Introduction
Respiratory diseases in pigs are common global problems for modern pork producers and are frequently associated with the porcine respiratory disease complex (PRDC) (Opriessnig et al., Reference Opriessnig, Giménez-Lirola and Halbur2011). PRDC is a multifactorial syndrome caused by the interaction of bacteria, viruses and stresses associated with management practices, environmental conditions and genetic predispositions (Opriessnig et al., Reference Opriessnig, Giménez-Lirola and Halbur2011; Schmidt et al., Reference Schmidt, Cibulski, Andrade, Teixeira, Valera, Scheffer, Franco, Almeida and Roehe2016). Within PRDC, Actinobacillus pleuropneumoniae is one of the most commonly identified bacterial pathogens that cause respiratory infections in pigs (Opriessnig et al., Reference Opriessnig, Giménez-Lirola and Halbur2011; Dayao et al., Reference Dayao, Gibson, Blackall and Turni2016). A. pleuropneumoniae is a Gram-negative rod-shaped bacterium belonging to the Pasteurellaceae family (Chiers et al., Reference Chiers, De Waele, Pasmans, Ducatelle and Haesebrouck2010; Gómez-Laguna et al., Reference Gómez-Laguna, Islas, Muñoz, Ruiz, Villamil, Carrasco and Quezada2014) and is the etiologic agent of porcine pleuropneumonia (Frey, Reference Frey1995; Buettner et al., Reference Buettner, Konze, Maas and Gerlach2011). This respiratory infection is the major cause of morbidity and mortality and is responsible for substantial economic losses worldwide (Chiers et al., Reference Chiers, De Waele, Pasmans, Ducatelle and Haesebrouck2010; Bossé et al., Reference Bossé, Li, Angen, Weinert, Chaudhuri, Holden, Williamson, Maskell, Tucker, Wren, Rycroft and Langford2014). The disease is characterized by an exudative, fibrinous, hemorrhagic, and necrotizing pneumonia and associated pleuritis (Chen et al., Reference Chen, Chien, Chang, Chen, Wu, Huang, Lee and Hsuan2011). Porcine pleuropneumonia is transmitted via aerosols or direct contact with infected animals including asymptomatic carriers (i.e. animals with a sub-clinical infection). Clinical infections may result into a chronic and persistent form, an acute form associated with the pathology described above or a peracute form associated with severe pathology and rapid death (Gottschalk, Reference Gottschalk2015).
In 1964, Shope was the first to describe a disease affecting pigs in Argentina as porcine contagious pleuropneumonia (PCP) and he named the causative agent Haemophilus pleuropneumoniae (Shope, Reference Shope1964; Shope et al., Reference Shope, White and Leidy1964). In 1983, Pohl and coworkers transferred the causative agents of PCP or similar infections to the genus Actinobacillus based on the higher DNA-sequence homology to the genus Actinobacillus (Actinobacillus lignieresii, 72–75%) (Pohl et al., Reference Pohl, Bertschinger, Frederiksen and Mannheim1983; Nicolet, Reference Nicolet1988). In 1986, O'Reilly and Niven identified the pyridine nucleotides, the precursors that were needed to satisfy the V-factor requirement, and the nicotinamide adenine dinucleotide (NAD) was identified as a supplement that supported in vitro growth (O'Reilly and Niven, Reference O'Reilly and Niven1986). A. pleuropneumoniae is now divided into two biotypes based on their NAD requirement for growth: biotype 1 is NAD-dependent, and biotype 2 is NAD-independent (Turni et al., Reference Turni, Singh, Schembri and Blackall2014; Gottschalk, Reference Gottschalk2015; Ito, Reference Ito2015).
