depositional ages at the oldest. From the South Shetland Islands, Hervé et al. (Reference Hervé, Lobato, Ugalde and Pankhurst1996) reported Middle Jurassic zircons from the Miers Bluff Formation, a unit that is considered part of the Trinity Peninsula Group.

Figure 1. Map of localities mentioned in the text and outcrop distribution of the Trinity Peninsula Group (dark grey) in the northern Antarctic Peninsula (after Paciullo et al. Reference Paciullo, Ribeiro, Andreis, Trouw, Gamble, Skinner and Henrys2002).
In texture and composition, the Trinity Peninsula Group (Doktor, Swierczewsku & Tokarski, Reference Doktor, Swierczewsku and Tokarski1994; Smellie, Reference Smellie, Thomson, Crame and Thomson1991) resembles contemporaneous subduction-driven regional assemblages such as the Torlesse rocks of New Zealand (Mackinnon, Reference Mackinnon1983; Wandres et al. Reference Wandres, Bradshaw, Weaver, Maas, Ireland and Eby2004). Proximity to an active volcanic arc is not supported by the petrology of the majority of the group (Willan, Reference Willan2003) in which quartz and feldspar are sub-equal, and approximately one third of the feldspar is potassic and includes microcline and perthitic grains. Biotite is also common. Lithic fragments constitute only a small percentage of the rock and are mainly of acid and intermediate composition (Doktor, Swierczewsku & Tokarski, Reference Doktor, Swierczewsku and Tokarski1994). Derivation from a contemporaneous or older continental margin arc with widespread plutonic rocks is indicated (Smellie, Reference Smellie, Thomson, Crame and Thomson1991; also compare Wandres et al. Reference Wandres, Bradshaw, Weaver, Maas, Ireland and Eby2004). An exception is the Hope Bay succession that is richer in volcanic detritus possibly derived from the Early Permian arc of the North Patagonia Massif (Willan, Reference Willan2003). The structure of the rocks at Hope Bay also differs and the relationship to the remainder of the group is uncertain.
2. The View Point assemblage
View Point (63°33′S, 057°23′W) is a small headland that sits at the western side of the entrance to Duse Bay in the hook of Trinity Peninsula on the eastern coast of Graham Land (Fig. 1). It forms a peninsula that strikes ENE–WSW and ranges in altitude from sea level at its eastern end to 350 m in the west. The peninsula is about 6 km long and 2.5 km wide. The assemblage described from within the peninsula differs from others Trinity Peninsula Group sequences so far described in having horizons of thick sandstone (Fig. 2) containing coarse to very coarse conglomerate that provides further insights into provenance and depositional setting. The View Point area was described by Aitkenhead (Reference Aitkenhead1965) who recorded the presence of conglomerate at two isolated localities; however, a substantial decrease in the snow and ice cover in the area in recent years has allowed mapping as part of this study to reveal that the conglomerates form at least ten separate units, individually up to 15 m thick with clasts up to 1.3 m in diameter, and to be much more abundant than previously documented. On the peninsula, the rocks are strongly deformed and generally overturned, dipping steeply to the north and younging to the south. The inverted rocks are truncated by an unconformity at the base of a very small, partly fault-bounded outlier of sedimentary breccia close to the tip of View Point (Fig. 2). These rocks closely resemble the Middle Jurassic Botany Bay Group at Hope Bay, 24 km to the northeast (Fig. 1). This correlation supports a Jurassic or older age for the deposits and deformation.
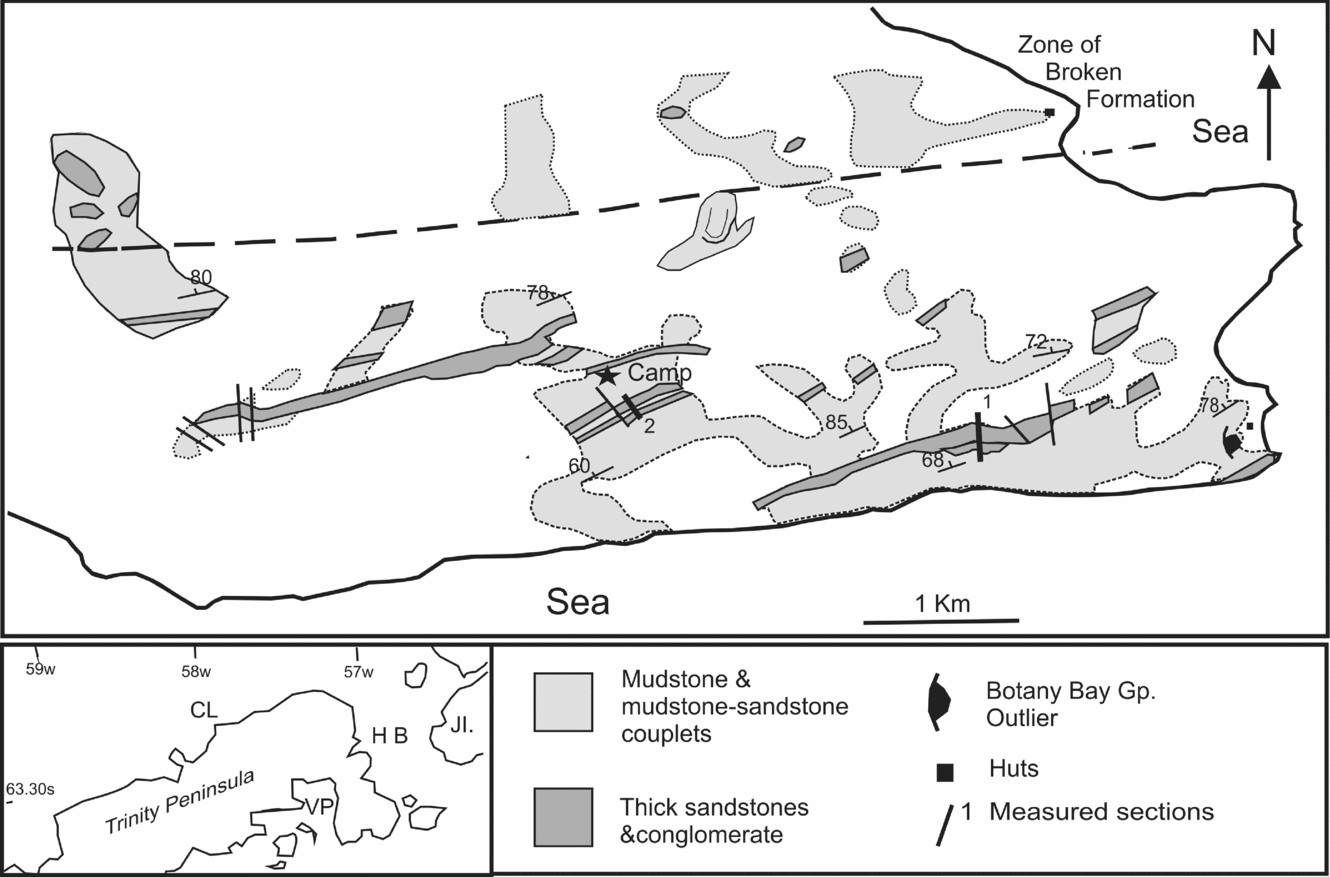
Figure 2. Map of the bed-rock geology in the View Point area. Inset map, location of View Point. VP – View Point; HB – Hope Bay; CL – Cape Legoupil; JI – Joinville Island.
