Introduction
In the last several years, natural layered double hydroxides (LDHs) have received considerable attention, owing to their interest from the viewpoint of mineralogy and material sciences. The recent advances include: nomenclature of the hydrotalcite supergroup (Mills et al., Reference Mills, Christy, Génin, Kameda and Colombo2012a); single-crystal structure refinements of takovite-3R (Mills et al., Reference Mills, Whitfield, Kampf, Wilson, Dipple, Raudsepp and Favreau2012d), cualstibite-1M (Kolitsch et al., Reference Kolitsch, Giester and Pippinger2013) and zincalstibite-9R (Mills et al., Reference Mills, Christy, Kampf, Housley, Favreau, Boulliard and Bourgoin2012b); Rietveld refinement and re-examination of stichtite-3R and -2H (Mills et al., Reference Mills, Whitfield, Wilson, Woodhouse, Dipple, Raudsepp and Francis2011); discovery of a new mineral, omsite (Mills et al., Reference Mills, Kampf, Housley, Favreau, Pasero, Biagioni, Merlino, Berbain and Orlandi2012c); definition of a hydrotalcite neotype material as found in both 2H and 3R polytypes (Mills et al., Reference Mills, Christy and Schmitt2016); re-investigation of jamborite, which demonstrated that it is not a LDH (Bindi et al., Reference Bindi, Christy, Mills, Ciriotti and Bittarello2015); redefinition of fougérite, Fe2+4Fe3+2(OH)12CO3·3H2O, and discovery of two new minerals species, trébeurdenite, Fe2+2Fe3+4O2(OH)10[CO3]·3H2O and mössbauerite, Fe3+6O4(OH)8[CO3]·3H2O (Génin et al., Reference Génin, Guérin, Herbillon, Kuzmann, Mills, Morin, Ona-Nguema, Ruby and Upadhyay2013, Reference Génin, Christy, Kuzmann, Mills and Ruby2014a,Reference Génin, Mills, Christy, Guérin, Herbillon, Kuzmann, Ona-Nguema, Ruby and Upadhyayb) and the observed correlation between the d value and the M 2+:M 3+ ratios for quintinite, hydrotalcite and pyroaurite (Zhitova et al., Reference Zhitova, Krivovichev, Pekov, Yakovenchuk and Pakhomovsky2016b).
Quintinite, [Mg4Al2(OH)12](CO3)(H2O)3, is a member of the quintinite group, which is a subdivision of the hydrotalcite supergroup (Mills et al., Reference Mills, Christy, Génin, Kameda and Colombo2012a). Quintinite-group minerals are defined as species with the M 2+:M 3+ ratio of 2:1 that contain interlayer CO32– or Cl– anions and H2O molecules (Mills et al., Reference Mills, Christy, Génin, Kameda and Colombo2012a). In our previous papers of the current series, we reported the crystal structures of three quintinite polytypes from the Kovdor phoscorite-carbonatite complex: 2T-3с [6R] (originally published as quintinite-2H-3c [6R], Krivovichev et al., Reference Krivovichev, Yakovenchuk, Zhitova, Zolotarev, Pakhomovsky and Ivanyuk2010a), 1M (Krivovichev et al., Reference Krivovichev, Yakovenchuk, Zhitova, Zolotarev, Pakhomovsky and Ivanyuk2010b) and 2H (Zhitova et al., Reference Zhitova, Yakovenchuk, Krivovichev, Zolotarev, Pakhomovsky and Ivanyuk2010). Crystal chemistry and information storage capacity of quinitinite-2T-3c [6R], 1M and 2H were reviewed by Krivovichev et al. (Reference Krivovichev, Yakovenchuk, Zhitova and Krivovichev2012b). Quintinite-1M was also described from rodingites at the Bazhenovskoe deposit (Middle Ural, Russia) (Krivovichev et al., Reference Krivovichev, Antonov, Zhitova, Zolotarev, Krivovichev and Yakovenchuk2012a) and cavities of metasomatically altered gabbro at Mariinskoe deposit (Middle Ural, Russia) (Zhitova et al., Reference Zhitova, Ivanyuk, Krivovichev, Yakovenchuk, Pakhomovsky and Mikhailova2016a). We note that the structural model of the 1M polytype proposed by us for quintinite-1M was used by Jayanthi et al. (Reference Jayanthi, Nagendran and Kamath2015) for synthetic LDHs and applied for the Rietveld structure refinement of the synthetic analogue of zaccagnaite, [Zn4Al2(OH)12][(CO3)(H2O)3] (Marappa and Kamath, Reference Marappa and Kamath2015).
Crystal structures of LDHs are based upon octahedral metal-hydroxide layers [M 2+1–xM 3+x(OH)2], (where M2+ = Mg, Ni, Mn, Fe, Zn, Cu and Ca; M3+ = Al, Fe, Cr, Mn and Co) alternating with interlayers represented by anion groups (CO3, OH, Cl, SO4 and Sb(OH)6), H2O molecules and, sometimes, with cationic complexes such as [Ca(H2O)6]2+, [Na(H2O)6]+ or [Mg(H2O)6]2+ (Cavani et al., Reference Cavani, Trifiro and Vaccari1991; Rives, Reference Rives2001; Evans and Slade, Reference Evans, Slade, Duan and Evans2006; Mills et al., Reference Mills, Christy, Génin, Kameda and Colombo2012a). LDHs are commonly observed in different structural modifications recognized as polytypes and usually denoted according to the Ramsdell system (Guinier et al., Reference Guinier, Bokij, Boll-Dornberger, Cowley, Durovic, Jagodzinski, Krishna, DeWolff, Zvyagin, Cox, Goodman, Hahn, Kuchitsu and Abrahams1984). The most common layer stacking sequences for LDHs are rhombohedral (known as the 3R 1 polytype) and hexagonal (known as the 2H 1 polytype) (Fig. 1).
