1. Introduction
The Ediacaran–Cambrian (E-C) transition (635–520 Ma) is an important period in Earth history that witnessed the rise of marine oxygen content and the Cambrian Explosion of life (Li et al. Reference Li, Bogdanova, Collins, Davidson, De Waele, Ernst, Fitzsimons, Fuck, Gladkochub, Jacobs, Karlstrom, Lu, Natapov, Pease, Pisarevsky, Thrane and Vernikovsky2008; Och & Shields-Zhou, Reference Och and Shields-Zhou2012; Shields-Zhou & Zhu, Reference Shields-Zhou and Zhu2013; Lyons, Reinhard & Planavsky, Reference Lyons, Reinhard and Planavsky2014; Chen et al. Reference Chen, Ling, Vance, Shields-Zhou, Zhu, Poulton, Och, Jiang, Li, Cremonese and Archer2015). Biological evolution may have been triggered by environmental changes and may also have affected the environment. Many studies have reported changes in both life and environment during this period and many geochemical indices are used in studies of marine environmental change, such as trace elements, rare earth elements, iron speciation, and isotopes of sulphur and molybdenum (Goldberg, Poulton & Strauss, Reference Goldberg, Poulton and Strauss2005; Lyons et al. Reference Lyons, Anbar, Severmann, Scott and Gill2009; Li et al. Reference Li, Love, Lyons, Fike, Sessions and Chu2010; Ling et al. Reference Ling, Chen, Li, Wang, Shields-Zhou and Zhu2013; Och et al. Reference Och, Shields-Zhou, Poulton, Manning, Thirlwall, Li, Chen, Ling, Osborn and Cremonese2013; Chen et al. Reference Chen, Ling, Vance, Shields-Zhou, Zhu, Poulton, Och, Jiang, Li, Cremonese and Archer2015; Cheng et al. Reference Cheng, Li, Zhou, Algeo, Zhang, Romaniello, Jin, Lei, Feng and Jiang2016). In South China, many typical marine sediment sections are exposed, from platform to slope to deep basin, in the Nanhua Basin during the Ediacaran–Cambrian transition, with various lithologies, such as carbonates, siliceous rocks and black shales. By studying some of these sections and other coeval sections in the world, several researchers concluded that the ocean became highly oxygenated in the terminal Neoproterozoic through pulses of oxygen increase in the ocean (Fike et al. Reference Fike, Grotzinger, Pratt and Summons2006; Canfield et al. Reference Canfield, Poulton and Narbonne2007;); meanwhile, other researchers obtained evidence for anoxic or ferruginous seawaters, or even a euxinic environment in the shelf margin area of the Yangtze Block, South China, during the Ediacaran–Cambrian transition (Steiner et al. Reference Steiner, Zhu, Weber and Geyer2001; Goldberg et al. Reference Goldberg, Strauss, Guo and Liu2007; Li et al. Reference Li, Love, Lyons, Fike, Sessions and Chu2010, Reference Li, Love, Lyons, Scott, Feng, Huang, Chang, Zhang and Chu2012; Och et al. Reference Och, Shields-Zhou, Poulton, Manning, Thirlwall, Li, Chen, Ling, Osborn and Cremonese2013; Feng et al. Reference Feng, Li, Huang, Chang and Chu2014; Jin et al. Reference Jin, Li, Algeo, Planavsky, Cui, Yang, Zhao, Zhang and Xie2016). To trace environmental redox evolution, it is crucial to distinguish redox condition changes of deep seawater from surface seawater and to discern changes in the depth of the marine chemocline. Such information may be retrieved through integrated studies on concentrations of Mo and U and isotopic compositions of S and N in the same sedimentary rock samples.
Systematic and prominent enrichments of redox-sensitive trace-metal elements such as Mo, U and V in sediments deposited in anoxic marine environments with good connections to the open ocean would reflect an increase in the global marine trace-metal element reservoir and a global increase in oxygen at the Earth's surface (Algeo & Lyons, Reference Algeo and Lyons2006; Scott et al. Reference Scott, Lyons, Bekker, Shen, Poulton, Chu and Anbar2008; Sahoo et al. Reference Sahoo, Planavsky, Kendall, Wang, Shi, Scott, Anbar, Lyons and Jiang2012), because the global oceanic reservoir of these redox-sensitive elements increased with atmospheric or oceanic oxidation, for example, in the two ‘Great Oxidation Events’ occurring at c. 2.4 Ga and 750–542 Ma (Canfield, Reference Canfield2005; Holland, Reference Holland2006; Lyons, Reinhard & Planavsky, Reference Lyons, Reinhard and Planavsky2014). However, the local depositional environment to record such enrichments of these elements and such global information must have been anoxic or even sulphidic and connected to the open ocean.
The condition of microbial sulphate reduction (MSR), which results in euxinic seawater and pyrite deposition in high-productivity areas of shelf margins (SO4 2- + 2CH2O → H2S + 2HCO3 −), can be recorded by sulphur isotopes (Canfield, Reference Canfield2004). The sulphur isotopic composition of sedimentary pyrite formed through MSR is mainly dependent on the isotopic composition of the marine sulphate reservoir (Holser et al. Reference Holser, Schidlowski, Mackenzie and Maynard1988) and on the magnitude of MSR isotope fractionation, which varies with sulphate concentration in the seawater (Habicht et al. Reference Habicht, Gade, Thamdrup, Berg and Canfield2002, Reference Habicht, Salling, Thamdrup and Canfield2005). MSR processes in pore water within sediments or euxinic seawater under different conditions produce various sulphur isotopes of pyrites in the sediments.