3. Sedimentary facies
There are two dominant sedimentary facies. The most voluminous comprises thinly bedded to laminated siltstone to very fine sandstone with variable thicknesses of mudstone interbeds. Less in volume but better exposed are bands of pale weathering sandstone and conglomerate. Two bands are continuous across more than half the area and a minimum of six bands can be mapped for some distance along strike. Mapping of the thinner bands is complicated by the presence of small folds and small faults with apparent dextral and sinistral components and by extensive block-fields. Thickness measurements on the larger sandstone bands and the distribution of conglomerate within them suggest that the coarse bands are lenticular (Fig. 2).
3.a. Thinly bedded lithologies
In fresh outcrop these lithologies appear very dark grey or black and might be mistaken for massive mudstone, but partial weathering reveals, in abundant fine detail, thin beds of silt and very fine sandstone. There are wide variations in the sandstone to mudstone ratio and individual sandy layers range from 1 to 50 mm in thickness (Fig. 3). Thicker beds in the 20–50 mm range may have well-developed ripple-scale cross-laminations and at the top show an upward gradation into mudstone. Sandstones of this type sometimes show sole structures of either eroded or collapsed bedding parallel burrows or, more rarely, small scale flutes. Load structures and flames are also developed. The thicker units can be described as Tbcd and Tcd turbidites (Walker, Reference Walker, Walker and James1992) and the thinner laminae could also be the deposits of low energy turbid flows or bottom currents. Bottom energy appears to have been extremely low but true pelagic claystone appears to be absent.

Figure 3. Selected outcrop photographs of the Trinity Peninsula Group near View Point. (a) Large (approximately 1.3 m) boulder in conglomerate bed (ice axe is 750 mm long). (b) Typical clast-supported conglomerate (notebook is 170 by 105 mm). (c) Pebbly mudstone. (d) Typical sandstone–mudstone couplets. (e) Large (430 mm) rounded boulder of bedded quartzite. (f) Thicker sandstones (100–150 mm) within sandstone–mudstone couplets. Note erosive base of sandstone. Rocks young to left (south).
Rare but widespread beds of pebbly mudstone and muddy fine sandstone occur. The pebbles are rounded and include quartzite and granitoid granules to cobbles. Pebbly mudstone also occurs within the ‘broken formation’ belt and the original conglomerate locality of Aitkenhead (Reference Aitkenhead1965) appears to have been one of these occurrences.
3.b. Thickly bedded lithologies
These lithologies comprise sandstone and conglomerate beds with minor mudstone interbeds developed in bodies from 10 m to more than 100 m in thickness (Figs 2 to 5). The sandstone is usually medium to fine grained, in some cases with a few millimetres of coarse sand at the base and a weakly developed gradation through fine sandstone into siltstone in the top 10–20 mm. Many of the thicker sandstone beds have layers of quartz granules and/or mudstone clasts that suggest levels of amalgamation between successive sedimentation events. In section 1 (Fig. 4) amalgamated units reach 20 m in thickness and are separated by thin layers typical of the thin-bedded association. Mudstone ‘rip-up clasts’ up to 500 mm in length occur in a few sandstone beds, particularly near their bases. The bases of some sandstone beds are clearly erosive and truncate thinly bedded rocks at surfaces with a relief of up to 400 mm. This erosion probably accounts for at least some of the mudstone clasts.

Figure 4. Details of longest measured section (number 1 on Fig. 2), with class descriptors of Pickering et al. (Reference Pickering, Stow, Watson and Hiscott1986). ‘A’ in columns indicates amalgamation surfaces; f – fault. The stratigraphic base is in the sharp ridge at 063.5538°S 057.4017°W. The section is drawn conventionally but the base is at the top of the hill in a section that is inverted and continues down the south face.
Granule to boulder conglomerate may occur at any level within the sandstone units in layers up to 4 m thick. Despite their thickness, the development of conglomerate beds is highly variable along strike within the sandstone units and conglomerate rapidly grades laterally into sandstone. Conglomerate types range from unstratified poorly sorted pebble to cobble conglomerate (Fig. 3) with dispersed larger boulders (350–1300 mm), through well-organized clast-supported pebble to cobble conglomerate with sandstone to granule matrix, to beds of sandstone with 10–20% granules and rare small pebbles. Most conglomerates were ungraded but grading was seen near the base or near the top in some instances. Reverse grading was evident in a number of beds (Figs 4 & 5). Cross-bedding was not present in either sandstone or conglomerate and imbrication was not observed.

Figure 5. Simplified section (number 2 on Fig. 2) to the south of the camp at 063.5546°S 057.4516°W. The stratigraphic base is at this location and the section is inverted and youngs to the south on a gentle slope.
The clasts in the conglomerates are moderately to very well rounded. Clast counts were made at three localities. A one-metre square was used and compositions noted at 100 mm grid intersections. Areas of very coarse conglomerate were avoided because a few very large clasts would unbalance the numbers. All three sites produced very similar results and simple average values are reported here. The largest and most abundant clasts are quartzite (50%) and granitoids (25%), and these were also the most common type of very large boulder. The remaining 25% were mainly other sandstones, some feldspathic (12%), acid and intermediate volcanic rocks (10%), and mafic volcanic rocks (3%), accompanied by rare small-pebble conglomerate, and very rare fossiliferous sandstone and limestone with indeterminate shelly fossils of probable Palaeozoic age (M. Williams pers. comm. 2006). No metamorphic rocks were seen. Some very large quartzite boulders were elongate parallel to their own internal bedding (Fig. 3) and some large granitoids were sub-quadrate, possibly reflecting the original joint pattern and spacing in the source granitoid.
4. Depositional setting
Sedimentological research on the Trinity Peninsula Group during the last two decades has consistently identified turbidite sedimentation in a submarine fan setting (e.g. Andreis, Ribeiro & Trouw, Reference Andreis, Ribeiro, Trouw and Ricci1997; Paciullo et al. Reference Paciullo, Ribeiro, Andreis, Trouw, Gamble, Skinner and Henrys2002; Ribeiro et al. Reference Ribeiro, Andreis, Paciullo, Trouw, Gamble, Skinner and Henrys2002) and favoured development in a mid-fan lobe environment. Facies models for submarine fan sedimentation have been proposed by Pickering et al. (Reference Pickering, Stow, Watson and Hiscott1986) and Walker (Reference Walker, Walker and James1992). These authors recognize seven major facies associations (A to G) and at least four of these can be recognized in the View Point succession. They are (A) gravels, muddy gravels, gravely muds and pebbly sands, (B) sands, (C) sand–mud couplets and sandy muds, and (D) silty muds and silt–mud couplets. This classification has been applied to the measured section (Fig. 4). These lithologies are also present in the sections described in the papers cited above, but the View Point succession differs from the others in the common presence of coarse conglomerate.