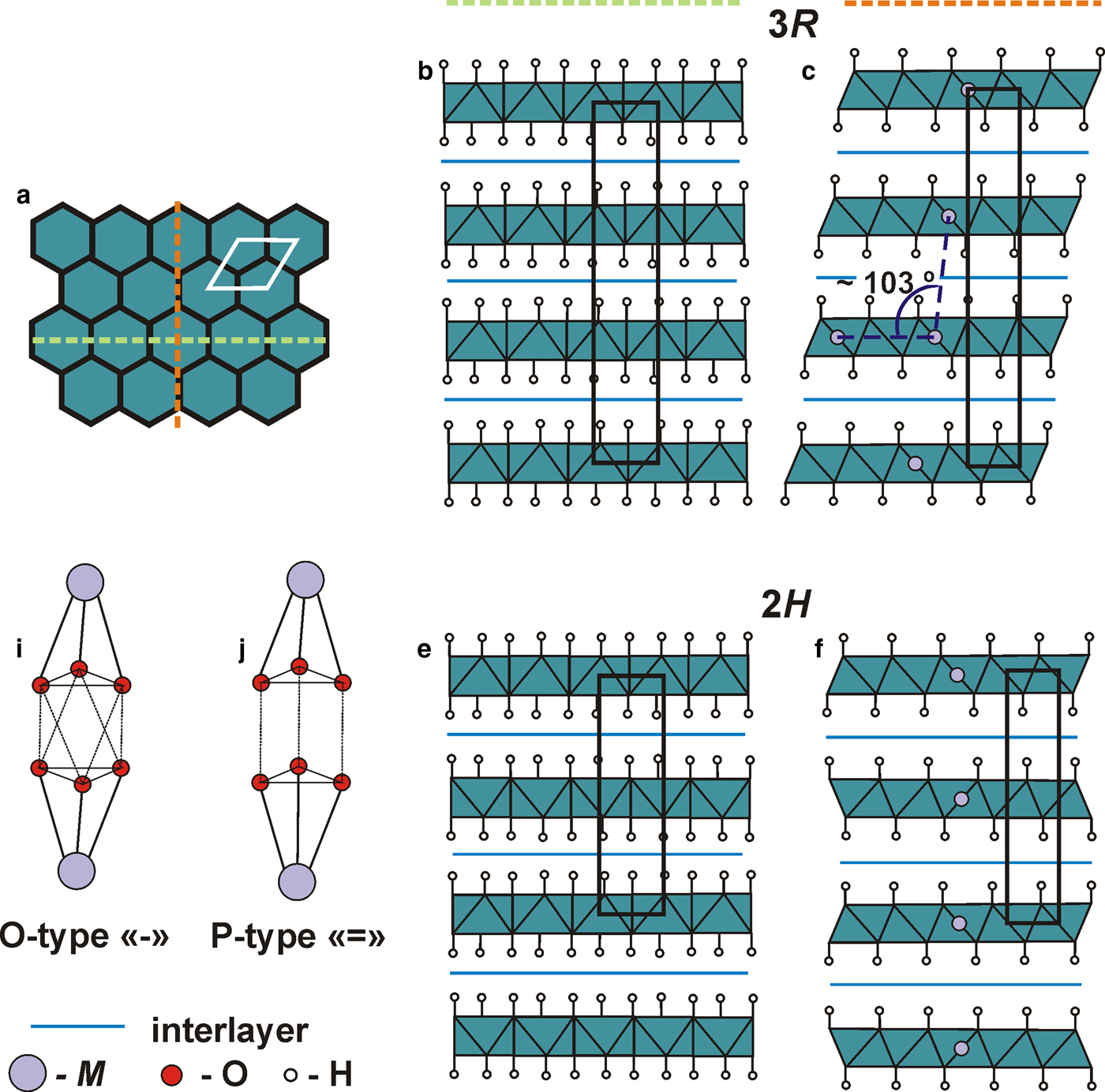
Fig. 1. Crystal structures of 3R and 2H polytype within the xy plane (a); and in two different orientations in respect to brucite-type layer: along orange (b, e) and green (c, f) lines. Two types of interlayer: octahedral (i) and prismatic (j).
A detailed approach to the characterization of LDH polytypes was proposed by Bookin and Drits (Reference Bookin and Drits1993) (see also: Drits and Bookin, Reference Drits, Bookin and Rives2001). In the framework of this proposal, positions of cations and hydroxyl groups are considered as positions of spheres in closest packing. Positions of hydroxyl groups are symbolized as A, B, C, whereas cations occupy the a, b, c sites within the hexagonal layer. For instance, a single layer may be denoted as AbC. Special attention is paid to the relative position of adjacent metal-hydroxide layers characterized by the positions of hydroxyl groups from upper and lower metal-hydroxide layers. There are two possible types of interlayers: (1) hydroxide ions of adjacent layers are of the same kind, this type of interlayer corresponds to a trigonal prism and is denoted as P (=); (2) hydroxide ions of adjacent layers are of a different kind, forming a distorted octahedron (O-type interlayer (−)) (Fig. 1). One-, two- and three-layered polytypes were summarized by Mills et al. (Reference Mills, Christy, Génin, Kameda and Colombo2012a). In the original scheme by Bookin and Drits (Reference Bookin and Drits1993), the polytypes were identified by the positions of hydroxyl groups only, e.g. …=AB = BC = CA = … for the 3R 1 polytype and …=AC = CA = … for the 2H 1 polytype. Krivovichev et al. (Reference Krivovichev, Yakovenchuk, Zhitova, Zolotarev, Pakhomovsky and Ivanyuk2010a,Reference Krivovichev, Yakovenchuk, Zhitova, Zolotarev, Pakhomovsky and Ivanyukb) demonstrated that the same type of stacking (polytype) may correspond to different structures due to the different degrees and patterns of cation ordering within octahedral layers, which makes consideration of cation positions an important issue in identification of different polytypes.
Previously published structural models of quintinite appeared to be helpful for the characterization of hydrotalcite-supergroup members (Lozano et al., Reference Lozano, Rossi, La Iglesia and Matesanz2012; Mills et al., Reference Mills, Christy, Génin, Kameda and Colombo2012a; Theiss et al., Reference Theiss, López, Frost and Scholz2015); synthetic layered double hydroxides (Zhang and Evans, Reference Zhang and Evans2012; Jayanthi and Kamath, Reference Jayanthia and Kamath2013, Reference Jayanthi and Kamath2016; Radha et al., Reference Radha, Jayanthi, Breu and Kamath2014; Jayanthi et al., Reference Jayanthi, Nagendran and Kamath2015; Marappa and Kamath, Reference Marappa and Kamath2015); their crystal structure simulation and analysis (Radha and Kamath, Reference Radha and Kamath2012; Richardson, Reference Richardson2013; Ghamami et al., Reference Ghamami, Golzani and Lashgari2016; Tsukanov and Psakhie, Reference Tsukanov and Psakhie2016).
