Introduction
Lichens are a symbiosis between an ascomycete and one or more photosynthesizing algae or cyanobacteria, often with small amounts of basidiomycete yeasts located in the lichen cortex (Spribille et al. Reference Spribille, Tuovinen, Resl, Vanderpool, Wolinski, Aime, Schneider, Stabentheiner, Toome-Heller and Thor2016). Lichens frequently grow in exposed microhabitats where they experience high light levels. In photosynthetic tissues, excessive solar radiation can cause a temporary or more permanent reduction of photosynthesis known as ‘photoinhibition’. Photoinhibition is caused, directly or indirectly, by stimulation of the production of potentially harmful reactive oxygen species (ROS) that occurs when photosystems cannot use the light energy they are absorbing (Gururani et al. Reference Gururani, Venkatesh and Tran2015; Pospíšil Reference Pospíšil2016). It is now realized that in addition to being harmful, these ROS can also act as important signalling molecules (Foyer Reference Foyer2018). For example, singlet oxygen triggers programmes related to over-excitation of photosystem II (PSII) while superoxide and hydrogen peroxide promote the expression of other suites of genes that may serve to alleviate electron pressure on the reducing side of photosystem I (PSI). The D1 protein, a key component of PSII, is believed to be most sensitive to damage during photoinhibition. Some damage appears to occur even in moderate light intensities, and photosynthesizing organisms must therefore continuously repair the damage to this protein, which requires a certain level of hydration in lichens. The ‘PSII repair cycle’, occurring in chloroplasts and in cyanobacteria, involves degrading and re-synthesizing the D1 protein, followed by activation of the photosynthetic reaction centre (Nath et al. Reference Nath, Jajoo, Poudyal, Timilsina, Park, Aro, Nam and Lee2013). Strong evidence exists that photoinhibition regularly occurs in lichens in field situations (e.g. Leisner et al. Reference Leisner, Green and Lange1997; Gauslaa & Solhaug Reference Gauslaa and Solhaug2000; Jairus et al. Reference Jairus, Lõhmus and Lõhmus2009; Míguez et al. Reference Míguez, Fernández-Marín, Becerril and García-Plazaola2017).
Higher plants can reduce ROS formation by converting excess light energy using a process termed ‘non-photochemical quenching’ (NPQ), which varies between and within species, and increases with increasing ambient light (Gururani et al. Reference Gururani, Venkatesh and Tran2015). While lichen photobionts also display NPQ (e.g. Adams et al. Reference Adams, Demmig-Adams and Lange1993), lichen mycobionts additionally often respond to UV-B and thus to high light (as a natural correlation exists between elevated UV-B and high photosynthetically active radiation (PAR)) by synthesizing cortical sun-screening compounds such as usnic acid, atranorin and parietin (Solhaug & Gauslaa, Reference Solhaug and Gauslaa2012). In some lichens, UV light induces the synthesis of dark brown melanic pigments, which in addition to UV also screens PAR (Mafole et al. Reference Mafole, Solhaug, Minibayeva and Beckett2019b). Melanin-producing lichens come from a wide range of lichen taxa and grow on a variety of substrata (Gauslaa & Solhaug Reference Gauslaa and Solhaug2001).
Good indirect evidence exists that cortical melanins protect lichen photobionts against photoinhibition. For example, McEvoy et al. (Reference McEvoy, Gauslaa and Solhaug2007) transplanted thalli of Lobaria pulmonaria to three sites with low, medium and high light intensities for 100 days. Lichens at the site with the highest light became significantly melanized. Thalli from each of these sites were then transplanted to a highly exposed area for 12 days. The melanized thalli displayed a much smaller reduction of F v/F m (maximal efficiency of PSII photochemistry) than the material that had been in the more shaded sites, strongly suggesting that melanins are involved in photoprotection. Recently, Mafole et al. (Reference Mafole, Solhaug, Minibayeva and Beckett2019a) collected melanized and pale thalli from the same species only a few metres apart. Under laboratory conditions, melanized thalli were significantly less photoinhibited than pale thalli following a controlled exposure to high light. However, an inherent problem with both approaches is that the melanized thalli had a history of exposure to higher light levels than pale thalli. As a result, the photobionts of melanized thalli may have developed mechanisms that increased tolerance to photoinhibition, such as increased NPQ. It is therefore difficult to separate the protective effects of a melanized cortex from photobiont adaptations to high light.