Nitrogen is an essential element for life, and the marine nitrogen cycle is mainly driven by biological processes. There are four main processes for the nitrogen cycle in the modern ocean (as shown in Fig. 1): N2 fixation by azotobacteria from the atmosphere into organic particulate N; nitrification, in which organic N or NH4 + is oxidized to NO3 −; denitrification, in which NO3 − is converted back to low-valence nitrogen (N2O or N2); and N assimilation, in which NO3 − or NH4 + is assimilated by eukaryotic phytoplankton in the surface seawater (Sigman, Karsh & Casciotti, Reference Sigman, Karsh and Casciotti2009). These nitrogen cycle processes have different isotope fractionations. Generally, N2 fixation has small nitrogen isotope fractionations (ΔPN-N2 = 0 –2 ‰) when azotobacteria use molybdenum enzymes (Zerkle et al. Reference Zerkle, Junium, Canfield and House2008; Sigman, Karsh & Casciotti, Reference Sigman, Karsh and Casciotti2009), but a large nitrogen isotope fractionation (ΔOrg-N2 = −8 ‰) occurs in N2 fixation when bacteria only use iron or vanadium enzymes in a sulphidic water column depleted in Mo (Zhang et al. Reference Zhang, Sigman, Morel and Kraepiel2014). There is no nitrogen isotope fractionation during nitrification when NH4 + is transformed to NO3 − completely under oxic conditions, while the fractionation is large (ΔNH4+ - NO2- = ~16 ‰) (Sigman, Karsh & Casciotti, Reference Sigman, Karsh and Casciotti2009) when oxygen is not sufficient. A small nitrogen isotope fractionation (ΔNO3- - N2 = 1–4 ‰) has been found in denitrification occurring in sediments (Prokopenko et al. Reference Prokopenko, Hammond, Berelson, Bernhard, Stott and Douglas2006), whereas large fractionations arise in denitrification occurring in the water column (ΔNO3- - N2 = 20–30 ‰) (Sigman, Karsh & Casciotti, Reference Sigman, Karsh and Casciotti2009), resulting in a 15N-enriched marine nitrate reservoir. In addition, Higgins et al. (Reference Higgins, Robinson, Husson, Carter and Pearson2012) proposed a model in which 15N is depleted in eukaryotic biomass when the marine nitrogen reservoir is dominated by NH4 + under anoxic conditions because there is a large nitrogen isotope fractionation (ΔNH4+ - Org is up to ~+27 ‰) where primary producers assimilate recycled NH4 + as a nitrogen resource. Nitrogen isotopic compositions of organic sediments could record various isotopic signatures of primary producers in the photic zone, reflecting different nitrogen species used by living organisms on continental margins or in basins, thus reflecting redox conditions of surface waters and the depth of the chemocline (Ader et al. Reference Ader, Sansjofre, Halverson, Busigny, Trindade, Kunzmann and Nogueira2014).

Figure 1. Brief introduction of marine nitrogen cycle, modified from Godfrey & Falkowski (Reference Godfrey and Falkowski2009).
To explore the evolution of the local marine redox environment and biological processes in a typical area on the Yangtze shelf margin during the early Cambrian era, we selected fresh samples from a drill core at Daotuo in Songtao County, northeast Guizhou Province, and measured redox-sensitive element (Mo and U) concentrations, bulk nitrogen isotopes and sulphur isotopes in pyrite in organic-matter-rich black shales and carbonates. Using these data, we aim to trace the variation of marine redox conditions and the nitrogen cycle in this typical area on the Yangtze shelf margin. The results of our analyses probably reflect the local marine information of the Yangtze shelf margin area as spatial heterogeneity of marine redox chemistry may have existed in the Nanhua Basin of South China during the early Cambrian (Jin et al. Reference Jin, Li, Algeo, Planavsky, Cui, Yang, Zhao, Zhang and Xie2016).
2. Stratigraphic setting
In South China, successive marine sedimentary strata of the Nanhua Basin are exposed from the Ediacaran to the Cambrian. Stratum and palaeogeographic reconstruction shows three types of sedimentary facies in the Nanhua Basin: carbonate platform, transitional slope and deep basin (Fig. 2) (Zhu et al. Reference Zhu, Zhang, Steiner, Yang, Li and Erdtmann2003, Reference Zhu, Strauss and Shields2007). The studied drill core located at Daotuo in Songtao County of NE Guizhou province, China, represents the mid-depth slope facies of the Nanhua Basin. The rocks of this drill section span from the pre-Cryogenian Banxi Group to the early Cambrian Qingxudong Formation. Our samples collected from the drill core are from the upper Liuchapo Formation (within the upper part of which the boundary between the Precambrian and Cambrian is located (Chen et al. Reference Chen, Ling, Vance, Shields-Zhou, Zhu, Poulton, Och, Jiang, Li, Cremonese and Archer2015)) to the Jiumenchong Formation (equivalent to the Niutitang Formation, whose middle and upper parts belong to Cambrian Stage 3 (Guo et al. Reference Guo, Shields, Liu, Strauss, Zhu, Pi, Goldberg and Yang2007)) (see Fig. 2). The Liuchapo Formation consists of dark-grey to black cherts and cherts interbedded with shales and is conformably underlain by the Doushantuo Formation and conformably overlain by the Jiumenchong Formation. The lower Cambrian Jiumenchong Formation is c. 120 m thick and consists mainly of carbonaceous shale, black shale and argillaceous carbonate. Near the base of the formation are a black shale layer containing phosphatic nodules and a polymetallic sulphide-rich layer. This formation is overlain by the Palang Formation, which belongs to Cambrian Stage 4. Several ages have been reported for the polymetallic sulphide-rich layer and adjacent black shale, including Pb–Pb isochron ages of 521 ± 54 Ma for the sulphide ores from the Sancha section in Hunan Province, 531 ± 24 Ma for the lower black shales from Zunyi in Guizhou Province (Jiang et al. Reference Jiang, Chen, Ling, Yang, Feng and Ni2006), a Re–Os isochron age of 521 ± 5 Ma for the sulphide ore samples from three sections in Guizhou and Hunan Provinces (Xu et al. Reference Xu, Lehmann, Mao, Qu and Du2011) and a SHRIMP (sensitive high-resolution ion microprobe) zircon U–Pb age of 522.7 ± 4.9 Ma from a tuffaceous bed in the basal Niutitang Formation in the Taoying section in Guizhou (Wang et al. Reference Wang, Shi, Jiang and Zhang2012). These ages suggest that the polymetallic sulphide-rich layer should lie at the base of Cambrian Stage 3. Samples for this study from the Daotuo drill core include cherts, black shales, carbonaceous shales and argillaceous carbonates of the early Cambrian upper Liuchapo Formation and Jiumenchong Formation. (Fig. 2).

Figure 2. Map of sedimentary facies on the Yangtze block and the stratigraphic column of the drill core at Daotuo (red triangle), Songtao County, NE Guizhou Province, South China, in the transitional period between Ediacaran and early Cambrian, modified from Guo et al. (Reference Guo, Shields, Liu, Strauss, Zhu, Pi, Goldberg and Yang2007).
3. Material and methods
For geochemical and isotopic analyses, samples from the Daotuo drill core of the early Cambrian upper Liuchapo Formation and Jiumenchong Formation were carefully selected to avoid diagenetic or hydrothermal veins. Visible pyrite nodules and veins were discarded to minimize the effect of late diagenetic processes on bulk pyrite chemical analyses. The samples were ground to 200-mesh powder and oven-dried at 60 °C for 3 h in preparation for trace element and isotope analyses. For all samples, we used 2 mol L−1 HCl to dissolve carbonate and phosphate, and concentrated HF + HNO3 to fully dissolve the remaining silicate components in Teflon cubes at a temperature of 190 °C. The two solutions of each sample were dried by heating and evaporating, re-dissolved in 3 % HNO3, and then mixed together for trace element analyses. Trace elements of bulk samples were measured with an ELEMENT II ICP-MS at the State Key Laboratory for Mineral Deposits Research, Nanjing University. Analytical uncertainties for most elements were lower than 5 %.