It is important to note that Mg–Al–CO3 LDHs are the most common in nature. At present, in addition to the localities mentioned above, quintinite had been described from Mont Saint-Hilaire (Quebec, Canada) and Jacupiranga (San Paulo, Brazil) (Arakcheeva et al., Reference Arakcheeva, Pushcharovskii, Atencio and Lubman1996; Chao and Gault, Reference Chao and Gault1997). The Kovdor complex has the most diverse suite of quintinite polytypes. In this paper, we report on yet another two polytypes of quintinite found in the Kovdor complex and consider structural evolution of polytypes from the viewpoint of structural complexity.
Occurrence
The Kovdor complex of peridotite, foidolite–melilitolite, phoscorite, carbonatite and related metasomatic rocks is situated in the southwestern part of the Murmansk Region, Russia. It is a ring multiphase volcano-plutonic complex that intruded through Archean biotite (granite) gneiss ~400 Ma (Ivanyuk et al., Reference Ivanyuk, Yakovenchuk and Pakhomovsky2002, Reference Ivanyuk, Kalashnikov, Pakhomovsky, Mikhailova, Yakovenchuk, Konopleva, Sokharev, Bazai and Goryainov2016; Mikhailova et al., Reference Mikhailova, Kalashnikov, Sokharev, Pakhomovsky, Konopleva, Yakovenchuk, Bazai, Goryainov and Ivanyuk2016). The complex has a distinctly concentrically zoned structure with peridotite located in the central part of the massif and surrounded by the concentric zones of foidolite–melilitolite and related metasomatic rocks: diopsidite, phlogopitite, melilite-, monticellite-, vesuvianite- and andradite-rich skarn-like rocks formed after peridotite, fenitized gneiss and fenite after gneiss.
Along the western contact of peridotite and foidolite–melilitolite intrusions, a concentrically zoned pipe of phoscorite and carbonatite intrudes the complex. These rocks have a magmatic origin and consist of magnetite, forsterite, apatite and carbonates (Le Maitre, Reference Le Maitre2002), forming a continuous series, where carbonatite contains ≥ 50 modal % of carbonate (Streckeisen, Reference Streckeisen1980). The outer zone of the phoscorite-carbonatite pipe is rich in forsterite, and its axial zone has calcite-rich rocks, where magnetite and apatite are common minerals (Mikhailova et al., Reference Mikhailova, Kalashnikov, Sokharev, Pakhomovsky, Konopleva, Yakovenchuk, Bazai, Goryainov and Ivanyuk2016). Transition between these zones is gradual. Numerous carbonatite veins cut the phoscorite body, with the highest concentration of veins encountered in its axial, calcite-rich zone. Both phoscorite and carbonatite formed at ~377–383 Ma (U–Pb and Th–Pb methods using zircon, baddeleyite, apatite and calcite) (Bayanova et al., Reference Bayanova, Kirnarsky and Levkovich1997; Amelin and Zaitsev, Reference Amelin and Zaitsev2002; Rodionov et al., Reference Rodionov, Belyatsky, Antonov, Kapitonov and Sergeev2012). The latest episode in the pipe formation is distinguished by funnel-like bodies of breccia consisting of fragments of phoscorite, carbonatite and fenite cemented by colloform carbonate-rich fluorapatite (‘staffelite’).
The concentric petrographic zoning of the phoscorite-carbonatite pipe is accompanied by zonal distribution of properties (morphology, grain size, characteristic inclusions) and composition of rock-forming minerals (Ivanyuk et al., Reference Ivanyuk, Kalashnikov, Pakhomovsky, Mikhailova, Yakovenchuk, Konopleva, Sokharev, Bazai and Goryainov2016; Mikhailova et al., Reference Mikhailova, Kalashnikov, Sokharev, Pakhomovsky, Konopleva, Yakovenchuk, Bazai, Goryainov and Ivanyuk2016). In particular, magnetite grains with rare exception contain inclusions of earlier ilmenite-group minerals or later spinel formed by (oxy)exsolution. Earlier (apatite)-forsterite phoscorites of the pipe marginal zone includes Ti-rich magnetite with geikielite–ilmenite lamellae, intermediate magnetite-rich phoscorites carry Mg–Al-rich magnetite with inclusions of spinel (always) and quintinite (sometimes), and late carbonatites again contain Ti-rich magnetite with inclusions of geikielite–ilmenite. In transition zones, there are magnetite grains with spinel inclusions in the core and riddled with ilmenite–geikielite inclusions in the rim.
Quintinite is widespread in the calcite-rich phoscorites and carbonatites, where it forms characteristic inclusions in the Mg–Al-rich magnetite. These inclusions result from the specific kind of magnetite ‘carbo-exsolution’ and alteration of spinel formed by the exsolution from Mg–Al-rich magnetite, and are restricted to the marginal zones of magnetite crystals, the cores of which contain exsolution inclusions of unaltered spinel. Thus, there are two typical kinds of quintinite inclusions: rounded homogeneous grains (Fig. 2a) and octahedral pseudomorphs in which a few spinel relics are preserved (Fig. 2b). There are two possible mechanisms of quintinite formation due to the ‘carbo-exsolution’ of Mg–Al-rich magnetite and alteration of exsolution spinel that can be schematically described by the following reactions (taking into account the composition of magnetite Mag55–66Mfr29–40 and spinel Spl87–91Hc3–5 in quintinite-bearing phoscorite, as well as content of spinel inclusions in magnetite of 6–30 vol.%):



Fig. 2. Back-scattered electron images of rounded inclusions of quintinite in the marginal zone of a magnetite grain (a); and relics of spinel in euhedral inclusions of quintinite in magnetite (b). Quin – quintinite, Mag – magnetite, Spl – spinel, Bdy – baddeleyite, Fo – forsterite and Phl – phlogopite.
Residual Al2O3 is localized in late quintinite, spinel, böhmite and other Al-(hydr)oxides crystals, which are common in cavities within these rocks (Ivanyuk et al., Reference Ivanyuk, Yakovenchuk and Pakhomovsky2002).