Volumetrically, the View Point succession is dominated by very thinly bedded fine-grained rocks that on slightly weathered surfaces show ‘Bouma’ interval patterns Tbcd or Tcd. They can be equated with facies C and D of Pickering et al. (Reference Pickering, Stow, Watson and Hiscott1986), and are probably the deposits of low energy turbidity flows or other deep-water currents. They reflect prevalent conditions of sedimentation at View Point. The gravely deposits (facies A) are the most varied part of the succession and can be accommodated in the same facies classification framework and is indicated on Figure 4. Closely related to the conglomerates in the field are sandstones with less than 5% pebbles, typically thick/medium-bedded disorganized sands, sometimes clearly in amalgamated groups (Fig. 4) corresponding to facies B1 of Pickering et al. (Reference Pickering, Stow, Watson and Hiscott1986).
Pickering et al. (Reference Pickering, Stow, Watson and Hiscott1986) suggested that their facies A and B deposits are mainly turbidites and to a lesser extent debris flows. On the other hand, Shanmugam (Reference Shanmugam2006) has argued that much sandy bathyal sedimentation is the result of debris flows and that the turbidite model has been too freely applied to deep-water sandy deposits. The View Point deposits with their abundance of boulders in excess of 300 mm show features characteristic of the deposits of debris flows and a lack the features of turbidites. These facies A rocks are probably debrites. They are closely associated with and pass laterally into thick facies B type sandstones (Figs 4 & 5) and therefore it is likely that many of these enclosing sandstones were deposited in the same debris flow process.
Other parts of the Trinity Peninsula Group (see next Section) have been interpreted as turbidites from supra-fan lobes in a middle fan setting. At View Point there appears to be a sharp contrast between the distal character of the fine-grained sediments and very proximal character of the conglomerates. This could be interpreted as an upper fan or canyon mouth assemblage, or alternatively deposits at the base of a submarine slope or shelf edge with periodic sediment-gravity flows.
5. Comparisons with other sections
Paciullo et al. (Reference Paciullo, Ribeiro, Andreis, Trouw, Gamble, Skinner and Henrys2002) reported in detail the succession at Hope Bay. There are two main sandstone-rich bodies, which occur above and below the thin central fine-grained member. Their columns (Paciullo et al. Reference Paciullo, Ribeiro, Andreis, Trouw, Gamble, Skinner and Henrys2002, figs 4 & 5) show 500 m of section, over half measured in detail. Most of the thickness is made up of massive sandstone classified as Bouma Ta or poorly developed Tab or Tac. In addition there are laminated sandstones composed of Bouma Tb intervals, and alternating sandstone–mudstone packages in which Bouma patterns Tae, Tab, Tabd or Tbde can be recognized.
Another interesting Trinity Peninsula Group assemblage occurs to the west of Hope Bay near Cape Legoupil (Fig. 1) and has been described by Ribeiro et al. (Reference Ribeiro, Andreis, Paciullo, Trouw, Gamble, Skinner and Henrys2002). They presented seven representative stratigraphic columns, each showing about 25 m of section. Sediments in their columns, (A–C of fig. 3) are sandstone dominated and the interpretation is similar to that reported from Hope Bay. Columns D and E are mudstone dominated with thin sandstones, only one of which is shown to be graded, and column F includes a thick debris flow deposit. The relative proportions of the various types of sediment are not reported, but their map of Largo Island shows that sandstone is dominant through a succession of about 500 m. The area is unusual in the presence on Gandara Island of a very large olistolith of fossiliferous shallow marine sediment at least 50 m thick and at least 500 m wide as judged from the reported occurrence on the adjacent Kopaitic Island. The succession is sandstone dominated, with cross-bedding, ripple marks and thin lenses of conglomerate. Originally considered to be of Cretaceous age, the brachiopod and gastropod fossils were subsequently shown to be of Triassic age (Thompson, Reference Thomson1975). Ribeiro et al. (Reference Ribeiro, Andreis, Paciullo, Trouw, Gamble, Skinner and Henrys2002) considered the host succession to be made up of turbidites deposited in a mid-fan setting and the olistolith to have been derived from a submarine canyon wall or submarine slope. It is difficult to envisage, however, that an extensive but thin slab (aspect ratio 10:1) could be detached from a submarine canyon wall and transported to a mid-fan position. As with View Point, a base of slope setting remains a viable alternative and suggests that a range of sedimentary environments are represented in the Trinity Peninsula Group.
6. Composition and provenance
Petrological studies of the Trinity Peninsula Group (Doktor, Swierczewsku & Tokarski, Reference Doktor, Swierczewsku and Tokarski1994; Smellie, Reference Smellie, Thomson, Crame and Thomson1991; Willan, Reference Willan2003) show that the group is characterized mainly by quartzo-feldspathic sandstones with a variable and commonly small proportion of lithic clasts. This is consistent with the data from the View Point conglomerates, where the high proportion of quartzite and granitoid clasts also suggests provenance from a sedimentary terrane with granitic intrusions. The sparse occurrence of volcanic clasts in the conglomerates is also consistent with sandstone composition in the more felsic Petrofacies II of Smellie (Reference Smellie, Thomson, Crame and Thomson1991; see also Willan, Reference Willan2003). Willan's (Reference Willan2003) data indicate that View Point and Hope Bay are distinctly different in QFL composition, being more quartzose and more felsic, respectively, than the average. In Chile, composition of sandstones in both the accretionary Duque de York Complex and in the Eastern Andes Metamorphic Complex (east of the Cretaceous batholith) are also quartzo-feldspathic (Faundez, Herve & Lacassie, Reference Faundez, Herve and Lacassie2002) and overlap with Trinity Peninsula Group sandstones and resemble most closely Petrofacies I of Smellie (Reference Smellie, Thomson, Crame and Thomson1991).
The conglomerate clasts at View Point are important because they provide large specimens of the rocks in their source area and constrain transport distances in fluvial and marine environments, in contrast to detrital zircons that may be far travelled. The large clasts are dominated by quartzite and granitoid boulders. The proportions and compositions of larger clast types strongly resemble the tillites and marine glacial sediments of the upper Palaeozoic Whiteout Conglomerate of the Ellsworth Mountains (Matsch & Okangas, Reference Matsch, Okangas, Webers, Craddock and Splettstoesser1992). The Ellsworth Mountains rocks differ in being mainly diamictites with abundant fine-grained matrix. They also include higher percentages of shale and limestone clasts (some with archaeocyathid fossils) than are seen at View Point. It is clear, however, that despite considerable variation through the 1000 m thickness of the Whiteout Conglomerate, quartzite and granite boulders are always the most abundant and that quartzite always exceeds granite (Matsch & Okangas, Reference Matsch, Okangas, Webers, Craddock and Splettstoesser1992, table 1). This compositional pattern is not seen in the Dwyka tillites of southern Africa, where igneous rocks, usually granite, are the dominant component and are accompanied by schist, carbonate rocks (including rare archaeocyathid limestone) and banded iron formation (Visser, Hall & Loock, Reference Visser, Hall and Loock1986). The Fitzroy Tillite in the Falkland Islands (West Falkland) includes common quartzite and a wide range of other lithologies (Aldiss & Edwards, Reference Aldiss and Edwards1999; Stone & Thomson, Reference Stone, Thomson, Vaughan, Leat and Pankhurst2005) including archaeocyathid limestone blocks (Stone & Thomson, Reference Stone, Thomson, Vaughan, Leat and Pankhurst2005). Archaeocyatha are known from the Cambrian of the Transantarctic Mountains and South Australia and point to a significant Antarctic contribution to these tillites. The dominant quartzite plus granite composition at View Point and the Ellsworth Mountains is distinctive and suggests either a common source or possibly secondary source for the View Point conglomerates in Carboniferous–Permian glacial diamictites or related outwash conglomerates of similar composition. The Ellsworth Mountains are probably a relatively small portion of a larger ice-covered tectonic block that originated in the Antarctic–South Africa portion of Gondwana. More specific interpretation is hindered by the allochthonous position of the Ellsworth Mountains and other crustal blocks of West Antarctica (Chernicoff & Zappettini, Reference Chernicoff and Zappettini2003; Curtis, Reference Curtis2001; König & Jokat, Reference König and Jokat2006).