For analyses of total organic carbon and total nitrogen contents, c. 200 mg of bulk powder of each sample was weighed and leached in 2 mol L−1 HCl overnight to thoroughly remove carbonate. After centrifugation, the solution was poured out, and the remains were washed and centrifuged with Milli-Q water to remove CaCl2 and residual HCl and then dried at 60 °C. Concentrations of total organic carbon and total nitrogen of the decarbonated samples were measured with a CHN-O-Rapid element analysis instrument at the Center of Modern Analysis, Nanjing University.
For bulk sample N isotopic analyses, 20–30 mg of powder of each sample was weighed in tin capsules according to the total nitrogen content. The powders were combusted to convert total nitrogen to NO2, and NO2 was reduced by copper-core pillar into N2 in the elemental analyser (Flash-EA); the isotopic composition of N2 was then analysed using a Thermo MAT 253 gas mass spectrometer at the State Key Laboratory for Mineral Deposits Research, Nanjing University. Nitrogen isotopic composition measurements were corrected by laboratory standards Aladdin KNO3 and urea, with the standard deviation of multiple repeated measurements better than ±0.1 %. Nitrogen isotopic compositions were reported relative to air.
For sulphur isotopic analyses, a wet chemical extraction method was used to leach the pyrite sulphur from each bulk sample. Sulphur in the pyrite was liberated through hot CrCl2, and then reacted with AgNO3 solution to convert it completely into Ag2S. Approximately 0.4 mg of Ag2S from pyrite extractions was combusted with ~0.6 mg of V2O5 and analysed using a Delta V Advantage isotope ratio mass spectrometer, at the Key Laboratory of Economic Stratigraphy and Palaeogeography, Nanjing Institute of Geology and Palaeontology, Chinese Academy of Science. The NBS-127 (BaSO4), IAEA-S-1 2 3 (Ag2S) standards and other laboratory standards (FeS2 and CuFeS2) were used to correct S isotope data, and analytical reproducibility was better than ±0.3 ‰. Sulphur isotope compositions were reported relative to the V-CDT (Vienna Cañon Diablo Troilite) standard.
Major elements of bulk rocks were measured using X-ray fluorescence (XRF) methods in the ALS Laboratory Group's Mineral Division, ALS Chemex, in Guangzhou, China.
4. Results
Concentrations of Al, TOC, TN and trace elements and isotope values of δ15N and δ34S for samples of the early Cambrian upper Liuchapo Formation and Jiumenchong Formation are given in Tables 1 and 2. We divide the studied section into three intervals based on the lithology and the variation trends of δ15Nbulk and δ34Spy (Fig. 3). Interval I includes cherts or black cherts in the upper part of the Liuchapo Formation, which probably represents the Fortunian and early Stage 2. Interval II is characterized by phosphatic nodules and a polymetallic enrichment layer in the lower Jiumenchong Formation black shales, which represents the upper Stage 2 and early Stage 3 because the polymetallic sulphide-rich layer occurs just below the boundary between Stages 2 and 3 (Jiang et al. Reference Jiang, Chen, Ling, Yang, Feng and Ni2006; Xu et al. Reference Xu, Lehmann, Mao, Qu and Du2011). Interval III consists of carbonaceous shales and muddy limestones of the middle and upper Jiumenchong Formation, possibly representing the middle and upper Stage 3.
Table 1. Al2O3, Mo, U and TOC contents of Daotuo drill core samples
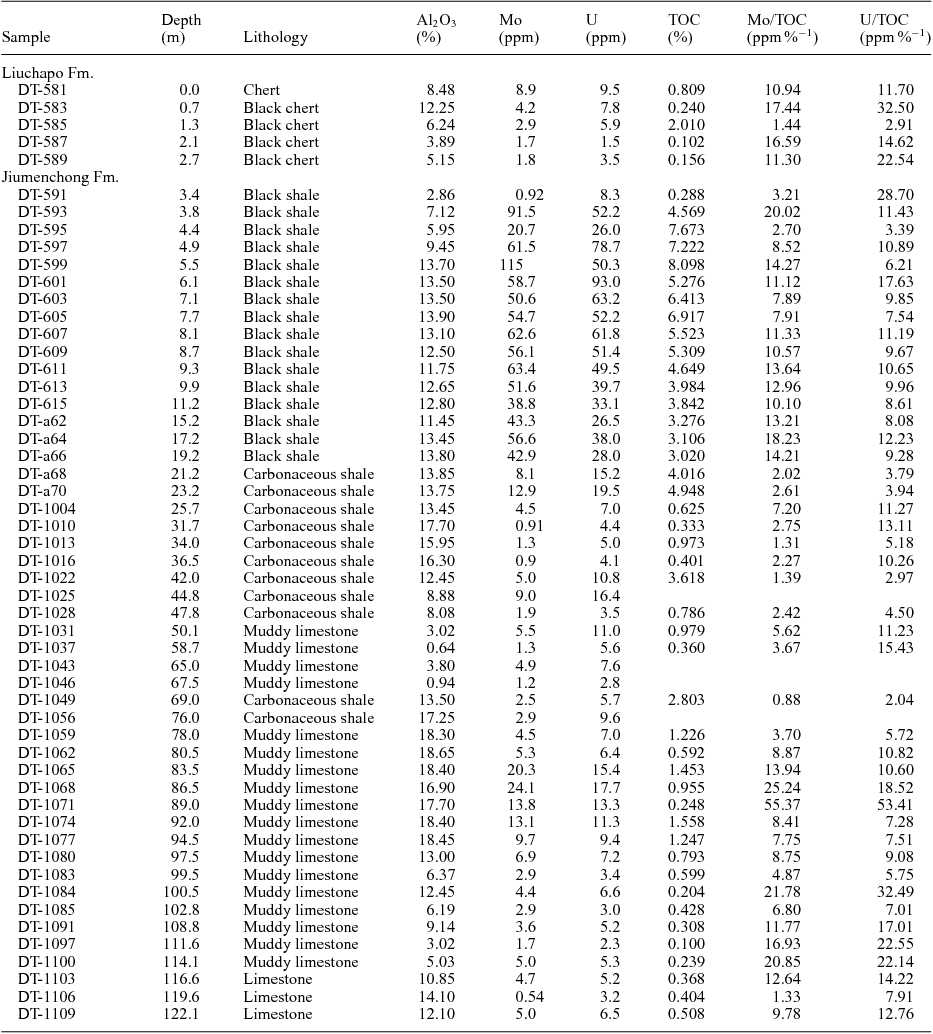
Table 2. TOC, TN, Sulphur- and nitrogen-isotope ratios of Daotuo drill core samples


Figure 3. Concentrations of Mo, U, C and Ndecarb, and isotope compositions of sulphur and nitrogen, in early Cambrian samples from Daotuo drill core.
The studied samples from Interval I have low concentrations of Mo (0.92–8.9 ppm) and U (1.5– 9.5 ppm). The δ34Spy values of this interval are relatively high (5.1–15.9 ‰, averaging 9.4 ‰). All studied samples from Interval I have relatively low δ15Nbulk values (1.2–1.9 ‰), except for one sample that has a high value of 3.5 ‰ occurring as a sharp positive excursion.