Late hydrothermal quininite can be divided in two major morphologically different groups: (1) Carbonatized magnetite-rich phoscorites of the intermediate zone contain numerous cavities (up to 20 cm diameter) with walls incrusted by well-shaped crystals of quintinite (Fig. 3). This quintinite occurs as isometric or flattened hexagonal pyramidal crystals, hexagonal plates and spherulites. The mineral ranges in colour from colourless and white to different shades of brown, yellow, red, blue, grey and green. The association of quintinite varies for each individual void, but such minerals as dolomite, calcite, magnetite, phlogopite, clinochlore and magnesite are typical for most of the quintinite-bearing samples (studied by Ivanyuk et al., Reference Ivanyuk, Yakovenchuk and Pakhomovsky2002; Krivovichev et al., Reference Krivovichev, Yakovenchuk, Zhitova, Zolotarev, Pakhomovsky and Ivanyuk2010a, Zhitova et al., Reference Zhitova, Yakovenchuk, Krivovichev, Zolotarev, Pakhomovsky and Ivanyuk2010 and reported therein). (2) Quintinite has also been found in veinlets in the calcite-magnetite phoscorites, which are often formed by pure quintinite, sometimes in association with calcite. This quintinite is morphologically different and occurs as colourless, white, pinkish or greyish flakes that in places form spherulites (studied by Krivovichev et al., Reference Krivovichev, Yakovenchuk, Zhitova, Zolotarev, Pakhomovsky and Ivanyuk2010b).

Fig. 3. Morphologically different quintinite generations grown on one another: Q6-I → Q6-II → Q6-III (a) and Q20-I → Q20-II (b).
In addition to quintinite, other hydrotalcite-supergroup minerals at Kovdor reported so far are karchevskyite, [Mg18Al9(OH)54][Sr2(CO3,PO4)9(H2O, H3O)11] (Britvin et al., Reference Britvin, Chukanov, Bekenova, Yagovkina, Antonov, Bogdanova and Krasnova2007) and pyroaurite-3R, [Mg6Fe3+2(OH)16][(CO3)(H2O)4] (Zhitova et al., Reference Zhitova, Popov, Krivovichev, Zaitsev and Vlasenko2016c) (originally described as ‘sjögrenite’ by Ivanyuk et al., Reference Ivanyuk, Yakovenchuk and Pakhomovsky2002).
Materials and methods
Three samples of quintinite have been investigated in the present study, denoted by Q6, Q20 and Q21. The last is remarkable for its green colour due to the high content of Fe2+ that will be discussed below. For samples Q6 and Q20, different quintinite generations, grown on one another, have been observed. The Q6 sample comprised three morphologically different modifications of quintinite (Fig. 3a), denoted in the order of their appearance as Q6-I (the earliest), Q6-II (intermediate) and Q6-III (final), while the Q20 sample has only two generations denoted as Q20-I and Q20-II (Fig. 3b).
The chemical composition of quintinite was determined by wavelength dispersion spectrometry using a Cameca MS-46 electron microprobe (Geological Institute of the Kola Science Center of the Russian Academy of Sciences) operating at 20 kV and 20–30 nA. The standards used were pyrope for Mg and Al and hematite for Fe. The obtained averaged values of 6–10 analyses for each specimen are given in Table 1.
Table 1. Chemical composition of the quintinite sample Q21, Q6 and Q20 (wt.%).

aEqual to the calculation on the basis of oxygens (18 + the number of H2O molecules – 3).
bCalculated according to the charge-balance requirement.
cCalculated from the crystal structure.
Single-crystal X-ray diffraction data for the samples Q6-I, Q6-II and Q20-II were collected by means of a Stoe IPDS II Image-Plate-based X-ray diffractometer (MoKα) operated at 50 kV and 40 mA (X-ray diffraction Resource Center, St. Petersburg State University). For the samples Q6-III, Q20-I and Q21, the measurements were carried out by means of a Bruker Smart Apex diffractometer (X-ray diffraction Resource Center, St. Petersburg State University), MoKα radiation, operated at 50 kV and 40 mA, equipped with a CCD area detector (Table 2). The unit-cell parameters were refined by least-squares methods. The SHELX program package was used for all structural calculations (Sheldrick, Reference Sheldrick2008).
Table 2. Crystallographic data, parameters of data collection and refinement for quintinite polytypes.

*$\; w = \; 1/\left[ {{\rm \sigma} ^2F_0^2 + {\left( {aP} \right)}^2 + bP} \right],{\rm \; where\; {\it{P}}} = \left( {\max (F_0^2, \; 0} \right) + 2F_c^2 )/3$
Positions of cations of the metal-hydroxide layer were determined by direct methods. The interlayer species (O and C) and hydrogen atoms were located from the inspection of difference-Fourier maps and refined isotropically. Site occupancies of Mg, Al and O of metal-hydroxide layers were found to be close to 100% except for the high-Fe sample Q21, where the occupancy of the M1 site was refined as Mg0.874(2)Fe0.126(2) (Tables 3, 4). Refined site occupancies of interlayer atoms (Tables 3, 4) suggest that, in some cases, carbon sites are also populated by oxygen atoms of H2O molecules (i.e. some sites assigned to CO2 groups are replaced by H2O molecules). In cases when an excess of electrons per formula unit (epfu) in the interlayer was observed (in comparison to the experimentally obtained chemical formula), it was treated as an excess of H2O molecules. The H2O contents given in Table 1 were calculated on the basis of crystal-structure refinement. Hydrogen atoms of the brucite-type layers were refined with no restraints. The hydrogen atoms of the interlayer cannot be located because of the high statistic disorder of the H2O molecules and carbonate groups.
Table 3. Atom coordinates, equivalent isotropic displacement parameters (Å2) and site occupancies for quintinite-2T.

Table 4. Atom coordinates, equivalent isotropic displacement parameters (Å2) and site occupancies for disordered quintinite-3R.

The infrared (IR) spectra of quintinite were recorded using a Bruker Vertex IR spectrometer (X-ray diffraction Resource Center, St. Petersburg State University). The measurements were taken at room temperature using the KBr technique.
In order to investigate structural complexity of quintinite polytypes, an estimate of an amount of structural Shannon information per atom (I G) and per unit cell (I G,total) was made according to the approach developed by Krivovichev (Reference Krivovichev2012, Reference Krivovichev2013, Reference Krivovichev2014, Reference Krivovichev, Danisi and Armbruster2015, Reference Krivovichev2016). It has recently been shown by Krivovichev (Reference Krivovichev2016) that the I G value provides a negative contribution to the configurational entropy (S cfg) of crystalline solids, in accordance with the general principle that the increase in structural complexity corresponds to the decrease of the S cfg value.