Here we developed a method of exposing the photobiont layer of melanized versus pale thalli to high light directly, thus eliminating the effect of an upper cortex. Briefly, in three lichen species the lower cortices and medullas were surgically removed, the algal layer exposed to high light from below and the subsequent recoveries from photoinhibition were tested. One assumption of this approach is that the algal layer is homogeneous in itself. Typically, the algal layer of lichens is rather thin. For example, in Lobaria pulmonaria it was barely 46 µm and did not significantly vary with level of exposure (Gauslaa & Coxson Reference Gauslaa and Coxson2011). Therefore, as the size of individual photobiont cells often exceeds 10 µm, any self-shading is likely to be small. Our main aim was to test whether the higher tolerance to photoinhibition in melanized lichens is mostly the result of solar radiation screening in the upper melanized cortex orchestrated by the fungal partner, or whether it derives in part from algal adaptations.
Materials and Methods
Lichen material
The cephalolichen Crocodia aurata (Ach.) Link. was collected from Leucosidea sericea trees growing along Nottingham Road, KwaZulu Natal, South Africa. The chlorolichen Cetraria islandica (L.) Ach. was collected from soil at Ski, S Norway and the cephalolichen Lobaria pulmonaria (L.) Hoffm. from the bark of oak trees at Langangen, S Norway. These species are hereafter referred to simply by their generic names Crocodia, Cetraria and Lobaria. For each site, collections of pale (shaded) and melanic (exposed) thalli from the same population (< 5 m apart) were made at the same time. The lichen material was dried at room temperature in the laboratory and stored refrigerated for a maximum of two weeks. One day before each experiment, discs 1 cm in diameter were cut, and thallus reflectance measured on dry thalli as described below. Each disc was then cut in half, kept dry, and under a dissecting microscope a small section (c. 2–4 mm−2) of the lower cortex and the adjacent medulla was scraped away from one of the halves using the tip of a Pasteur pipette until the lower part of the continuous green photobiont layer was fully exposed. Both halves of the discs (i.e. one half intact and the other scraped) were then allowed to hydrate overnight on moist filter paper in Petri dishes under dim lighting (5 μmol m−2 s−1) at 15 °C in a thermostatically controlled chamber. Chlorophyll fluorescence parameters were measured the following day, and immediately afterwards lichen discs were subjected to a high light treatment as described below.
Reflectance measurements to estimate melanin content
Reflectance spectra of the upper cortex were recorded on dry, intact discs before the start of the experiment as described by Solhaug et al. (Reference Solhaug, Gauslaa, Nybakken and Bilger2003). Briefly, an integrating sphere (model ISP-50-REFL, OceanOptics, Eerbeek, The Netherlands) was pressed against the thalli, which were then illuminated with a halogen lamp (model DH2000, OceanOptics) through a 600 µm thick optical fibre connected to the input port of the integrating sphere. Reflectance (400–1050 nm) was measured with a spectrometer (model SD2000, OceanOptics) connected to the output port of the sphere with a 400 µm fibre. Reflection was calculated relative to a reference spectrum derived from a white reference tile (WS-2, OceanOptics). By using the readings at 550, 700 and 750 nm, the browning reflectance index (BRI), calculated as (1/R550 − 1/R700)/R750 (Solovchenko et al. Reference Solovchenko, Chivkunova, Merzlyak and Reshetnikova2001), was used as a quantitative estimate of melanic compounds.
Chlorophyll fluorescence
Chlorophyll fluorescence was measured using the ‘maxi’ red version of the Imaging PAM fluorimeter (Walz, Effeltrich, Germany). At each sampling event, an image of the two halves of each disc was captured. These images were compared with photographs of the discs. In the intact discs, fluorescence parameters were integrated over a large area. For the scraped discs, parameters were integrated over the area from which the lower cortex had been removed, typically several mm2 in size. Figure 1 illustrates a typical picture derived from the Imaging PAM. Thalli were initially dark-adapted at room temperature for 10 min, and a saturating flash was applied to determine F v/F m.