The Interval II black shale samples have high concentrations of Mo and U (averages of 57.9 and 49.6 ppm, respectively), with one black shale sample from the polymetallic sulphide-rich layer (Guo et al. Reference Guo, Shields, Liu, Strauss, Zhu, Pi, Goldberg and Yang2007; Xu et al. Reference Xu, Lehmann, Mao, Nägler, Neubert, Böttcher and Escher2012; Och et al. Reference Och, Shields-Zhou, Poulton, Manning, Thirlwall, Li, Chen, Ling, Osborn and Cremonese2013) showing the highest concentrations of Mo (115 ppm) and U (93.0 ppm) in all analysed samples of the section. In Interval II the lowest δ34Spy values (−16.8 to −14.8 ‰) of all the studied samples also occur. In addition, the mean δ34Spy of Interval II is relatively low (−1.3 ‰). At the top of Interval II, the δ34Spy values rise and become positive (from 0.03 ‰ to 5.6 ‰). The δ15Nbulk values in Interval II are very low, from −1.0 ‰ to −3.2 ‰, but rise to positive values (0.9–1.7 ‰) at the top of this interval.
In Interval III, there are relatively low concentrations of Mo (<25 ppm) and of U (<18 ppm) except for two samples (20.3 and 24.1 ppm). The δ34Spy values of most samples from this interval are high (1.4–19.2 ‰, averaging 8.0 ‰) except for two negative values close to 0 ‰. The δ15Nbulk values are between 2 ‰ and −2 ‰ with a decreasing trend from the bottom to the top of this interval.
5. Discussion
5.a. Marine environment in the Yangtze shelf margin area implied by covariations of Mo vs U and Mo vs TOC
Trace elements in black shale can reflect information about the lithogenous sources from the weathering and erosion of the continents or the authigenic seawater source (Goldberg, Reference Goldberg and Shepard1963; Piper & Calvert, Reference Piper and Calvert2009). Authigenic seawater components, such as organic matter, pyrite and phosphorite, can record the original environmental information of the ocean. Because Al is an essential element of aluminosilicate rocks in weathering and eroding continents and its concentrations in detritus eroded from continents are similar, and much higher than that in seawater, the content of Al2O3 is frequently used to evaluate the fraction of trace elements from terrigenous debris in a marine deposit (Taylor & McLennan, Reference Taylor and McLennan1985; Wedepohl, Reference Wedepohl1995; Piper & Calvert, Reference Piper and Calvert2009). As shown in Figure 4A and B, there is no clear correlation between Mo or U concentrations and Al2O3 concentrations in the studied samples. Compared with the low Mo and U concentrations in PAAS (Post-Archaean Australian Shale, Mo = 1 ppm, U = 3.1 ppm; Taylor & McLennan, Reference Taylor and McLennan1985), which represents fine detrital sediments with little or no organic matter, Mo and U concentrations in most of the studied samples are clearly higher. These observations indicate that Mo and U contained in most of the studied samples were dominantly deposited from a seawater source and thus could record information about the marine environment.

Figure 4. Analyses of Mo, U concentrations vs Al2O3, total nitrogen (TNdecarb), total organic carbon (TOCdecarb) and C/Ndecarb data in the decarbonated samples of Daotuo drill core.
According to studies on modern marine sediments, Mo concentrations in sediments can be up to or greater than 100 ppm under a continuous euxinic marine environment, while they are lower than 25 ppm under oxic or dysoxic environmental conditions (Scott & Lyons, Reference Scott and Lyons2012). Strong microbial sulphate reduction in high-productivity marine settings such as semi-restricted shelf margins creates a euxinic marine environment (with the presence of S2− in seawater) and stimulates the precipitation of Mo (Canfield, Reference Canfield2004; Li et al. Reference Li, Love, Lyons, Fike, Sessions and Chu2010). Unlike the precipitation of Mo, which requires sulphidic redox conditions, soluble U (VI) from oxic seawater can be reduced to insoluble U (IV) and deposited into sediments under anoxic conditions without sulphide (Tribovillard et al. Reference Tribovillard, Algeo, Lyons and Riboulleau2006). Based on analyses of iMo and U concentrations of sediments from various representative modern marine environments, different covariations of enrichment factors of Mo and U (expressed as Mo-EF and U-EF, respectively, and defined by X-EF = (X/Al)sample/(X/Al)PAAS; Taylor & McLennan, Reference Taylor and McLennan1985) in sediments have been found and used to distinguish various redox environments (Algeo & Tribovillard, Reference Algeo and Tribovillard2009; Tribovillard et al. Reference Tribovillard, Algeo, Baudin and Riboulleau2012). Algeo & Tribovillard (Reference Algeo and Tribovillard2009) show that different Mo-EF vs U-EF covariation patterns reflect various redox conditions and particulate shuttles of marine sedimentary environments, based on a study of three different modern low-oxygen marine environments (the California Borderlands as an open marine setting, Cariaco Basin, Venezuela, as a particulate shuttle basin, and the Black Sea as a strongly restricted basin).
Rapid Mo precipitation in a strongly restricted euxinic basin would deplete dissolved Mo in the water column in the basin due to limited Mo replenishment from the open ocean. Therefore, Mo/TOC ratios of sediments would decrease with the enhancement of the extent of water-mass restriction. Research on modern marine sedimentary environments with diverse extents of restriction (Black Sea; Framvaren Fjord, Norway; Cariaco Basin; and Saanich Inlet, Canada) has found various slopes of the Mo–TOC linear covariation, which can be used to constrain the extents of marine water-mass restriction of palaeoenvironments (Algeo & Lyons, Reference Algeo and Lyons2006; Algeo & Rowe, Reference Algeo and Rowe2012).