The structures of all quintinite polytypes contain a high proportion of largely vacant interlayer sites, which are difficult to take into account (it should also be noted that some sites may or may not be split, which introduces additional artifacts related to the specifics of handling the structure refinements). In order to avoid these problems, only fully occupied sites were taken into account, that is, only the sites that belong to the [Mg4Al2(OH)12]2+ layers. This condition may significantly affect the structural complexity parameters, especially for low-symmetry polytypes, and therefore the calculated parameters (Table 5) should be considered as a first approximation only.
Table 5. Crystallographic data and structural complexity parameters for five experimentally observed polytypes of quintinite.

* Only atoms from the metal-hydroxide layers were taken into account (see Materials and methods).
** (1) Krivovichev et al. (Reference Krivovichev, Yakovenchuk, Zhitova, Zolotarev, Pakhomovsky and Ivanyuk2010a); (2) this work; (3) Zhitova et al. (Reference Zhitova, Yakovenchuk, Krivovichev, Zolotarev, Pakhomovsky and Ivanyuk2010); (4) Krivovichev et al. (Reference Krivovichev, Yakovenchuk, Zhitova, Zolotarev, Pakhomovsky and Ivanyuk2010b).
Results
Chemical composition
In all the samples studied, the dominant elements are Mg and Al, with the only significant impurity in the Kovdor quintinites being Fe (Table 1). Other elements (e.g. Mn and Zn) were also considered but their content was lower than the detection limit of the electron microprobe. The chemical formula was calculated on the basis of Mg + Al + Fe = 6. In the quintinite crystals reported here, the Fe amount gradually decreases from the central part to the rim. The Fe content displays a negative correlation relative to the Mg content, which is most evident in the high-Fe sample Q21 (Table 1), whereas the Al content is relatively constant. Therefore, Fe is considered as Fe2+, suggesting that there is a solid solution between quintinite and its Fe2+-analogue, caresite. The observed values of the M 2+:M 3+ ratio for the quintinite samples under investigation range from 2:1 to 2.3:1. These ratios are consistent with the ideal chemical formula of quintinite, [Mg4Al2(OH)12]CO3(H2O)3 rather than hydrotalcite. The increase of M 2+:M 3+ from 2:1 to 2.3:1 implies the decrease of the CO2 (charge-balancing interlayer species) content. The CO2 content in Table 1 was calculated on the basis of the charge balance of the metal–hydroxide layer. Then the calculated number of epfu for CO2 was excluded from the total epfu refined for the interlayer, the remaining epfu were recalculated to interlayer H2O molecules. The total sums provided in Table 1 range from 96 to 103.9 wt.%, which is not surprising taking into account that the volatile constituents of quintinite sum to ~45 wt.%.
The IR spectra of quintinite with M 2+:M 3+ = 2:1 (Fig. 4) contain the following main absorption bands (cm–1): ~3450 (O–H stretching), 3100–2700sh (interaction of interlayer water and anions), 1750–1500sh (H2O molecules), ~1400sh and 1355 (carbonate), 960–940sh (Al–OH), 880–860sh (overlap of the O–H bending and carbonate ions), ~785 (Al–OH), 680–670sh (M–OH vibration or/and carbonate), 552, 449–446 and 393–390 (М–О, М–О–М and О–М–О) (Hernandez-Moreno et al., Reference Hernandez-Moreno, Ulibarri, Rendon and Serna1985; Cavani et al., Reference Cavani, Trifiro and Vaccari1991; Moroz and Arkhipenko, Reference Moroz and Arkhipenko1991; Chao and Gault, Reference Chao and Gault1997; Prikhod'ko et al., Reference Prikhod'ko, Sychev, Astrelin, Erdmann, Mangel' and van Santen2001; Kloprogge, Reference Kloprogge and Kloprogge2005; Frost et al., Reference Frost, Spratt and Palmer2009; Theiss et al., Reference Theiss, López, Frost and Scholz2015), where sh – shoulder.

Fig. 4. Characteristic IR spectrum of Kovdor quintinite with M 2+:М 3+ = 2:1.
Crystal structure
The single-crystal X-ray diffraction study showed that different generations of quintinite in each of samples Q6 (-I, -II, -III) and Q20 (-I, -II) correspond to different polytypic modifications. The crystal structures of Q6-I and Q20-I were refined in the hexagonal space group P63/mmc, with the atomic arrangement characterized by the random distribution of Mg and Al cations in octahedral sites and a hexagonal stacking sequence, which corresponds to a 2H polytype as described by Zhitova et al. (Reference Zhitova, Yakovenchuk, Krivovichev, Zolotarev, Pakhomovsky and Ivanyuk2010). The structure of Q6-II corresponds to a 2T polytype, which is new and is described in more details below. The crystal structures of the Q6-III and Q20-II samples were refined in the monoclinic space group C2/m. In their structures, Mg2+ and Al3+ cations have ordered arrangements forming a $\sqrt 3 $ ×
$\sqrt 3 $ superstructure within the brucite-type layers. The stacking of the layers agrees with the rhombohedral pattern, but the cation ordering results in the decreasing of symmetry from rhombohedral to monoclinic (1M polytype) (Krivovichev et al., Reference Krivovichev, Yakovenchuk, Zhitova, Zolotarev, Pakhomovsky and Ivanyuk2010b, Reference Krivovichev, Antonov, Zhitova, Zolotarev, Krivovichev and Yakovenchuk2012a,Reference Krivovichev, Yakovenchuk, Zhitova and Krivovichevb, Zhitova et al., Reference Zhitova, Ivanyuk, Krivovichev, Yakovenchuk, Pakhomovsky and Mikhailova2016a).