Table 1. Summary of U–Pb zircon geochronology from View Point

1. asterisk indicates data from Millar, Pankhurst & Fanning (Reference Millar, Pankhurst and Fanning2002). All other data are from this study.
While not identical, there are important similarities between the View Point assemblage and the Pliocene–Pleistocene mega-channel deposits of the tectonically active Alaskan margin (Eyles & Lagoe, Reference Eyles and Lagoe1998). Here thinly bedded turbidites are cut by broad channels that include conglomerates and massive sandstones. They differ from the View Point rocks in the common presence of dropstones and the clearly defined channel geometry. The absence of dropstones may indicate that the View Point rocks were either deposited beyond the range of floating ice or at a time of waning glaciation when montane glaciers or ice caps no longer reached the coast. The lack of recognizable channels may simply reflect the smaller area, discontinuous exposure and structural inversion. The View Point thick sandstones and conglomerate beds, with their distinctive grain size and detrital zircon population (see Section 7) probably represent the reworking of conglomerates of Carboniferous or earliest Permian age, probably glacial deposits derived from continental rocks of Gondwana. The matrix of the conglomerate was derived from the same source and does not include the abundant middle Permian zircons typical of Trinity Peninsula Group rocks of probable Triassic age. The revised interpretation is consistent with derivation from an uplifted continental margin with felsic plutonic rocks as suggested by Smellie (Reference Smellie, Thomson, Crame and Thomson1991). The immediate source of the conglomeratic units was probably Carboniferous glacial or glacial outwash units exposed on the shelf edge or locally uplifted parts of the continental margin.
Although a glacial origin for the boulders cannot be proven, the alternatives require specific circumstances. Large boulders (>500 mm) usually occur within mountain valleys and do not escape much beyond the range-front by fluvial transport. Most large boulders are deposited in alluvial fans close to the mountains and only if these intersect the coast do boulders in any quantity reach the sea where they form coast parallel bars or fan deltas. Only where submarine canyon heads reach close to the coastline can cobble- or boulder-rich materials reach deep water. Another alternative is submarine rock avalanches or fault scarp collapse. Both these explanations require high relief and/or active tectonics close to the site of the basin. The rounding of most boulders in the conglomerates is not consistent with this explanation. These alternative explanations require a tectonically active area of continental crust relatively close to the site of deposition.
7. Detrital zircon geochronology and Hf isotope geochemistry
In this paper we present U–Pb geochronology and Lu–Hf isotope geochemistry of zircons separated from six conglomerate clasts and two sandstones collected from the View Point assemblage of the Trinity Peninsula Group. Results are summarized in Table 1 and U–Pb data are given in Table S1 and Lu–Hf data in Table S2 in online Appendix 2 at http://journals.cambridge.org/geo, which also contains a more detailed description of the analysed samples.
U–Pb zircon SIMS geochronology was completed at the Australian National University, Canberra and at the Swedish Museum of Natural History, Stockholm and the analytical procedures are outlined by Williams (Reference Williams, McKibben, Shanks and Ridley1998) and Whitehouse, Kamber & Moorbath (Reference Whitehouse, Kamber and Moorbath1999), respectively. Following geochronology, hafnium isotope compositions of the zircons were measured by laser ablation ICP–MS at the NERC isotope Geosciences Laboratory, Keyworth, Nottingham, UK following the method of Flowerdew et al. (Reference Flowerdew, Millar and Vaughan2006). Further analytical details are given in online Appendix 1 at http://www.journals.cambridge.org/geo. In the following text and diagrams 2 sigma uncertainties are given for all ages and εHf values, unless otherwise indicated.
7.a. Igneous clasts
Most of the zircons separated from granite gneiss clast (DJ.1336.3) are bright and prismatic with aspect ratios of 2:1 and exhibit classic growth zoning under cathode luminescence (CL), a feature normally attributed to magmatic growth (Fig. 6; Corfu et al. Reference Corfu, Hanchar, Hoskin, Kinny, Hanchar and Hoskin2003). Fourteen of 15 analyses from such grains yield a concordia age of 466 ± 3 Ma that dates crystallization of the granite protolith. A few less well faceted and rounded grains are markedly different under CL with complex and non-luminescent patterns, and these grains yield older ages. In view of the lack of zoned magmatic overgrowth, the older grains are interpreted to have been inherited during emplacement of the granite, perhaps from a sedimentary host. The Ordovician magmatic zircons yield εHf values ranging between −6.5 and +5.2 with a weighted average of 0.3 ± 2.1, a value that corresponds to a depleted mantle model age of 1073 Ma (Table S2 in online Appendix 2 at http://www.journals.cambridge.org/geo).

Figure 6. Concordia diagrams for igneous cobbles from the View Point conglomerate. Panels to the right show representative CL images of zircons and the location of analysis spots after zircon geochronology (solid) and Hf isotope geochemistry (dashed). Scale bar with the zircon images is 100μm.
The white granite clast (DJ.1336.9) yielded stubby, well-faceted, CL-bright prismatic zircons with growth zoning, yielding a concordia age of 373 ± 5 Ma (Fig. 6) that dates crystallization of the granite protolith. Like the granite gneiss clast (above), rare grains with more rounded morphologies and different CL characters yield older ages and are similarly interpreted as inherited during emplacement. The magmatic zircons with εHf values between +3.9 and +14.2 yield a weighted average of +7.6 ± 3.5, a value corresponding to a depleted mantle model age of 699 Ma.
Zircons from volcanic clast (DJ.1412.4) are euhedral, well-faceted grains with aspect ratios of 2:1 that display growth zoning under CL. Although the analyses spread slightly along concordia (Fig. 6) the weighted mean of the 206Pb–238U ages for all 18 analyses yields an age of 487 ± 4 Ma, which is considered to date eruption of the volcanic protolith. Zircons yield εHf values between −1.0 and +7.9 and yield a weighted average of +2.2 ± 2.6, a value corresponding to a depleted mantle model age of 783 Ma.
We also present εHf geochemical data for zircons from granite clasts with ages of 464 ± 5 Ma and 3161 ± 13 Ma (Table S2 in online Appendix 2 at http://journals.cambridge.org/geo), originally dated by Millar et al. (Reference Millar, Pankhurst and Fanning2002).