In this study, we apply Mo-EF vs U-EF and Mo vs TOC covariations to the Daotuo drill core samples to constrain the evolution of redox conditions and the restriction extents of the sedimentary environment in the study area of the Yangtze shelf margin during the early Cambrian. Mo-EF–U-EF covariation of the Daotuo drill core samples is shown in Figure 5A. The overall distribution of the studied samples shows a well-defined pattern of Mo-EF–U-EF covariation, which slightly deviates from the pattern defined for unrestricted modern marine settings (grey area in Figure 5A; Algeo & Tribovillard, Reference Algeo and Tribovillard2009). This likely indicates a weakly restricted environment on the Yangtze shelf margin, without particulate shuttle enrichment for Mo, which is consistent with the implication of the Mo/TOC data of the samples discussed below. Furthermore, the oceanic Mo concentration in the early Cambrian is estimated as 50 nmol L−1 (X. Wang et al. Reference Wang, Shi, Zhao and Tang2015), approximately half of that in the modern ocean (102 nmol L−1), implying that the oceanic Mo reservoir was higher than in earlier times (e.g. 10–20 nmol L−1 in the Mesoproterozoic) (Scott et al. Reference Scott, Lyons, Bekker, Shen, Poulton, Chu and Anbar2008). Aqueous Mo in the Nanhua Basin, though precipitating in deep euxinic water, was replenished from the open ocean whose Mo reservoir was evidently increased compared with that of the Mesoproterozoic. Because U precipitates at the Fe (II) – Fe (III) boundary (i.e. anoxic–suboxic boundary) while Mo precipitates in sulphidic water or sediments, the low Mo-EF (from 0.7 to 38) and low Mo/U weight ratios (from 0.1 to 1.4, which are much lower than the modern seawater value of 3.1) of the samples from Intervals I and III reflect suboxic or anoxic marine conditions in the Cambrian Fortunian Stage to lower Stage 2 and in upper Stage 3. In contrast, Interval II has significantly high Mo-EF (from 57.3 to 242) and Mo/U weight values (up to 2.3), suggesting that the marine environment was briefly in euxinic conditions from upper Stage 2 to lower Stage 3.

Figure 5. (A) Mo-EF vs U-EF covariation and (B) Mo vs TOC covariation patterns of Daotuo drill core samples. SW: modern seawater. Modified from Algeo & Tribovillard (Reference Algeo and Tribovillard2009) and Algeo & Lyons (Reference Algeo and Lyons2006), respectively.
Mo–TOC covariation patterns of the studied samples from the three intervals are shown in Figure 5B. The Mo/TOC ratios of the Black Sea (a strong restricted basin), Framvaren Fjord and Cariaco Basin (an Fe–Mn particulate shuttle area) are 4.5 ± 1, 9 ± 2 and 25 ± 5, respectively (Algeo & Lyons, Reference Algeo and Lyons2006). The Mo/TOC values of Interval I samples range from 1.44 to 16.6 ppm %−1 (averaging 10.2 ppm %−1), those of Interval II from 2.7 to 20.0 ppm %−1 (averaging 11.8 ppm %−1), and those of Interval III from 0.88 to 50.4 ppm %−1 (averaging 9.68 ppm %−1). Cross-plots of Mo content with TOC content of Interval I and II samples are distributed in the area between the covariation lines of Cariaco Basin and Framvaren Fjord sediments, indicating a weakly restricted marine environment during deposition of the two intervals. In this figure, a few samples of Interval III are plotted in the area below the Black Sea line, suggesting a more restricted environment in this period than in the previous two intervals. In general, the mean Mo/TOC value of the studied samples is c. 10 ppm %−1, much higher than that of the Black Sea (4.5 ± 1 ppm %−1), suggesting a weakly restricted sedimentary environment on the Yangtze shelf margin area in the early Cambrian era.
5.b. Marine sulphate concentration of the Yangtze margin area during the early Cambrian
Several factors control the sulphur isotopic compositions of pyrites precipitating from anoxic seawater into black shales or other reduced sediments. One factor is the sulphate concentration in the seawater, which mainly controls the MSR fractionation in seawaters. Laboratory experiments demonstrate that fractionation caused by MSR decreases with falling sulphate concentration when sulphate concentration in seawater is lower than 2 mmol L−1 (Harrison & Thode, Reference Harrison and Thode1958; Canfield, Reference Canfield2001; Habicht et al. Reference Habicht, Salling, Thamdrup and Canfield2005) and that the MSR fractionation is large when sulphate concentration in the seawater is ample (much higher than 2 mmol L−1). The ocean sulphate concentration effectively reflects the oxygen level of the Earth's surface because the primary source of sulphate in seawater is the oxidative weathering of pyrite on the continents (Canfield, Reference Canfield2004) and because a greater total of anoxic bottom water areas in the ocean provides more opportunities to sequester sulphate in the seawater through MSR. The other factor controlling sulphur isotopic compositions of pyrites precipitating in sediments is the isotopic composition of the oceanic sulphate reservoir, which depends on the isotopic composition of sulphur input and output (Strauss, Reference Strauss1999). In a relatively restricted marine environment, the two different sulphate origins, i.e. the riverine sulphate and the oceanic sulphate reservoirs, may result in diverse isotopic compositions of local seawater sulphate in various nearshore areas and thus may generate variable pyrite sulphur isotopic compositions in different sedimentary environments (Feng et al. Reference Feng, Li, Huang, Chang and Chu2014). Riverine sulphate generally has a low S-isotope composition (~+8 ‰) (Berner & Berner, Reference Berner and Berner1996). The large variation range and relatively strong fluctuation of the δ34Spy values of the samples in this study (from −16.8 ‰ to 19.2 ‰) cannot be explained by simple sulphate source exchange. Other factors, such as the oxidative portion of the microbial sulphur cycle and sulphur disproportionation, can result in a substantial increase in sulphur fractionation relative to primary MSR, while these two processes require a relatively oxic condition (Jones & Fike, Reference Jones and Fike2013). In our study area, an anoxic–euxinic marine redox condition and a weakly restricted environment are supported by trace element proxies (Mo-EF, U-EF covariation and Mo–TOC covariation). These data suggest that marine sulphate concentration was the most important factor for sulphur fractionation during MSR for the samples in this study.
Based on the empirical relationship between seawater sulphate concentrations and Δ34SCAS-PY analysed for 81 modern depositional systems, Algeo et al. (Reference Algeo, Luo, Song, Lyons and Canfield2015) proposed an MSR-trend method to quantitatively estimate the mean global seawater SO4 2− concentration in a specific geological period: log[SO4 2−] = (log (Δ34SCAS-PY) – 1.10)/0.42. The upper and lower uncertainty limits for estimates of marine [SO4 2−] based on this relationship are log[SO4 2−] = (log (Δ34SCAS-PY) – 1.18)/0.40 and log[SO4 2−] = (log (Δ34SCAS-PY) – 1.02)/0.44, respectively (Algeo et al. Reference Algeo, Luo, Song, Lyons and Canfield2015). The δ34S values of the global seawater sulphate during the terminal Neoproterozoic to the early Cambrian were estimated between 25 ‰ and 35 ‰ (Strauss, Reference Strauss1997; Shen et al. Reference Shen, Zhao, Chu and Lei1998; Goldberg, Poulton & Strauss, Reference Goldberg, Poulton and Strauss2005). Based on these estimated seawater sulphate δ34S values, our δ34S results for pyrite and the MSR-trend method, we calculated the seawater sulphate concentrations in the three intervals (Table 3). Because of the uncertainties in Δ34SCAS-PY and S-isotope fractionation associated with MSR, minimum Δ34SCAS-PY values with the upper uncertainty limit equation are used to estimate minimum marine [SO4 2−], and maximum Δ34SCAS-PY values with the lower uncertainty limit equation are used to estimate maximum marine [SO4 2−]. Assuming that pyrite formed in sediment pore water during early diagenesis would yield higher δ34Spy than pyrite formed in seawater, as depletion of SO4 2− occurring in the pore water would cause a decrease in fractionation between SO4 2− and S2− during MSR. Thus, the calculated Δ34SCAS-PY values are likely minimum values if part of the pyrites was formed by MSR occurring in the sediments (e.g. Interval III), and the resulting seawater sulphate estimates are minimum values. Because, in periods of euxinic marine environments, pyrites were formed largely in sulphidic seawater, and, in periods of non-euxinic environments, pyrites were mainly formed in sediments, only δ34S values of pyrites in euxinic black shales could reflect seawater sulphate concentrations. However, seawater sulphate concentrations could be higher than calculated seawater sulphate concentrations calculated from δ34S values of pyrites in non-euxinic sedimentary rocks.