2T polytype
The inspection of hk0 sections of reciprocal space for Q6-II (Fig. 5) showed the presence of clear and regular superstructure reflections. Initial indexing of diffraction patterns provided the unit-cell with parameters, a ≈ 5.28 and c ≈ 15.12 Å implying the presence of a $\sqrt 3 $ ×
$\sqrt 3 $ (honeycomb) ordering of the M 2+–M 3+ cations within octahedral layers. The crystal structure of Q6-II consists of the M 2+–M 3+-ordered brucite-type [Mg4Al2(OH)12]2+ layers in which each Al3+ site is surrounded by six Mg2+, avoiding sharing of edges between two M 3+ octahedra and forming a
$\sqrt 3 $ ×
$\sqrt 3 $ superstructure (Fig. 6). There are two M positions in the metal–hydroxide layer that are different in the average <M–O> bond lengths: 2.066 and 1.926 Å (Table 6). The longer bond refers to the < Mg–O > distance, whereas the shorter one corresponds to the <Al–O> distance. The [Mg4Al2(OH)12]2+ layers are stacked along the c axis according to a hexagonal 2H pattern (Fig. 6). The ordering of the Al3+ cations results in the appearance of a superstructure and the decrease of symmetry from hexagonal to trigonal. The residual electron density map at the interlayer level is shown in Fig. 6 and is typical for quintinite (Krivovichev et al., Reference Krivovichev, Yakovenchuk, Zhitova, Zolotarev, Pakhomovsky and Ivanyuk2010a,b; Zhitova et al., Reference Zhitova, Yakovenchuk, Krivovichev, Zolotarev, Pakhomovsky and Ivanyuk2010). It contains toroidal rings with the electron density corresponding to the interlayer O atoms with the separate maxima corresponding to the C4+ cations and H2O molecules. Some of the C–O distances given in Table 6 do not match the expected C–O bond lengths because of the toroidal distribution of electron density and the resulting imprecise determination of positions of interlayer O atoms. As the symmetry of the structure is trigonal and the unit cell contains two layers, the polytype should be identified as 2T in accordance with the polytype nomenclature (Guinier et al., Reference Guinier, Bokij, Boll-Dornberger, Cowley, Durovic, Jagodzinski, Krishna, DeWolff, Zvyagin, Cox, Goodman, Hahn, Kuchitsu and Abrahams1984).

Fig. 5. Slices of reciprocal space of 3R (a, b) and 2T (c, d) polytypes. Cation ordering results in the appearance of weak superstructure reflections at hk1 (c) that are presented as regular reflections or diffuse lines at 0kl (d).

Fig. 6. Crystal structure of 3R and 2T polytypes within the metal-hydroxide layer (a, d) and along stacking (b, e), respectively. The white dashed line indicates the cutting direction with respect to the layer stacking. Maps of the residual electron density at the interlayer level are shown in (c) and (f) for 3R and 2T polytypes, respectively.
Table 6. Selected bond lengths (Å) and angles (°) in the crystal structure of quintinite-2T and -3R.

3R polytype
The inspection of the hk0 and h01 sections of reciprocal space for the sample Q21 revealed only strong reflections corresponding to the –h + k + l = 3n condition (Fig. 5). The absence of superstructure reflections indicates the absence of ordering of Mg, Al and Fe in the overall crystal structure. Initial indexing of the diffraction pattern provided the unit cell with the parameters of a ≈ 3.06 and c ≈ 22.67 Å. The coordinates of atoms in the metal–hydroxide layer were determined in the space group R3 and subsequently transformed into the space group R $\bar 3$m using the PLATON program (Speck, Reference Speck2003).
The crystal structure of Q21 is based upon double hydroxide layers [M 2+,3+(OH)2] with disordered distribution of Mg, Al and Fe in the single M site (Fig. 6). Metal–hydroxide layers are stacked according to the rhombohedral pattern (Fig. 6). The <M–O> bond length is equal to 2.028 Å. The difference-Fourier electron-density map at the interlayer level is shown in Fig. 6. As the unit-cell consists of three layers and has a rhombohedral symmetry, the sample should be identified as quintinite-3R (Guinier et al., Reference Guinier, Bokij, Boll-Dornberger, Cowley, Durovic, Jagodzinski, Krishna, DeWolff, Zvyagin, Cox, Goodman, Hahn, Kuchitsu and Abrahams1984).
Discussion
Genetic features of quintinite
Hydrotalcite-supergroup members are normally late-stage hydrothermal minerals. Many of them have been described as alteration products of primary minerals. For instance, woodallite, [Mg6Cr2(OH)16][Cl2(H2O)4], forms as a result of hydrothermal alteration (T < 320°C) of primary magmatic chromite by Cl-rich solutions (Grguric et al., Reference Grguric, Madsen and Pring2001) and stichtite, [Mg6Cr2(OH)16][(CO3)(H2O)4], was reported recently to be formed during serpentinization of rocks in a methane- and/or hydrogen-rich atmosphere within fluid channels at T ≤ 300°C and P of 0.8–1.2 GPa (Melchiorre et al., Reference Melchiorre, Bottrill, Huss and Lopez2017). Hydrowoodwardite, [Cu1–xAlx(OH)2][(SO4)x/2(H2O)n], is an alteration product of primary phosphates (Witzke, Reference Witzke1999), whereas hydrohonessite, [Ni1–xFe3+x(OH)2][(SO4)x/2(H2O)n], is a weathering product of Ni–Fe sulfides (Nickel and Wildman, Reference Nickel and Wildman1981; Bindi et al., Reference Bindi, Christy, Mills, Ciriotti and Bittarello2015). Droninoite, [Ni6Fe3+2(OH)16][Cl2(H2O)4], is a product of terrestrial alteration of iron meteorite (Chukanov et al., Reference Chukanov, Pekov, Levitskaya and Zadov2008, Reference Chukanov, Pekov, Levitskaya and Zadov2009), while mountkeithite, [Mg9Fe3+3(OH)24][Mg2(SO4)3.5(H2O)9], replaces pyroaurite under influence of low-temperature solutions (Hudson and Bussell, Reference Hudson and Bussell1981).