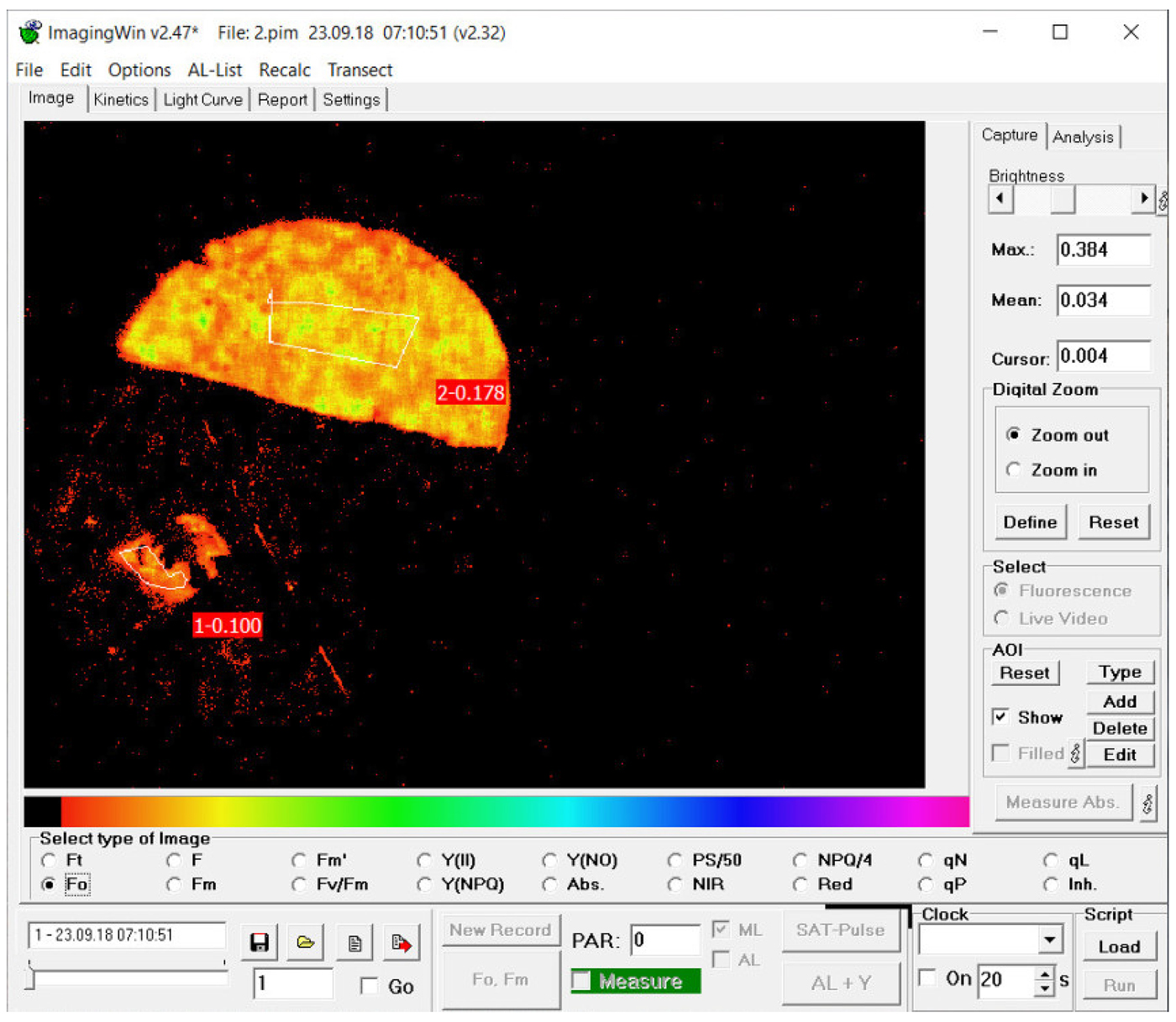
Fig. 1. Typical screenshot from the Walz Imaging PAM fluorimeter, here of a photoinhibited thallus of melanized Lobaria pulmonaria. The disc was 1 cm in diameter. For the half disc from which the lower cortex and medulla were removed (the lower half disc), parameters were measured from the lower side of the disc over the several mm2 from which tissue had been removed. From the intact disc (the upper half disc), parameters were measured from a large area on the normal upper surface. The parameter illustrated here is F o (minimal fluorescence yield of the dark adapted state).