Table 3. Approximate estimated marine sulphate concentrations on the Yangtze shelf margin in the early Cambrian with the MSR-trend method

Estimates of marine sulphate concentration are shown in Table 3; the studied samples from Interval I (cherts from the Liuchapo Formation) of the Daotuo drill core have relatively high δ34Spy (up to 15.9 ‰; mean value of 9.4 ‰), indicating relatively low oceanic sulphate concentration (c. 1.7–5.4 mmol L−1; the lowest value is 0.3 mmol L−1) and MSR in sediment pore water with anoxic bottom water in the Cambrian Fortunian and lower Stage 2 (Fig. 6A). Black shales in Interval II of the lower Jiumenchong Formation show evidently low δ34Spy (down to −16.8 ‰; mean value of −1.3 ‰) and high Mo concentrations (up to 115.6 ppm), indicating relatively high marine sulphate concentration (mainly c. 5.8–12.4 mmol L−1 with the highest up to 37.8 mmol L−1). Strong MSR occurred in the seawater, probably due to the increased riverine sulphate input and a pulse of shrinking total area of anoxic bottom waters in global oceans (Chen et al. Reference Chen, Ling, Vance, Shields-Zhou, Zhu, Poulton, Och, Jiang, Li, Cremonese and Archer2015). A large quantity of Mo, U and other redox-sensitive metallic elements also precipitated in this euxinic seawater environment, forming the euxinic black shale bearing the polymetallic enrichment layer (Fig. 6B). The sulphur isotopes in Interval III returned to relatively heavy except for two samples and then fluctuated with time in this period (mean δ34Spy is 8.0 ‰). Such isotopic variation may reflect variable sulphate concentration in the seawater and relatively low average concentration of marine sulphate (2.0–6.1 mmol L−1; the lowest value is 0.1 mmol L−1), if the sulphur isotopic compositions of the seawater sulphate reservoir remained nearly unchanged from slope to basin in the Nanhua Basin during this short period (Feng et al. Reference Feng, Li, Huang, Chang and Chu2014). The deep marine environment became anoxic again without significant Mo precipitation, and MSR mainly occurred within the sediments (Fig. 6C). It should be noted that pyrites are mainly formed in sediments in Intervals I and III, and the estimates of marine sulphate concentrations in these periods are probably lower than the actual values. Only the estimates derived from the pyrites formed in sulphidic seawater in Interval II could reflect the actual marine sulphate concentrations.

Figure 6. Schematic scenarios for geochemistry cycle in the early Cambrian ocean on the Yangtze shelf margin with three intervals: (A) Cambrian Fortunian and lower Stage 2, the relatively deep chemocline which separates anoxic deep water from oxic surface water and the oxic photic zone. (B) Upper Stage 2 and lower Stage 3, the rise of the chemocline and the expansion of the mid-depth sulphidic zone on the Yangtze margin (based on Li et al. (Reference Li, Cheng, Algeo and Xie2015)) with the anoxic, or even euxinic, photic zone through upwelling deep water. (C) Middle and upper Stage 3, the depression of the mid-depth sulphidic zone and the relatively shallow chemocline separating oxic surface water and anoxic deep water with a decreased nitrate reservoir.
5.c. Marine nitrogen cycle of the Yangtze margin area during the early Cambrian
Early diagenesis and thermal maturation during burial of organic matter may affect nitrogen isotopic compositions of bulk samples. To evaluate whether the nitrogen isotopic values reflect the primary marine information or not, we examined the relationships between total organic carbon (TOCdecarb), total nitrogen (TNdecarb), C/Ndecarb molar ratios and δ15N values in the decarbonated samples (Fig. 4C, D, E, F). The relatively clear linear correlation between TNdecarb and TOCdecarb (Fig. 4D) indicates that the nitrogen in the sediments is derived from two components, an organic component from primary production and an inorganic clay-bound nitrogen component originating from digestion of organic matter by microorganisms, represented by the intercept on the TN axis (Calvert, Reference Calvert2004; D. Wang et al. Reference Wang, Ulrich, Ling, Guo, Shields-Zhou, Zhu and Yao2015). The mean C/N molar ratio in modern marine sediments is c. 6 (Redfield, Reference Redfield and Hill1963), while Precambrian samples often have much higher C/N molar ratios (>100) due to the loss of N during diagenesis through degradation of organic matter via microorganisms (e.g. D. Wang et al. Reference Wang, Ulrich, Ling, Guo, Shields-Zhou, Zhu and Yao2015; Wei et al. Reference Wei, Wang, Li, Ling, Chen, Wei, Zhang, Zhu and Yan2016). The studied samples have an average C/N molar ratio of 21.3 and show a clear linear correlation between C/N and TOCdecarb (Fig. 4C), suggesting weak loss of organic nitrogen from the studied samples during their early diagenesis (Cremonese et al. Reference Cremonese, Shields-Zhou, Struck, Ling, Och, Chen and Li2013). The δ15Nbulk values of sediments normally increase as organic matter degrades, usually with preferential loss of 14N during diagenesis (Pinti et al. Reference Pinti, Hashizume, Orberger, Gallien, Cloquet and Massault2007; Thomazo, Ader & Philippot, Reference Thomazo, Ader and Philippot2011). There is no obvious linear correlation between δ15Nbulk and TOC or TNdecarb (Fig. 4E, F), suggesting that the nitrogen isotopes of the studied samples have not changed significantly with the nitrogen loss of the organic matter. In summary, the nitrogen isotopic data of the studied samples principally record the primary marine geochemical information of the Yangtze shelf margin.
Based on nitrogen isotopic compositions of the studied bulk samples from the Daotuo drill core, combined with trace elements of the bulk samples and sulphur isotopes in pyrites, we discuss the nitrogen cycles for the early Cambrian Yangtze shelf margin as follows.