7.b. Sedimentary rocks
The quartzite clast (DJ.1412.2), conglomerate matrix (DJ.1433.3) and the background sandstone–mudstone facies to the conglomerate/sandstone units (DJ.1405.2) have remarkably similar detrital zircon age distributions (Fig. 7). Concordia diagrams and CL images of detrital grains are presented in Figure 8. In all cases the most abundant populations are at 495–500 Ma, 620 Ma, 1020–1110 Ma and 2700–2780 Ma. Relative probability plots constructed with or without discordant data are very similar. Only the sandstone–mudstone couplet (DJ.1405.2) from the voluminous thinly bedded facies demonstrates that the succession is significantly younger than the Late Devonian granite clast. The sample yielded three concordant grains with a weighted mean age of 302 ± 3 Ma indicating that the View Point succession was deposited at or after the Carboniferous–Permian boundary that is placed by Ogg, Ogg & Gradstein (Reference Ogg, Ogg and Gradstein2008) at 299.0 Ma.

Figure 7. Top panels show cumulative frequency distribution plots for the three (meta)sandstone clasts. Filled distributions represent concordant data only and the number of concordant out of the total analysed is given to the right of each panel with the sample number. The black line represents the cumulative frequency distribution when all data, concordant (within 2σ uncertainty) and discordant, are included in the plot construction. Bottom panel shows the εHf versus age distribution for the samples together with those from the igneous clasts. Grey markers indicate Hf analyses performed on zircon whose U–Pb age is discordant.

Figure 8. Concordia diagram for (meta)sedimentary cobbles from the View Point conglomerate and sandstone beds. Panel below shows representative CL images for each sample with the location of spots from zircon geochronology (solid) and Hf isotope geochemistry (dashed). Scale bar is 100 μm.
The εHf values for the detrital zircons are also similar for each sample (Fig. 7). The majority of the dominant ~ 500–620 Ma grains in each sample yielded εHf values between approximately −8 and +2, corresponding to depleted mantle model ages of between 1500 Ma and 1000 Ma. Each sample contained a significant proportion of zircons in this age range with much more negative εHf values, with groupings at approximately −15, −22 and −30, corresponding to depleted mantle model ages of ~ 1740, 1980 and 2300 Ma. Quartzite clast DJ.1412.2 has one ~ 513 Ma grain with an εHf value of −58, which results in a ~ 3290 Ma depleted mantle model age. The other significant detrital zircon age populations that are shared by each sample also share similar εHf compositions. The majority of the 1000–1100 Ma grains have positive εHf values ranging between +2 and +12, corresponding to depleted mantle model ages between ~ 1550 Ma and 1100 Ma. The ~ 2700–2780 Ma populations have negative εHf values of ~ −3 and a depleted mantle model age of ~ 3210 Ma. The young ~ 300 Ma grains, which are only present in sandstone sample DJ.1405.2, have εHf values of approximately −3.5 with a corresponding depleted mantle model age of ~ 1070 Ma.
8. Interpretation of the geochronology and Hf isotope geochemistry
The granite and granite gneiss cobbles are of Middle Ordovician age and the third granite is of Late Devonian age. Granites of very similar ages (474 ± 6 Ma to 476 ± 6 Ma) are reported from the Sierra Grande area of the North Patagonia Massif (Pankhurst et al. Reference Pankhurst, Rapela, Fanning and Marquez2006) and similar but less well constrained ages from granite in the Deseado Massif of southern Patagonia (Pankhurst et al. Reference Pankhurst, Rapela, Loske, Marquez and Fanning2003). Granitoids within this range are also reported from the Famatinian arc in the Sierras Pampeanas further to the north (Chernicoff et al. Reference Chernicoff, Zappettini, Santos, Allchurch and McNaughton2010; Dahlquist et al. Reference Dahlquist, Pankhurst, Rapela, Galindo, Alasino, Fanning, Saavedra and Baldo2008). The volcanic clast is early Ordovician in age and silicic volcanic rocks of similar age (477 ± 4 Ma) also occur in the Famatinian arc (Dahlquist et al. Reference Dahlquist, Pankhurst, Rapela, Galindo, Alasino, Fanning, Saavedra and Baldo2008). Granite cobbles with zircons in a similar range of ages (between 460 and 490 Ma) also occur in the basal Permian La Golondrina Formation of the Deseado Massif (Pankhurst et al. Reference Pankhurst, Rapela, Loske, Marquez and Fanning2003), but the absence of Permian detrital zircons in the matrix at View Point suggests that it is older than the La Golondrina Formation, although the clasts may be derived from a similar source. The Devonian granite clast is, within error, the same age as a granite (371 ± 4 Ma) from the southwestern part of the North Patagonia Massif (Pankhurst et al. Reference Pankhurst, Rapela, Fanning and Marquez2006). The Archaean granite has an age (3161 ± 13 Ma) and Hf isotope composition that matches closely with the Kaapvaal Craton of South Africa (Zeh, Gerdes & Barton, Reference Zeh, Gerdes and Barton2009).
Alternative sources for the Ordovician granites are not compelling. They lie outside the range of crystallization ages for the Cambrian–earliest Ordovician granites of the Ross–Delamerian orogen of the Transantarctic Mountains and southern Australia (Foden et al. Reference Foden, Elburg, Dougherty-Page and Burtt2006). Similarly, they do not correspond to the Cambrian ages recently determined from the Tierra del Fuego basement (Hervé et al. Reference Hervé, Fanning, Pankhurst, Mpodozis, Klepeis, Calderon and Thomson2010) or to Late Precambrian–Cambrian granites in southern Africa (Scheepers & Armstrong, Reference Scheepers and Armstrong2002). The granites are older than ages reported from orthogneiss in southern Graham Land and western Palmer Land (Harrison & Piercy, Reference Harrison, Piercy, Thomson, Crame and Thomson1991; Millar, Pankhurst & Fanning, Reference Millar, Pankhurst and Fanning2002; Milne & Millar, Reference Milne, Millar, Thomson, Crame and Thomson1991).
Alternatives sources for the Devonian granites are difficult to find despite the common occurrence of Devonian zircon in sediments. Hervé, Fanning & Pankhurst (Reference Hervé, Fanning and Pankhurst2003) suggested that granites of this age may be more abundant than present exposures suggest or lie outside South America. A possible hypothesis is that the Lachlan Orogen of Australia–northern Victoria Land and western Marie Byrd Land (Bradshaw, Reference Bradshaw, Cooper and Raymond2007) extends into eastern Marie Byrd Land with a similar range of granite ages to those seen in SE Australia (Birch, Reference Birch2003) and Tasmania (Black et al. Reference Black, McClenaghan, Korsch, Everard and Foudoulis2005).
Detrital zircons from a quartzite clast are dominated by a complex grouping between 500 and 700 Ma accompanied by significant grouping around 1000 Ma (across the Neoproterozoic–Mesoproterozoic boundary) and a scattering of Palaeoproterozoic and Archaean ages. This suggests an early Palaeozoic age for quartzite sedimentation. The pattern is one commonly seen along the Gondwana margin, particularly in the SW Pacific region (Ireland et al. Reference Ireland, Flottmann, Fanning, Gibson and Preiss1998), extending into the Ellsworth–Whitmore Mountains (Flowerdew et al. Reference Flowerdew, Millar, Curtis, Vaughan, Horstwood, Whitehouse and Fanning2007) and also represented in pre-Carboniferous rocks of Patagonia (Pankhurst et al. Reference Pankhurst, Rapela, Loske, Marquez and Fanning2003, Reference Pankhurst, Rapela, Fanning and Marquez2006). Drilling has also revealed Cambrian granodioritic gneiss in the basement of Tierra del Fuego (Hervé et al. Reference Hervé, Fanning, Pankhurst, Mpodozis, Klepeis, Calderon and Thomson2010; Pankhurst et al. Reference Pankhurst, Rapela, Loske, Marquez and Fanning2003; Söllner, Millar & Herve, Reference Söllner, Miller and Herve2000). These occurrences support an extension of the Pampean Orogen into eastern Patagonia including the southern Deseado terrane, a region regarded as an allochthonous crustal block by Pankhurst et al. (Reference Pankhurst, Rapela, Fanning and Marquez2006) that had amalgamated (or reunited) with northern Patagonia by the Permian period. Hervé et al. (Reference Hervé, Fanning, Pankhurst, Mpodozis, Klepeis, Calderon and Thomson2010) reported evidence of a high-grade metamorphic event of Permian age in Tierra del Fuego.