The ‘ImagingWin’ software supplied by Walz with the Imaging PAM was used to estimate the maximal efficiency of PSII (F v/F m) calculated as:

where F m is the maximum fluorescence (photosynthetic reaction centres closed) and F o is the minimum fluorescence (photosynthetic reaction centres open).
High light stress
Hydrated lichen discs were exposed to high light stress using a white LED light-source panel (model SL-3500, Photon System Instruments, Brno, Czech Republic). For Lobaria, light was given at 600 μmol m−2 s−1 for 4 h; for Crocodia and Cetraria, light was given at 900 μmol m−2 s−1 for 4 h. In all cases, the two halves of each disc were exposed simultaneously; the intact discs were exposed from above, while the discs from which a part of the lower cortex and medulla had been removed were exposed from below. Chlorophyll fluorescence was measured for intact thalli from above and for scraped thalli from below, in moist discs before the high light treatment (‘time zero’), at the end of the high light treatment, and then after 0·5, 1, 2, 4, 6 and 20 h. As thalli were always dark-adapted for 10 min before measurement, the actual time of measurement was always 10 min later than indicated in Fig. 2. During recovery, discs were maintained at room temperature under dim lighting (5 μmol m−2 s−1), as recommended by Solhaug (Reference Solhaug2018). All experiments were repeated at least twice, with representative results being shown.
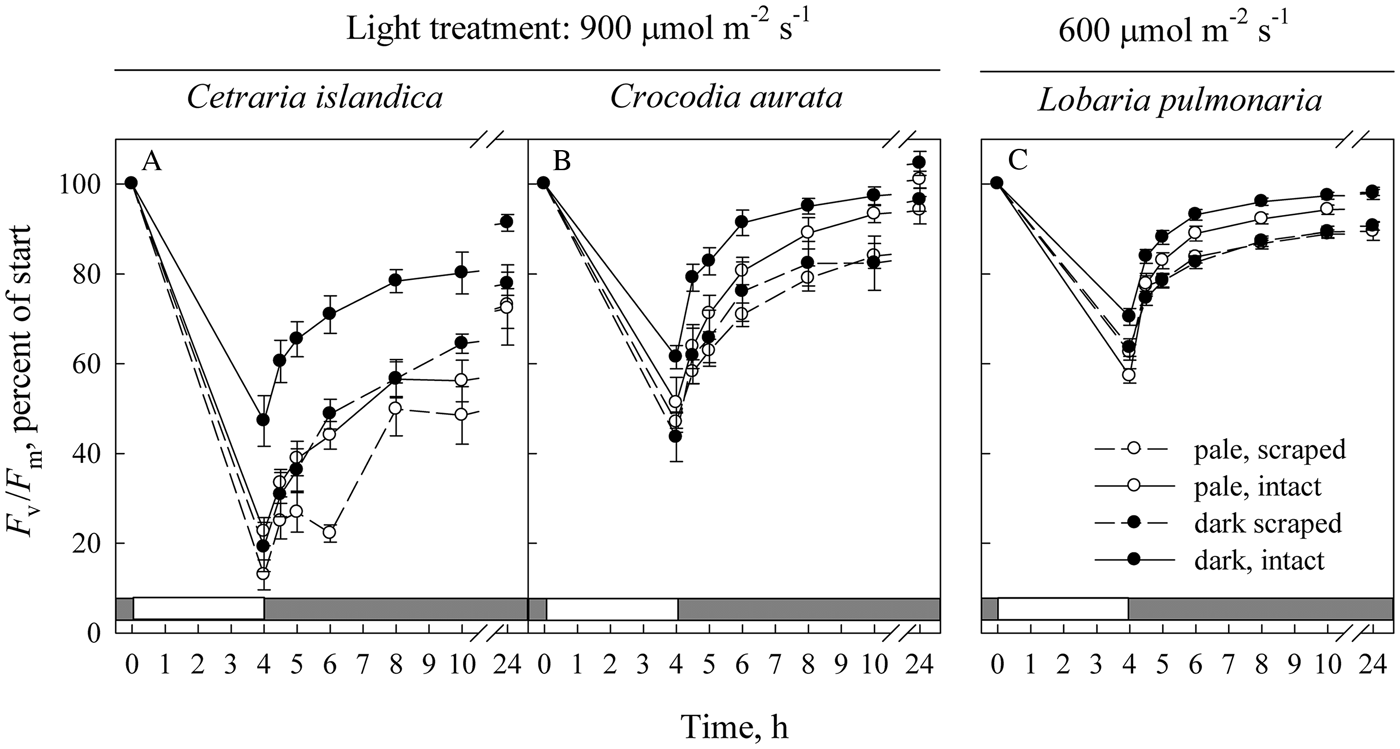
Fig. 2. The effect of melanization on the recovery of F v/F m (maximal efficiency of PSII photochemistry) following photoinhibitory exposure to light in Cetraria islandica (A), Crocodia aurata (B) and Lobaria pulmonaria (C). The initial data points (time = 0) are the values in freshly collected, hydrated material. As thalli were always dark-adapted for 10 min before measurement, the actual time of measurement of F v/F m was always 10 min later than indicated. Open symbols indicate pale thalli, while solid symbols represent melanized thalli. Solid lines represent intact material exposed to high light from above, while dashed lines represent material with part of the lower cortex and medulla removed and illuminated from below. Error bars of the mean show 1 SE (n = 12) when larger than symbol size. The white section in the grey row at the base of each graph indicates the time period when samples were exposed to light stress.