In the Kovdor complex, the earlier quintinite is formed after Mg–Al rich magnetite and spinel at T < 375°C (temperature of quintinite decomposition [Panikorovsky et al., Reference Panikorovskii, Zhitova, Krivovichev, Zolotarev, Britivn, Yakovenchuk and Krzhizhanovskaya2015]). The average equilibration temperature of ilmenite–geikielite oxy-exsolution in 35 quintinite-bearing Mg–Al–Ti-rich magnetites estimated using the geothermometer of Ghiorso and Evans (Reference Ghiorso and Evans2008) is 329 ± 79°C. Later exsolution of residual Mg–Al rich magnetite (with exsolution lamellae of ilmenite–geikielite) occurred at a lower temperature (up to 200°C) therefore even the earlier ‘carbo-exsolution’ quintinite is in fact a low-temperature mineral. It should be pointed out that LDHs can be synthesized hydrothermally from mixed oxides (for Mg–Al LDHs, from MgO and Al2O3) at ~110°C for 5 or 10 days (Xu and Lu, Reference Xu and Lu2005). This method of the LDH synthesis results in better crystallinity of the obtained material compared to room-temperature coprecipitation. Moreover, there were a number of experiments of re-crystallization of LDHs from mixed-metal oxides obtained by calcination of the initial LDH phases (Rocha et al., Reference Rocha, del Arco, Rives and Ulibarri1999; Stanimirova et al., Reference Stanimirova, Stoilkova and Kirov2007; Stanimirova and Balek, Reference Stanimirova and Balek2008; Klemkaite et al., Reference Klemkaite, Prosycevas, Taraskevicius, Khinsky and Kareiva2011). It was found that LDHs could be recovered from hydrothermally treated mixed-metal oxides. The M 2+:M 3+ ratio depends upon the ratio in the initial LDH and the composition of solutions, while cation affinity is determined by pH. The Mg–Al LDHs are formed at pH > 10 (Stanimirova et al., Reference Stanimirova, Stoilkova and Kirov2007).
Late hydrothermal quintinite generations in the Kovdor complex can be divided roughly into two groups: (1) well-developed hexagonal crystals found in voids and (2) monomineralic filling of cracks within phoscorite. All five polytypic modifications: 2T-3c [6R], 2T, 2H, 3R, 1M are found in euhedral hexagonal crystals in voids with quintinite-2H being the most common polytype (Krivovichev et al., Reference Krivovichev, Yakovenchuk, Zhitova, Zolotarev, Pakhomovsky and Ivanyuk2010a, Reference Krivovichev, Yakovenchuk, Zhitova and Krivovichev2012b, Zhitova, Reference Zhitova2013), whereas material forming veinlets was identified as quintinite-1M (Krivovichev et al., Reference Krivovichev, Yakovenchuk, Zhitova, Zolotarev, Pakhomovsky and Ivanyuk2010b).
Kovdor quintinites remain close to the M 2+:M 3+ ratio of 2:1, but some deviations up to 2.3:1 have also been observed (Table 1). As there is an inverse correlation between Mg and Fe in high-Fe samples, it can be concluded that quintinite may form a solid solution series with caresite [Fe2+4Al2(OH)12][(CO3)(H2O)3] as observed in Mont Saint-Hilaire, Quebec, Canada, by Chao and Gault (Reference Chao and Gault1997). The amount of Fe3+ in quintinite appears to be restricted, in contrast to hydrotalcite, which accommodates Fe as Fe3+ rather than Fe2+ replacing Al (Mills et al., Reference Mills, Christy and Schmitt2016). When Fe is in the ferric state at Kovdor, it leads to the formation of pyroaurite, [Mg6Fe3+2(OH)16][(CO3)(H2O)4], instead of quintinite. It is noteworthy that an Fe3+-analogue of quintinite and an Fe2+-analogue of hydrotalcite have not yet been found in nature, although synthesis of LDHs with Mg:Fe3+ = 2:1 has been reported (Vucelic et al., Reference Vucelic, Jones and Moggridge1997; Hadnadev-Kostić et al., Reference Hadnadev-Kostić, Vulić and Marinković-Nedućin2010).
Crystal structure of quintinite-2T
The crystal structure of quintinite-2T reported herein is similar to that of quintinite-2H determined by Arakcheeva et al. (Reference Arakcheeva, Pushcharovskii, Atencio and Lubman1996) for quintinite from the Jacupiranga mine, São Paulo, Brazil. The crystal structure of Brazilian quintinite was reported in the P $\bar 6$2m space group with the unit-cell parameters a = 5.283(3) and c = 15.150(9) Å. The main difference between our and their reported structure is in the architecture of the interlayer space. In the model proposed by Arakcheeva et al. (Reference Arakcheeva, Pushcharovskii, Atencio and Lubman1996) there are three types of alternating sheets: (1) [Mg4Al2(OH)12]2+ octahedral layers characterized by the
$\sqrt 3 $ ×
$\sqrt 3 $ superstructure due to the M 2+–M 3+ ordering; (2) the layer of carbonate groups; and (3) the layer of H2O molecules. The three types of layers are stacked in the -12131213- sequence. While in the crystal structure of quintinite-2T reported herein, all interlayer spaces are symmetrically equivalent to each other and consist of disordered arrangements of CO3 and H2O groups (Fig. 5). The alternating arrangement of carbonate and H2O layers in Brazilian quintinite is rather questionable, because, in that model, adjacent cationic octahedral layers are supposed to be connected alternatively via negatively charged carbonate groups (layer 2) and neutral H2O molecules (layer 3). Such a structure would result in the expansion of the interlayer 1-3-1 accompanied by the contraction of the 1-2-1 interlayer due to the weaker bonding.
Quintinite-2T is characterized by the combination of the $\sqrt 3 $ ×
$\sqrt 3 $ superstructure of octahedral layers and the hexagonal stacking sequence (Fig. 6). Quintinite-2H (Zhitova et al., Reference Zhitova, Yakovenchuk, Krivovichev, Zolotarev, Pakhomovsky and Ivanyuk2010) is different from quintinite-2T by the random cation arrangement in octahedral layers (Fig. 6). The crystal structure of quintinite-2T-3c [6R] (originally reported as 2H-3c [6R] by Krivovichev et al., Reference Krivovichev, Yakovenchuk, Zhitova, Zolotarev, Pakhomovsky and Ivanyuk2010a) is built up of the Mg–Al-ordered metal–hydroxide layers (Fig. 6). Crystallographic parameters of these polytypes are compared in Table 2.