Detrital zircons from the sandstone matrix from the View Point conglomerate show a similar pattern with most ages between 490 and 650 Ma and a scattering of older ages. A Ross–Delamerian contribution (490–650 Ma) is typical of Gondwana margin Palaeozoic rocks. Rather surprisingly, detrital zircons of an age corresponding to that of the granite clasts have not been found. A few grains give younger apparent ages, including a single grain that gives concordant ages at 320 ± 5 Ma. This is thought to be a true age and indicates a source in Late Carboniferous igneous rocks and is, within error, similar to ages (321 ± 2 Ma, 318 ± 2 Ma and 323 ± 3 Ma) reported from northern Patagonia by Pankhurst et al. (Reference Pankhurst, Rapela, Fanning and Marquez2006). It is, however, also close to the ages of granites at Target Hill (Millar, Pankhurst & Fanning, Reference Millar, Pankhurst and Fanning2002), about 350 km SSW of View Point. These few grains are the only representatives of post-Cambrian populations that are well represented in other South American basins (Augustsson et al. Reference Augustsson, Münker, Bahlburg and Fanning2006; Bahlburg et al. Reference Bahlburg, Vervoort, Du Frane, Bock, Augustsson and Reimann2009). This raises some doubts about the provenance of the matrix sediment and a need to consider possible alternative sources. Sediments and metasediments in Dronning Maud Land, the Ellsworth–Whitmore Mountains and the southern Antarctic Peninsula have 500–650 Ma and 1000–1200 Ma aged populations and εHf consistent with origin from 1000–1500 Ma crust (Flowerdew, Millar & Vaughan, Reference Flowerdew, Millar, Vaughan, Horstwood and Fanning2006; Flowerdew et al. Reference Flowerdew, Millar, Curtis, Vaughan, Horstwood, Whitehouse and Fanning2007; Veevers & Saeed, Reference Veevers and Saeed2007). Rocks of similar age and Hf composition are exposed in the Shackleton Range (Will et al. Reference Will, Zeh, Gerdes, Frimmel, Millar and Schmadicke2009, Reference Will, Frimmel, Zeh, Roux and Schmadicke2010) and at Haag Nunataks (Flowerdew et al. Reference Flowerdew, Millar, Curtis, Vaughan, Horstwood, Whitehouse and Fanning2007). More important perhaps, are the ~ 550 Ma grains that have extreme negative εHf values and form a ‘keel’ on Figure 9. Grains of this age and Hf signature are apparently rare from South America (Bahlburg et al. Reference Bahlburg, Vervoort, Du Frane, Bock, Augustsson and Reimann2009) but are relatively abundant in the upper parts of the Ellsworth Mountains succession (Flowerdew et al. Reference Flowerdew, Daly, Riley, Cooper and Raymond2007) and also from sediments and cobbles in Mesozoic sedimentary rocks from the southern Antarctic Peninsula (Flowerdew, Millar & Vaughan, Reference Flowerdew, Millar, Vaughan, Horstwood and Fanning). Flowerdew et al. (Reference Flowerdew, Daly, Riley, Cooper and Raymond2007) proposed these grains must have originated from southern Africa, but with respect to the View Point succession, it is entirely possible that detritus was first deposited in the Ellsworth–Whitmore Mountains block or a geologically similar crustal fragment and then recycled into the View Point basin in Late Carboniferous times. These considerations resonate with the quartzite–granite-dominated character of the large clasts in both the diamictites of the Ellsworth Mountains and the View Point conglomerates discussed above and points, at least in part, to a common provenance. It is also consistent with a southern Target Hill area source for the Late Carboniferous zircons.

Figure 9. Grey markers show age against εHf from this study (diamonds – quartzite clast DJ1412.2; triangles – sandstone DJ.1433.3; circles – DJ.1405.2; crosses – igneous cobbles, undifferentiated) and are overlain with bars representing the range of potential igneous and volcanic source rocks, identified by the adjacent abbreviation, from South America, Antarctica and South Africa: AP – Antarctic Peninsula, EM – Ellsworth Mountains, Antarctica; FA – Famatinian arc, South America; HN – Haag Nunataks, Antarctica; KC – Kaapvaal craton, southern Africa; NC – Nampula Complex, southern Africa; RC – Rayner Complex, Antarctica; SR – Shackleton Range, Antarctica. (Data from Chernicoff et al. Reference Chernicoff, Zappettini, Santos, Allchurch and McNaughton2010; Flowerdew, Millar & Vaughan, Reference Flowerdew, Millar and Vaughan2006; Halpin et al. Reference Halpin, Gerakiteys, Clarke, Belousova and Griffin2005; Thomas et al. Reference Thomas, Jacobs, Horstwood, Ueda, Bingen and Matola2010; Will et al. Reference Will, Frimmel, Zeh, Roux and Schmadicke2010; Zeh, Gerdes & Barton, Reference Zeh, Gerdes and Barton2009.)
In contrast, detrital zircon spectra from the group in other parts of the Trinity Peninsula show distinctly different ages. Five recently published detrital zircon age spectra from the Trinity Peninsula Group (Barbeau et al. Reference Barbeau, Davis, Murray, Valencia, Gehrels, Zahid and Gombosi2010) show a predominance of Permian zircons in all samples with major peaks in the range 270–280 Ma. One sample, from the most southerly location at Lahille Island, Leroux Bay showed a significantly younger peak at 260 Ma. A sixth sample from the Botany Bay Group (Jurassic age) shared the 280 Ma peak, although these rocks rest unconformably on folded and metamorphosed Trinity Peninsula Group rocks. These ages are consistent with our previously unpublished detrital zircon data from Gaston Islands and Hope Bay that show strong populations with essentially the same ‘middle Permian’ age (around 275 ± 5 Ma) forming 30% and 60% of analysed grains, respectively. These rocks also have a broad scatter of grains between 350 and 600 Ma with significant concentrations of zircons at 480, 530 and 560 Ma and lesser concentrations of 350–400 Ma and 1000–1100 Ma. Barbeau et al. (Reference Barbeau, Davis, Murray, Valencia, Gehrels, Zahid and Gombosi2010) suggested that the Trinity Peninsula Group has restricted access to sources in the Gondwana continent based on the subdued peaks of older Palaeozoic zircons in their samples. This could, however, be interpreted as the result of swamping of the older signal by a flood of Permian material rather that a physical barrier. The new data and the compilation of available ages from the southern rim of the Pacific by Barbeau et al. (Reference Barbeau, Davis, Murray, Valencia, Gehrels, Zahid and Gombosi2010) show a ‘flare-up’ of volcanic and plutonic magmatism in Permian times and point to a significant change in the plate tectonic regime at this time. Differing detrital zircon distributions and sandstone modes from the same region, however, show marked differences in provenance, pointing to a number of sources and discrete fluvial systems. Further, their ages are spread across the whole of the Permian period (approximate 50 million years), sufficient for a number of distinct tectonomagmatic events.