Statistics
Initial mean values of F v/F m were compared by ANOVA (Microsoft Excel) for each species. For the effects of light stress, species responded in species-specific ways. Therefore, we analyzed each species separately. Minitab 18 (Minitab Inc., State College, PA, USA) was used to carry out generalized mixed linear models (repeated measured) for the maximal photochemical efficiency of PSII, F v/F m. The fixed factors were: 1) colour (pale versus melanic); 2) freely exposed photobiont layer (scraped) versus an intact photobiont layer beneath an upper cortex; 3) time during recovery (0, 0·5, 1, 2, 4, 6 and 24 h). The random factor was thallus identity. To improve homoscedasticity and normality, F v/F m was expressed as percentage of start values.
Results
The BRI, assessing melanization, varied between the lichen species studied (Table 1). For example, in Lobaria the BRI of melanized thalli was about double that of pale thalli, while in Cetraria and Crocodia the BRI was about nine times greater. Cetraria had the darkest melanized thalli, and Crocodia the palest of the pale thalli.
Table 1. Browning reflectance index (BRI) of the lichens used in this study. Figures are given ± 1 SD, n = 12. BRI was calculated as described by Solovchenko et al. (Reference Solovchenko, Chivkunova, Merzlyak and Reshetnikova2001).

Prior to the light exposure, F v/F m varied slightly between species and tended to be rather similar in pale and melanized thalli (Table 2). However, the values of F v/F m of the photobionts measured from below (i.e. with the lower cortex removed) were on average 7% lower than those measured from above through the upper cortex. Differences between scraped and intact discs were significant in most cases, but not for melanized Cetraria and Crocodia. To facilitate comparisons between treatments, we present F v/F m in Fig. 2A–C as percentages of initial values.
Table 2. Initial mean values ± 1 SD of the F v/F m (maximal efficiency of PSII photochemistry) data presented in Fig. 2 as percentages (n = 12). Data was analyzed by ANOVA for each species followed by Duncan's multiple range test. Within each species, figures followed by the same letter do not differ significantly (P < 0·05).