In terms of the approach developed by Bookin and Drits (Reference Bookin and Drits1993), the 2H 1 and 2T polytypes both correspond to the sequence …=AC = CA=… if no account is taken of the distribution of M 2+ and M 3+ cations. If cation ordering is taken into account (Krivovichev et al., Reference Krivovichev, Yakovenchuk, Zhitova, Zolotarev, Pakhomovsky and Ivanyuk2010a,b), then three distinct positions for cation layers can be distinguished on the basis of the Al localization: e.g. b1, b2 or b3 positions. Thus the 2T polytype reported here should be described as …=Ab1C = Cb1A=…, where b1 corresponds to the positions of Al. The full description of the layer sequence corresponds to a two-layer polytype and is characterized by the presence of cation ordering within brucite-type layers. However, the …=Ab1C = Cb1A=… 2T polytype is different from the …AbC-BcA = AbC… 2T polytype listed by Mills et al. (Reference Mills, Christy, Génin, Kameda and Colombo2012a), which has two different types of the interlayer space (P and O).
Crystal structure of quintinite-3R
The crystal structure of quintinite-3R is isotypic to that published by Allmann and Jepsen (Reference Allmann and Jepsen1969) for quintinite (originally reported as ‘hydrotalcite’ with M 2+:M 3+ = 2:1) from Věžná, Moravia, Czech Republic. The structure of the Moravian mineral was reported in the same space group (R $\bar 3$m), which is the highest symmetry for LDHs with a rhombohedral type of layer stacking (Mills et al., Reference Mills, Christy, Génin, Kameda and Colombo2012a). The unit-cell parameters of Moravian quintinite are a = 3.054 and c = 22.81 Å.
Despite the general viewpoint that the 3R polytype is the most common for hydrotalcite-supergroup minerals, crystal-structure studies of members that combine the M 2+:M 3+ ratio of 2:1 and a rhombohedral stacking sequence indicate a high tendency for the M 2+ and M 3+ cations to form $\sqrt 3 $ ×
$\sqrt 3 $ superstructures within brucite-type layers that results in a monoclinic symmetry (Table 2) due to the disappearance of threefold axes (synthetic LDHs with Li:Al = 1:2 [Sissoko et al., Reference Sissoko, Iyagba, Sahai and Biloen1985; Britto et al., Reference Britto, Thomas, Kamath and Kannan2008; Britto and Kamath, Reference Britto and Kamath2009], quintinite-1M [Krivovichev et al., Reference Krivovichev, Yakovenchuk, Zhitova, Zolotarev, Pakhomovsky and Ivanyuk2010b, Reference Krivovichev, Antonov, Zhitova, Zolotarev, Krivovichev and Yakovenchuk2012a, Zhitova et al., Reference Zhitova, Popov, Krivovichev, Zaitsev and Vlasenko2016c; Jayanthi et al., Reference Jayanthi, Nagendran and Kamath2015]; synthetic LDHs with Zn:Al = 2:1 [Marappa and Kamath, Reference Marappa and Kamath2015]). It is important to emphasize that a ‘classic’ 3R polytype was found for a quintinite sample with a very high content of Fe2+. The observed absence of superstructure reflections that would be indicative of the M 2+–M 3+ ordering is due to the presence of a mixed M 2+ site statistically occupied by Mg (≥85 %) and Fe2+ (≤15 %). The statistical disorder of Fe and Mg prevents registration of the M 2+–M 3+ ordering by single-crystal X-ray diffraction. According to Bookin and Drits (Reference Bookin and Drits1993), the crystal structure of our sample belongs to the ‘classic’ 3R 1 polytype and the stacking sequence of layers can be expressed as …=АB = BC = CA=… Due to the fact that all M sites are symmetrically equivalent, characterization of cation positions (b) is omitted.
The evolution of polytype formation 2H → 2T → 1M
According to the observations of polytype overgrowth, the sequence of polytype formation for quintinite is 2H → 2T → 1M that can be described as a sequential change from the Mg–Al-disordered polytype with a hexagonal stacking (2H) through the Mg–Al-ordered polytype with a hexagonal stacking (2T) to the Mg–Al-ordered polytype with rhombohedral stacking (1M). This sequence was attributed to the decrease of temperature during crystallization process. The idea that type of layer stacking (2H and 3R) can be affected by the temperature of formation was expressed by Allmann (Reference Allmann1968) for pyroaurite from the Långban deposit, Sweden, where the rhombohedral low-temperature polytype was found in the outermost rims of crystals, while the 2H polytype was found in the cores. Synthetic LDHs with Mg:Al = 2:1 obtained at nearly ambient conditions are usually reported as having a rhombohedral (3R) stacking sequence (Drits and Bookin, Reference Drits, Bookin and Rives2001). It is worth mentioning that nearly all synthetic Mg–Al LDHs have Mg:Al = 2:1, however, they are reported in the literature as ‘hydrotalcites’ regardless of their chemical composition, which implies that they are synthetic analogues of quintinite, also noted by Mills et al. (Reference Mills, Christy, Génin, Kameda and Colombo2012a).
The evolution of polytypic forms of quintinite during crystallization can be examined from the viewpoint of information-based structural complexity measures calculated by means of the equations. According to this approach, the 2H → 2T → 1M sequence corresponds to the following evolution of structural complexity parameters (I G/I G,total, in bits): 1.522/15.219 → 1.706/51.168 → 2.440/36.603. It can be seen that the structural information amount per atom (I G) is gradually increasing along the sequence. Krivovichev (Reference Krivovichev2016) demonstrated that the I G value that contributes negatively to the configurational entropy of crystalline solids and therefore the evolution of polytypic modifications during crystallization corresponds to the decreasing configurational entropy. This is in agreement with the general principle that decreasing temperature corresponds to the appearance of more complex structures. The occurrence of the low-complexity 3R polytype in the present study is likely to be causally linked to its very high content of Fe2+. It is possible that significant concentrations of this cation disrupt weak interactions between Mg and Al, which determine long-range ordering in the other polytypes. On the other hand, the 2T-3c [6R] polytype has the highest complexity among all known quintinite polytypes, and its appearance cannot be explained easily. However, the general trend of the crystallization of more complex low-entropy structures with decreasing temperature agrees well with the observed sequence of phases in quintinite-bearing samples.
Acknowledgements
This research was supported by the Russian Science Foundation (project no. 16-17-10173). X-ray diffraction measurements and chemical studies (partially) have been carried out at the X-ray diffraction and Geomodel Resource Centres of St. Petersburg State University. The authors are grateful to Natalia V. Zubkova, Lyudmila M. Lyalina and Oxana V. Karimova for fruitful discussion of the results. We thank Stuart Mills, Pete Leverett, an anonymous reviewer and the Principal Editor Peter Williams for improving the manuscript quality.