9. Discussion
There is no compelling evidence to suggest an accretionary complex origin for the Trinity Peninsula Group in the sense of being rocks deposited on the down-going plate and accreted to an over-riding plate, and the suggestion of deposition on or close to a pre-existing continental basement is consistent with an upper plate origin. Intercalations of red chert up to several metres thick in Trinity Peninsula Group rocks of the South Orkney Islands indicate the presence of a deep-water starved basin and possibly an entrapped piece of older oceanic crust. The outliers of the Botany Bay Group suggest that both the View Point and Hope Bay areas were deformed by Early Jurassic times but evidence that the very similar Trinity Peninsula type rocks of the Miers Bluff Formation of the South Shetland Islands are of Jurassic age (Hervé et al. Reference Hervé, Faundez, Brix and Fanning2006) suggest a second younger belt of sedimentation and deformation that post-dates Late Triassic deformation (Vaughan & Livermore, Reference Vaughan, Livermore, Vaughan, Leat and Pankhurst2005) in the Antarctic Peninsula. The Trinity Peninsula Group may thus comprise two or more discrete sedimentary-tectonic tracts that amalgamated during the Mesozoic and collectively accreted to the magmatic Central Domain of Vaughan & Storey (Reference Vaughan and Storey2000) in Cretaceous times. Amalgamation of two or more lithologically similar but chronologically distinct sedimentary tracts is known also from the Torlesse rocks of New Zealand (Wandres & Bradshaw, Reference Wandres, Bradshaw, Vaughan, Leat and Pankhurst2005). Their location on the Gondwana margin before Cretaceous times is uncertain, but the evidence from the View Point conglomerates clearly suggests an origin on the Gondwana margin with close links to Patagonia but also with links to the region that was either the source of the Ellsworth Mountain-type succession or was Ellsworth-like itself. The allochthonous nature of several blocks in this part of the Gondwana margin makes identifying the site of the latter source difficult, but the size of the material in the View Point conglomerates suggests the source was close and located in the future ‘Weddell Sea’ area. If it were the Ellsworth block, it would require intra-continental displacement to achieve the Jurassic position indicated by König & Jokat (Reference König and Jokat2006). The convergent, multi-source provenance pattern broadly resembles that proposed for Patagonia by Hervé et al. (Reference Hervé, Fanning and Pankhurst2003; Fig. 9), but we would suggest closer proximity of the northern Antarctic Peninsula to the Ellsworth–Whitmore mountain block and possibly East Antarctica and Africa north of the Cape Fold Belt.
Contemporaneous sediments of the Duque de York Complex of the Chilean Pacific margin (Hervé, Fanning & Pankhurst, Reference Hervé, Fanning and Pankhurst2003) resemble much of the Trinity Peninsula Group in having a very dominant Permian peak and a very subsidiary Devonian peak but differ in having weak and variable early Palaeozoic–Late Neoproterozoic components. They also differ from other southern Chilean metasediments in having very few older Palaeozoic zircons and lack the strong Carboniferous, Devonian and Cambrian peaks seen in the Eastern Andes Metamorphic Complex at similar latitude (Hervé, Fanning & Pankhurst, Reference Hervé, Fanning and Pankhurst2003). On the whole, the age spectra in the Trinity Peninsula Group do not resemble those of the contemporaneous accretionary complex of Patagonia but seem closer to the portions of the Eastern Andes Metamorphic Complex (Augustsson et al. Reference Augustsson, Münker, Bahlburg and Fanning2006; Hervé, Calderon & Faundez, Reference Hervé, Calderon and Faundez2008). Some parts of this complex may be as old as Devonian (Faundez, Herve & Lacassie, Reference Faundez, Herve and Lacassie2002) but other parts have detrital zircon peaks of Carboniferous and Permian ages (Hervé, Fanning & Pankhurst, Reference Hervé, Fanning and Pankhurst2003) and probably overlap with Trinity Peninsula Group and Duque de York Complex deposition. Interestingly, both the Trinity Peninsula Group and the Eastern Andes Metamorphic Complex lack the accreted Carboniferous–Permian limestone and Permian red chert that is common in the Duque de York (subduction) Complex (Rapalini et al. Reference Rapalini, Herve, Ramos and Singer2001) and it is possible that the Eastern Andes Metamorphic Complex and the Trinity Peninsula Group share a common supra-subduction tectonic setting in Permian and Triassic times. There is little evidence of an active volcanic arc in the provenance of either unit other than the Permian zircons that due to their small size, typically less than 200 microns, could be far travelled. The origin of Permian zircons and the site of deposition of the Duque de York Complex is discussed in detail by Sepúlveda et al. (Reference Sepúlveda, Palma-Heldt, Herve and Fanning2010).
The absence of Permian zircons from the matrix of the View Point conglomerates is very surprising. It suggests that the View Point rocks are an older part of the Trinity Peninsula Group and pre-date the advent of Permian igneous activity evidenced in other sections. A Late Carboniferous or Early Permian age of deposition, contemporaneous with, or slightly younger than Gondwana glaciation is indicated. This age is consistent with the more quartzose continent-derived character of the sediments and suggests an early onset of basin subsidence, possibly a far-field response to Gondwanian deformation. A possible sequence of sedimentary-tectonic events is:
(1) Initiation of sedimentation on the subsiding trailing edge of the Deseado continental ribbon, and deposition of continental-sourced View Point conglomerate around the time of the Carboniferous–Permian boundary (TPG View Pt, Fig. 10).

Figure 10. Schematic section and time/rock mass diagram for the Trinity Peninsula Group and other continental margin and subduction-related units in the Antarctic Peninsula and Patagonia. Arrows on the left suggest the pattern of westward subduction zone retreat in the Permian and Mesozoic. BBG – Botany Bay Group; CA – Chon Aike suite; CPG – Central Patagonia Granites; K & F – Karroo and Ferrar mafic suites; LeMay & DY – older LeMay and Duque de York accretionary complexes; PB & APB – Patagonia and Antarctic Peninsula batholiths; SCB – Subcordilleran Batholith; So Cpx – Sarmiento Complex; TPG – parcels of Trinity Peninsula Group with U–Pb zircon age control.
(2) Final continental collision of the Deseado and North Patagonia blocks (Gondwana) as proposed by Pankhurst et al. (Reference Pankhurst, Rapela, Fanning and Marquez2006), with the elimination of a subduction zone. Erosion releases abundant Permian zircons into Triassic sediments deposited along the outer edge of the continent (TPG 1, Fig. 10).