5.c.1. Cambrian Fortunian and lower Stage 2 (Interval I)
In the stratified redox ocean, 15N-enriched NO3 − would occur in the oxic or dysoxic surface seawater, while 15N-depleted NH4 + would occur in the anoxic deep seawater (Higgins et al. Reference Higgins, Robinson, Husson, Carter and Pearson2012; Ader et al. Reference Ader, Sansjofre, Halverson, Busigny, Trindade, Kunzmann and Nogueira2014). For the Cambrian Fortunian and lower Stage 2 (Interval I), the low Mo concentrations and relatively high δ34Spy values of this study and Fe speciation data available from the literature (Och et al. Reference Och, Shields-Zhou, Poulton, Manning, Thirlwall, Li, Chen, Ling, Osborn and Cremonese2013; Feng et al. Reference Feng, Li, Huang, Chang and Chu2014; Chen et al. Reference Chen, Ling, Vance, Shields-Zhou, Zhu, Poulton, Och, Jiang, Li, Cremonese and Archer2015) indicate that the Nanhua Basin was stratified, with oxygenated surface seawater overlying ferruginous deep seawater. The δ15Nbulk values of the studied samples in this interval vary from −1.2 ‰ to 3.5 ‰. The high value of 3.5 ‰ indicates that primary producers may have assimilated nitrogen from the seawater nitrate reservoir, and thus the photic zone may have been oxic or dysoxic with a relatively deep chemocline. Meanwhile, the nitrate reservoir may have been not very large, and the nitrogen sources of phytoplankton may have been both nitrate and ammonium under conditions of equilibrium among nitrogen assimilation, N2 fixation and denitrification (Cremonese et al. Reference Cremonese, Shields-Zhou, Struck, Ling, Och, Chen and Li2013). In summary, the deep seawater in the Yangtze shelf margin area was anoxic or ferruginous, while the surface seawater was oxic or dysoxic during the Cambrian Fortunian and lower Stage 2, as shown schematically in Figure 6A.
5.c.2. Upper Stage 2 and lower Stage 3 (Interval II)
This interval features a polymetallic sulphide-rich layer that was deposited from the mid-depth zone of sulphidic seawater on the shelf margin, sandwiched between oxic surface water and ferruginous deep water, produced by MSR due to an increasing sulphate supply, possibly from continental weathering as a result of increased riverine sulphate input (Guo et al. Reference Guo, Shields, Liu, Strauss, Zhu, Pi, Goldberg and Yang2007; Lehmann et al. Reference Lehmann, Nägler, Holland, Wille, Mao, Pan, Ma and Dulski2007; Xu et al. Reference Xu, Lehmann, Mao, Nägler, Neubert, Böttcher and Escher2012; Och et al. Reference Och, Shields-Zhou, Poulton, Manning, Thirlwall, Li, Chen, Ling, Osborn and Cremonese2013; Feng et al. Reference Feng, Li, Huang, Chang and Chu2014; Li et al. Reference Li, Cheng, Algeo and Xie2015). Some of the studied samples in this interval show high molybdenum concentrations (>100 ppm) and low δ34Spy (<−15 ‰), which is consistent with this sulphidic zone developed in the basin connecting well with the open ocean and enhanced oxygenation of the global ocean inferred from data of other redox proxies such as Mo isotopes (Chen et al. Reference Chen, Ling, Vance, Shields-Zhou, Zhu, Poulton, Och, Jiang, Li, Cremonese and Archer2015).
This interval has the lowest δ15Nbulk value (−3.2 ‰) of the studied core section. Such a low δ15Nbulk value in the sediments may have occurred due to several potential mechanisms. One possible mechanism is that the primary producers in the shelf margin assimilated 15N-depleted NH4 + upwelling from anoxic deep seawater where the nitrogen reservoir was dominated by NH4 + (Higgins et al. Reference Higgins, Robinson, Husson, Carter and Pearson2012). The upwelling anoxic seawater following a widespread transgression event in the early Cambrian (Steiner et al. Reference Steiner, Zhu, Weber and Geyer2001) may also have driven shallowing of the chemocline. Another potential mechanism is that the photic zone became anoxic or euxinic due to a rise of the chemocline, and thus N2 fixation prevailed through green or purple sulphur bacteria, resulting in the large nitrogen isotopic fractionation during azofication (Ohkouchi et al. Reference Ohkouchi, Nakajima, Okada, Ogawa, Suga, Oguri and Kitazato2005; Cremonese et al. Reference Cremonese, Shields-Zhou, Struck, Ling, Och, Chen and Li2013). Different nitrogenases also had varying influences on nitrogen isotopic compositions of organisms. Zhang et al. (Reference Zhang, Sigman, Morel and Kraepiel2014) reported that diazotrophs using molybdenum nitrogenases would produce very small nitrogen isotope fractionation, but vanadium-only or iron-only nitrogenases would produce fixed nitrogen with significantly lower δ15N (−6 to −7 ‰), resulting in the low δ15N (<−2 ‰) recorded in sediments. In euxinic seawater, molybdenum precipitates rapidly in sediments with sulphides and organic matter, decreasing the concentration of molybdenum in the local seawater significantly, especially in a restricted marine environment (Scott et al. Reference Scott, Lyons, Bekker, Shen, Poulton, Chu and Anbar2008; Reinhard et al. Reference Reinhard, Planavsky, Robbins, Partin, Gill, Lalonde, Bekker, Konhauser and Lyons2013). Meanwhile, vanadium concentration is lowered as well, although not as much as the decreasing extent of molybdenum; thus, there is still reactive vanadium in the euxinic seawater (Emerson & Huested, Reference Emerson and Huested1991). In contrast, anoxic conditions generally tend to increase iron bioavailability, as Fe (II)-sulphides are more soluble than Fe (III)-oxides. Therefore, euxinic conditions would be favourable to V- and/or Fe- nitrogenases as important N2-fixed enzymes rather than Mo-nitrogenases, resulting in large nitrogen isotope fractionations (Anbar & Knoll, Reference Anbar and Knoll2002; Zhang et al. Reference Zhang, Sigman, Morel and Kraepiel2014). The significantly low δ15Nbulk in this interval is probably the end product of all these factors. The above data and discussion suggest a decrease of the seawater oxygenation in the local Yangtze shelf margin area in the period of the upper Cambrian Stage 2 and lower Stage 3, caused by an enhancement in the MSR process as a result of increasing local marine sulphate concentration, evidenced by the decrease of δ34Spy values noted above (Table 3). The black shales at the top of Interval II show low Mo concentrations, δ34Spy values of 0–5 ‰ and δ15Nbulk values between +1 ‰ and +2 ‰. These data indicate recession of the previous euxinic seawater and a return to anoxic (ferruginous) conditions of the local marine environment after lower Cambrian Stage 3.