Within 24 h, all three species recovered fully (Crocodia) or partly (Cetraria, Lobaria) from the high light-induced photoinhibition (Fig. 2A–C). Cetraria experienced the strongest photoinhibition. In all three species, intact melanized thalli were less photoinhibited. As a main effect in the general mixed linear analyses, melanins had highly significant effects on F v/F m in Cetraria only, with a weak effect in Lobaria and no effect in Crocodia (Table 3). The significant main effect of colour in Cetraria implies that both intact and scraped discs experienced less photoinhibition in melanic than in pale thalli (Fig. 2A). For intact discs of Lobaria, the melanic thalli recovered significantly faster than the pale ones, whereas recovery did not depend on pigmentation in scraped discs of this species. Scraping significantly exacerbated photoinhibition in high light in all species regardless of pigmentation (Fig. 2, Table 3; scraping as the main effect). Recovery time as a main factor was highly significant in all species (Table 3), and melanized thalli recovered significantly faster than pale thalli following exposure to high light (Fig. 2, Table 3; the colour × time interaction). In both Crocodia and Lobaria (Lobariaceae), the recovery from high light stress in thalli with the lower cortex and medulla surgically removed was very similar in melanized and pale thalli (Fig. 2B & C). By contrast, the photobionts in melanized Cetraria (Parmeliaceae) directly exposed to light were less inhibited and recovered significantly faster than those from pale thalli (Fig. 2A, Table 3).
Table 3. Generalized mixed linear models (repeated measured) for the maximal photochemical efficiency of PSII, F v/F m as percentage of start values in discs of Lobaria pulmonaria, Crocodia aurata and Cetraria islandica during recovery after high-light exposure. Fixed factors: 1, colour (pale versus melanic); 2, freely exposed photobiont layer (scraped) versus an intact photobiont layer beneath an upper cortex; 3, time during recovery after high-light treatment (0, 0·5, 1, 2, 4, 6 and 24 h). Random factor: thallus id.

Analyzed data are shown in Fig. 2A–C.
The random effect of thallus identity was highly significant for F v/F m, although with lower F-values than some fixed factors (Table 3). This shows that each thallus has its own characteristics, probably because of the specific field conditions experienced prior to collection.
Discussion
Earlier work (e.g. McEvoy et al. Reference McEvoy, Gauslaa and Solhaug2007; Mafole et al. Reference Mafole, Solhaug, Minibayeva and Beckett2019a) showed that photobionts in melanized lichens are more resistant to photoinhibition than those in pale ones, apparently due to fungal pigment synthesis. However, an inherent problem with these studies is that the melanized thalli were likely to have experienced stronger exposure to light than pale thalli. The photobionts of melanized thalli themselves might therefore have possessed other photoprotective mechanisms. In the present study, we separated photoprotective effects of the fungal solar radiation screen from photobiont acclimation traits by removing the lower cortex and medulla, and directly exposing the underlying algal layer to high light. For the two Lobariaceae species, which both have a photobiont from the genus Symbiochloris (Škaloud et al. Reference Škaloud, Friedl, Hallmann, Beck and Dal Grande2016), recovery from high light stress in thalli with the lower cortex and medulla surgically removed was similar in melanized and pale thalli (Fig. 2B & C). The third species used in the present study was the more heavily melanized Cetraria, which has a photobiont belonging to the genus Trebouxia (Thell & Moberg Reference Thell and Moberg2011; Onuț-Brännström et al. Reference Onuț-Brännström, Benjamin, Scofield, Heiðmarsson, Andersson, Lindström and Johannesson2018). In Cetraria, the photobionts from melanized thalli appear to possess significantly greater tolerance to high light stress than those from pale thalli (Fig. 2A), suggesting that some photobiont acclimation can take place. However, taken together, the results presented here show that in melanized thalli of the species studied, most of the improved tolerance of the photobiont to high light derives from fungal melanins in the upper cortex rather than algal acclimation.
Melanized thalli are more tolerant to high light stress than pale thalli
Even though sampled from an open habitat, Cetraria was more sensitive to photoinhibition than Crocodia at 900 μmol m−2 s−1 (Fig. 2A & B). This was presumably because the Crocodia used was from a subtropical region in South Africa where light intensities are on average higher than in Norway. In all three species, intact melanized thalli illuminated from above were less photoinhibited and recovered faster than intact pale thalli following exposure to high light (Fig. 2), consistent with the results of Mafole et al. (Reference Mafole, Solhaug, Minibayeva and Beckett2019a). Protection was related to the absolute difference in reflectance between the pale and melanized thalli (compare Table 1 and Fig. 2). For example, in Lobaria the increase in BRI was 1·9 and the protection offered was small but significant (Table 3), while in Cetraria the increase was 9·0 and the benefit much greater. Crocodia had intermediate values of both increase in BRI and photoprotection. The results presented here confirm earlier findings that melanized thalli are more tolerant to high light than pale thalli.