(3) Stronger convergence from the end of Triassic times and through the Early Jurassic epoch deforms the Trinity Peninsula Group of the northern Antarctic Peninsula and the Scotia Complex of the South Orkney Islands (Trouw et al. Reference Trouw, Passchier, Simoes, Andreis and Valeriano1997; Vaughan & Livermore, Reference Vaughan, Livermore, Vaughan, Leat and Pankhurst2005). A similar or slightly younger age of deformation is reported from the Duque de York accretionary complex and for the uplift of the Diego de Almagro Schists (Thomson & Hervé, Reference Thomson and Hervé2002).
(4) Relaxation (roll-back) in Jurassic times (Fig. 10) with sedimentation of the Middle Jurassic Botany Bay Group (Hunter et al. Reference Hunter, Cantrill, Flowerdew and Millar2005), Powell Island Conglomerate (Cantrill, Reference Cantrill2000) and Miers Bluff Formation (Hervé et al. Reference Hervé, Faundez, Brix and Fanning2006). Jurassic relaxation may also be the key to the intrusion of the subcordillaran granites in Early Jurassic times (Rapela et al. Reference Rapela, Pankhurst, Fanning, Hervé, Vaughan, Leat and Pankhurst2005). The formation of the Rocas Verdes Basin and Sarmiento Ophiolite in Late Jurassic times (Stern & de Wit, Reference Stern, de Wit, Dilek and Robinson2003) may be a further effect of this episode. A northward opening or ‘unzipping’ of a wedge-shaped basin is recognized by both Stern & de Wit (Reference Stern, de Wit, Dilek and Robinson2003) and Hunter et al. (Reference Hunter, Cantrill, Flowerdew and Millar2005).
(5) Cretaceous convergence, metamorphism and the formation of a foreland thrust and fold belt in Patagonia is followed by major granitic batholith intrusion. The proximity of the batholiths to the present subduction zone points to tectonic erosion of the margin at some time after the Cretaceous period.
Episodic convergence and compression on the Pacific margin has been identified by Vaughan & Livermore (Reference Vaughan, Livermore, Vaughan, Leat and Pankhurst2005). A pattern of alternating extension and convergence along an active margin resembles that seen in the Palaeozoic rocks of eastern Australia (e.g. Foster, Gray & Spaggiari, Reference Foster, Gray and Spaggiari2005; Gray & Foster, Reference Gray and Foster2004). The extension and break-up of Gondwana in Jurassic and Cretaceous times must have been accompanied by a substantial reduction in size of the Pacific basin as a whole and consequently by ocean-ward migration of subduction zones. These episodes of subduction zone retreat, possibly stimulated by mantle plumes (Storey, Reference Storey1995), may well have alternated with periods of compression driven by the arrival at the subduction zone of younger buoyant crust, spreading ridges and oceanic plateaux. It is evident that from Early Permian to Cretaceous times, the sector of the Gondwana margin between Patagonia and New Zealand was extremely active and driven in part by oblique convergence, with sinistral displacement reported from the SE Pacific sector and dextral displacement in the SW Pacific. In this tectonic environment, the relative positions and relationship of events, variously dated by fossils in sediments, detrital minerals, crystallization ages in magmatic rocks and isotopic closure during post-metamorphic cooling, are difficult to integrate (Fig. 10), but because of physical limits to possible transport distances, studies of coarse and very coarse-grained sediments can provide important constraints on reconstructions.
10. Conclusions
The Trinity Peninsula Group of the northern Antarctic Peninsula and the South Shetland Islands has been interpreted as relatively uniform in sedimentary character and composition and has been interpreted as turbidites deposited in a submarine fan setting. This conclusion needs to be reconsidered because parts of the group might be better interpreted as the results of debris flows, slumps and slides on, or at the foot of a submarine slope near the continental edge. The Gandara olistolith probably represents a detached portion of a Triassic shelf succession that had prograded across older synglacial deposits and was subsequently involved in shelf edge collapse.
The View Point succession lacks the abundant Permian zircons typical of the Trinity Peninsula Group. Three detrital zircons from the intra-conglomerate sandstone–mudstone couplets date deposition at 302 ± 3 Ma, at or shortly after the Carboniferous–Permian boundary, indicating that the succession probably represents an older part of the Trinity Peninsula Group of Late Carboniferous to Early Permian age. The conglomerates are dominated by quartzite and granitoid clasts of early Palaeozoic age. Zircons from granitoid clasts and a silicic volcanic clast yield U–Pb ages of 466 ± 3 Ma, 373 ± 5 Ma and 487 ± 4 Ma, respectively and have corresponding average εHft values between +0.3 and +7.6. A quartzite clast, conglomerate matrix and sandstone interbedded with the conglomerate units have broadly similar detrital zircon age distributions and Hf isotope compositions. U–Pb zircon ages of clasts and detrital zircon ages from the sandstone matrix show good correlations with Patagonia. The clast and detrital zircon ages match well with sources within Patagonia; however, the age of one granite clast and the εHf characteristics of some detrital zircons point to a lesser South Africa or Ellsworth Mountains-like contribution, and the quartzite and granite-dominated composition of the conglomerates is similar to upper Palaeozoic diamictites in the Ellsworth Mountains. The conglomerates at View Point are not diamictites but could be either contemporaneous with Carboniferous diamictites and glacial outwash, or derived by subsequent erosion of glacial deposits. The coarseness of the deposits suggests that in Carboniferous–Permian times, before the opening of the Weddell Sea, this crustal block and the eastern side of the present Antarctic Peninsula were in close proximity.
The most likely sources for the abundant Permian zircons in the typical Trinity Peninsula rocks (TPG 1, Fig. 10) are magmatic rocks of northern Patagonia that were intruded following the collision and amalgamation of the Deseado terrane and the North Patagonian Massif (Pankhurst et al. Reference Pankhurst, Rapela, Fanning and Marquez2006). The source of the bulk of the succession is most likely to be Patagonia. The Trinity Peninsula Group was probably deposited in a supra-subduction setting at an early stage of the development of a subduction zone. The Trinity Peninsula Group and the Eastern Andes Metamorphic Complex may represent parts of a related series of upper plate basins inboard of the Duque de York and LeMay accretionary complexes. The LeMay accretionary complex (Willan, Reference Willan2003) appears to overlap with both the older Triassic and younger Jurassic portions of the Trinity Peninsula Group. The Gondwana margin was probably subject to alternating episodes of compression induced by the arrival of more buoyant oceanic crustal elements and extension related to roll-back of the subduction zone. Crustal extension within the Gondwana continent is documented by Dalziel & Lawver (Reference Dalziel, Lawver, Alley and Bindschadler2001), Rapela et al. (Reference Rapela, Pankhurst, Fanning, Hervé, Vaughan, Leat and Pankhurst2005) and Eagles & Vaughan (Reference Eagles and Vaughan2009).
Acknowledgements
Two of the authors (J. D. B & R. A. J. T) thank the British Antarctic Survey for the invitation to join the expedition to View Point. We thank the captain, crew and helicopter pilots of HMS Endurance and BAS staff for their cooperation and assistance with logistics. The authors would like to thank Jon Ineson and Dave Barbeau for constructive and helpful reviews. We also thank Lev Ilyinsky and Kerstin Lindén at the NordSIMS facility, Stockholm. The facility is operated under an agreement between the research councils of Denmark, Norway and Sweden, the Geological Survey of Finland and the Swedish Museum of Natural History. This is NordSIMS contribution 274.