5.c.3. Middle and upper Stage 3 (Interval III)
Geochemical data of the studied samples in the middle and upper Cambrian Stage 3 (Interval III) indicate that a widespread euxinic marine environment gradually shrank with the regression, which is supported by Feng et al. (Reference Feng, Li, Huang, Chang and Chu2014) and Jin et al. (Reference Jin, Li, Peng, Cui, Shi, Zhang, Luo and Xie2014). Both Mo and U concentrations of the samples in this interval are much lower than those in Interval II, suggesting an anoxic but not euxinic marine environment on the slope zone of the Yangtze shelf margin. The δ15Nbulk values of the samples in this interval vary from +1.7 ‰ to −1.7 ‰ with a descending trend from the middle to the upper Jiumenchong Formation, which probably reflects the decrease of the nitrate reservoir and the falling oxygenation level of the Yangtze shelf-margin area in this period. With consumption of the local marine nitrate reservoir due to the preceding euxinic event during the upper Cambrian Stage 2 and lower Stage 3, the nitrogen sources used by organisms in the middle and upper Cambrian Stage 3 would be mainly from atmospheric N2 through azofication or partly from ammonium from the deep seawater, resulting in the low δ15Nbulk values in this period compared with those of the Cambrian Fortunian and lower Stage 2. Meanwhile, TOC and TN contents in this period also appear in a decreasing trend, probably reflecting reduction of primary production and nitrogen assimilation in the shelf margin as a result of the low N2-fixation efficiency. In the period after the lower Cambrian Stage 3, sea level fluctuated frequently (Haq & Schutter, Reference Haq and Schutter2008). With probably an event of sea level fall after the transgression in the lower Cambrian Stage 3 (Interval II), the chemocline shifted below the photic zone. Nevertheless, the anoxic zone on the Yangtze shelf margin in this period was larger than that in the Cambrian Fortunian and lower Stage 2, which possibly resulted from an increase of the restriction extent of the Nanhua Basin with the regression. The decreasing trend of δ15Nbulk from the middle to the upper Cambrian Stage 3 (Interval III) may have been caused by enhancement of the anoxic extent of the Yangtze shelf margin area as another local anoxic event in this period (D. Wang et al. Reference Wang, Ulrich, Ling, Guo, Shields-Zhou, Zhu and Yao2015), which may have resulted in the extinction of Niutitang sponge biota at Cambrian Stage 3 (Zhu et al. Reference Zhu, Strauss and Shields2007). However, the redox state levels of marine environments in other regions than the Yangtze shelf margin and of the global ocean in the period of the Cambrian middle to upper Stage 3 require further study in the future.
5.d. Possible link between ocean geochemical cycles and biological evolution
Phosphorus and nitrogen are both essential nutrients for living organisms. Because of the enhanced marine P reservoir in the terminal Neoproterozoic, the previously P-limited marine environment may have transformed into a N-limited marine environment in the early Cambrian (e.g. Kikumoto et al. Reference Kikumoto, Tahata, Nishizawa, Sawaki, Maruyama, Shu, Han, Komiya, Takai and Ueno2014). Biogenic nitrogen fixation provides the main input of nitrogen for the Earth ecosystem, and metallic ions of Mo, Fe and V are essential for the nitrogenases. In euxinic and ferruginous environments with very low Mo concentrations in seawater, vanadium and iron ‘alternative’ nitrogenases produce fixed nitrogen with significantly lower δ15Nbulk than that produced by molybdenum nitrogenases in oxic and suboxic environments (Zhang et al. Reference Zhang, Sigman, Morel and Kraepiel2014). The production efficiency of N2 fixation by vanadium or iron nitrogenases is also much lower than that by molybdenum nitrogenases. Recent laboratory experimental results indicate that when Mo concentration is below ~5 % of the present Mo concentration in the ocean, the rate of N2 fixation in cultured cyanobacteria begins to decrease (Zerkle et al. Reference Zerkle, House, Cox and Canfield2006). Efficient molybdenum precipitation in euxinic seawater reduces molybdenum concentration in local seawater and thus reduces the N2 fixation rate, causing a decrease of primary production and consequently a decrease of TN and TOC in sediments. This bio-inorganic bridge linking Mo to N bioavailability would act as a negative feedback (Fig. 7) limiting the spatial and temporal extent of euxinic conditions because the bacterially mediated reduction of sulphate to sulphide would be depressed by the decrease of organic matter (Scott et al. Reference Scott, Lyons, Bekker, Shen, Poulton, Chu and Anbar2008). After temporary euxinic conditions (upper Cambrian Stage 2 and lower Stage 3), the seawater of the Yangtze shelf margin returned to anoxic or ferruginous (middle and upper Stage 3). As hydrogen sulphide was pernicious to aerobic respiration, the transition from sulphidic to ferruginous deep seawater detoxified the basin margin, providing expanded opportunities for eukaryote diversification. The Cambrian Explosion may have benefited from such an environmental transition occurring in the Nanhua Basin as a representative in the global ocean in the early Cambrian (e.g. Chengjiang biota or Niutitang sponge biota).

Figure 7. Schematic depiction for the biogenic negative feedback on seawater redox in the Yangtze shelf margin area. Stratified marine redox model is based on Li et al. (Reference Li, Cheng, Algeo and Xie2015).
6. Summary and conclusions
Trace elements, sulphur isotopes and nitrogen isotopes of the Daotuo drill core samples (the upper Liuchapo Formation and the entire Jiumenchong Formation) in Songtao County, NE Guizhou Province, were analysed to reconstruct the marine environmental and biological evolution of the Yangtze shelf margin during the early Cambrian. Redox conditions varied among three intervals during the period from the early Cambrian Fortunian to Stage 3 (Fig. 3). Seawater became sulphidic through microbial sulphur reduction (MSR), and the photic zone was anoxic, even euxinic, in the upper Stage 2 and lower Stage 3. Seawater returned to anoxic or ferruginous conditions after the recession of sulphidic conditions in the middle and upper Stage 3. These processes were recorded and reflected by molybdenum and uranium concentrations, sulphur isotopes and nitrogen isotopes. Furthermore, decreasing δ15Nbulk values in the middle and upper Stage 3 suggest a gradually shrinking local marine nitrate reservoir in this period, which probably reflected the oxygenation level of the Yangtze shelf margin area falling in this period. The change of marine environment may have exerted significant influence on biological activity, affecting the nitrogen cycle in the Yangtze shelf margin area, as recorded by nitrogen isotopes in the bulk samples. Meanwhile, biological activity may also have had significant negative feedback on the local sulphidic seawater. The sulphidic seawater during the early Cambrian upper Stage 2 decayed partly due to depression in primary production as a result of the rapid deposition of molybdenum and the consequent decrease of N2 fixation efficiency. In addition to biological activity, the change of marine redox environments between anoxic (ferruginous) and euxinic (sulphidic) conditions might be related to other factors, such as fluctuation of sea level and continental weathering flux; thus, the connections between the redox state levels of the marine environment and these factors merit further study in the future.
Acknowledgements
We thank Nina Zhao and Fuqiang Shi for assistance with the field work; Prof. Zhaohui Zhang and Qian Liu for chemical analyses; and Prof. Thomas J. Algeo and Prof. Chao Li for helpful suggestions. This study was funded by the National Basic Research Program of China (2013CB835004) and a National Natural Science Foundation of China (NSFC) program (41230102).