Even a non-melanized cortex provides protection from high light
In the pale thalli of all three species, removing the lower cortex and medulla and illuminating the thallus from below caused more photoinhibition than illuminating intact thalli from above (Fig. 2). Gauslaa & Solhaug (Reference Gauslaa and Solhaug2001) showed that in hydrated Lobaria pulmonaria, the cortex of even pale thalli reduced transmittance by up to 50% in the PAR range. Light filtering has two components, the first due to the physical presence of fungal hyphae which scatter light, and the second due to lichen substances. In pale thalli the main substances are lichen acids, which can reflect PAR as a consequence of their crystalline structure (Solhaug & Gauslaa Reference Solhaug and Gauslaa2012) and may additionally absorb them. This depends on the quantity and the spectral characteristics of the respective lichen substances (Solhaug et al. Reference Solhaug, Larsson and Gauslaa2010; Phinney et al. Reference Phinney, Gauslaa and Solhaug2019). While in L. pulmonaria, removal of readily extractable lichen substances by acetone rinsing only increased transmittance by c. 5%, this value can be much higher in lichens having cortical lichen substances with greater acetone solubility (Solhaug et al. Reference Solhaug, Larsson and Gauslaa2010). Thus the upper cortex, even of pale thalli, gives the photobiont some measure of protection against high light, explaining the greater tolerance of intact thalli to high light.
Most photoprotection in melanized thalli comes from cortical melanins
Our results indicate that the larger tolerance to high light in melanic compared with pale thalli occurs because sun-screening fungal melanins give more powerful photoprotection than algal acclimation traits. In the two Lobariaceae species, recovery from high light stress in thalli with the lower cortex and medulla surgically removed was similar in melanized and pale thalli (Fig. 2B & C). The lower photoinhibition in Lobaria than in Crocodia can likely be attributed to the lower light treatment used for this species (600 compared with 900 μmol m−2 s−1). If there had been a significant difference in photobiont tolerance to high light stress between melanized and pale members of these two species, then the photobionts of melanized thalli directly exposed to high light would have been expected to show greater tolerance than the photobionts of pale thalli. Possibly, differences in light levels at the photobiont layer under natural field conditions between pale and melanic lichens are sufficiently small that no acclimation of the Symbiochloris photobionts is needed. However, in Cetraria, the most melanized species tested here (Table 1), the Trebouxia photobionts in scraped discs possessed a significantly greater tolerance to high light stress in melanized than in pale thalli. The relatively high light levels that typically occur in the open habitats where Cetraria normally grows may exceed the capacity of the melanins to shield the Trebouxia from light. However, in melanic thalli of all three species, most of the increased tolerance to high light appears to derive from the melanins in their upper cortices.
Conclusions
Results presented here show that in Crocodia and Lobaria the photoprotective effect of melanization derives from sun-screening fungal pigments present in the upper cortex. However, in the more heavily melanized Cetraria the photobionts under a melanized upper cortex have greater tolerance to high light than those under a pale upper cortex. Lichen algae have various mechanisms of photoprotection (Verhoven et al. Reference Verhoven, García-Plazaola and Fernández-Marín2018), but more work is needed to elucidate which of these occur in Cetraria. A potentially useful supplementary approach to the surgical scraping used here could be to isolate algae from pale and melanized thalli and to study their adaptation to high light in the absence of any fungal tissues. Nevertheless, using the Imaging PAM was highly effective, allowing us to measure fluorescence parameters in areas of the thallus from which the lower cortex and medulla had been removed.
Richard Beckett thanks the University of KwaZulu-Natal for study leave to carry out the research at the Norwegian University of Life Sciences. Farida Minibayeva thanks the Russian Science Foundation (grant no. 18-14-00198) and the Russian Government Programme of Competitive Growth of Kazan Federal University for partial financial support.
Abbreviations
BRI – browning reflectance index; F m – maximum fluorescence; F o – minimal fluorescence yield of the dark adapted state; F v – variable fluorescence or (F m – F o); F v/F m – maximal efficiency of PSII photochemistry; NPQ – non-photochemical quenching; ROS – reactive oxygen species