A. pleuropneumoniae is further divided into 16 serotypes (or serovars) based on the antigenic properties of the capsular polysaccharides and the O-chain of the lipopolysaccharides (LPS) (Sárközi et al., Reference Sárközi, Makrai and Fodor2015; Kim et al., Reference Kim, Kim and Yang2016; Bossé et al., Reference Bossé, Li, Sárközi, Gottschalk, Angen, Nedbalcova, Rycroft, Fodor and Langford2017). Serotypes 1–12 and 15 typically belong to biotype 1, whereas serotypes 13 and 14 are typically biotype 2 (Serrano et al., Reference Serrano, Tenorio-Gutiérrez, Suárez, Reyes-Cortés, Rodríguez-Mendiola, Arias-Castro, Godínez-Vargas and de la Garza2008; Gottschalk, Reference Gottschalk2015). The serotype 16 is not yet officially grouped in any biotype. However, this is not an absolute rule since variants of serotype 2, 4, 7, 9 and 11 have been identified as NAD-independent (biotype 2) (Perry et al., Reference Perry, Angen, Maclean, Lacouture, Kokotovic and Gottschalk2012). Furthermore, there has been an increase in the prevalence of isolates that are untypable (UT) (Morioka et al., Reference Morioka, Shimazaki, Uchiyama and Suzuki2016). Despite the global distribution of A. pleuropneumoniae, the prevalence of different serotypes varies between countries (Morioka et al., Reference Morioka, Shimazaki, Uchiyama and Suzuki2016). Specifically, serotypes 1, 5, and 7 are predominantly found in North America, serotype 2 is the most common type in Europe and serotypes 1, 3, 4, 5, and 7 are typically isolated in China (Buettner et al., Reference Buettner, Konze, Maas and Gerlach2011; Gottschalk and Lacouture, Reference Gottschalk and Lacouture2015; Morioka et al., Reference Morioka, Shimazaki, Uchiyama and Suzuki2016). For South America, serotypes 4, 6, and 7 are reported as the dominant serotypes in the region (Gómez-Laguna et al., Reference Gómez-Laguna, Islas, Muñoz, Ruiz, Villamil, Carrasco and Quezada2014).
Infection and persistence of A. pleuropneumoniae are mediated by multiple virulence factors. Well-characterized virulence factors of A. pleuropneumoniae include: the Apx toxins (ApxI, ApxII, ApxIII and ApxIV), lipopolysaccharide (LPS), capsule polysaccharide (CPS), proteases (e.g. LonA), urease, iron acquisition systems (e.g. transferrin-binding protein [Tbp], haemoglobin-binding protein [HbpA]), enzymes involved in anaerobic respiration (e.g. two-component signal transduction system [TCSTS] arcB and arcA), type IV pilus, Flp pilus, autotransporters (e.g. Trimeric Autotransporter Adhesin [TAA]), and more recently biofilms (Chiers et al., Reference Chiers, De Waele, Pasmans, Ducatelle and Haesebrouck2010; Tremblay et al., Reference Tremblay, Labrie, Chénier and Jacques2017). The role of biofilm in persistence, survival, and pathogenesis of A. pleuropneumoniae is relatively new and the importance of biofilm is not fully understood. It has now been demonstrated that biofilms can develop during an infection and a recent report describes the growth of A. pleuropneumoniae as aggregates in lungs obtained from natural pig infections (Tremblay et al., Reference Tremblay, Labrie, Chénier and Jacques2017). In this review, our aim is to highlight and summarize the current knowledge on A. pleuropneumoniae biofilm formation and suggest its possible role in pathogenesis. Furthermore, we will also talk about vaccination and new strategies based on recent biofilm findings.
Biofilms and animal health
It is well accepted by the scientific community that most bacteria can produce biofilms in their natural ecosystem as well as in artificial in vitro ecosystems (Briandet et al., Reference Briandet, Fechner, Naïtali and Dreanno2012). Biofilms are defined as structured communities enclosed in a self-produced matrix that is attached to a surface (biotic or abiotic); however, recent evidence have demonstrated that in vivo biofilms and bacterial aggregates are not necessarily attached to the surface and are often embedded in host material (Bjarnsholt et al., Reference Bjarnsholt, Alhede, Eickhardt-Sorensen, Moser, Kühl, Jensen and Hoiby2013; Kragh et al., Reference Kragh, Hutchison, Melaugh, Rodesney, Roberts, Irie, Jensen, Diggle, Allen, Gordon and Bjarnsholt2016). Our group has extensively reviewed biofilm formation by animal and zoonotic pathogens, and we will not cover general information about biofilm in this review (see Jacques et al., Reference Jacques, Aragon and Tremblay2010). Several members of the Pasteurellaceae family, which include many important animal pathogens, are able to form biofilms and several studies in the past decade have demonstrated the ability of its members such as Haemophilus influenzae, Pasteurella multocida, Aggregatibacter actinomycetemcomitans, Mannheimia haemolytica, Histophilus somni, and Haemophilus parasuis to produce a biofilm (Olson et al., Reference Olson, Ceri, Morck, Buret and Read2002; Kaplan et al., Reference Kaplan, Velliyagounder, Ragunath, Rohde, Mack, Knobloch and Ramasubbu2004; Jin et al., Reference Jin, Zhou, Kang, Luo, Cai and Chen2006; Sandal et al., Reference Sandal, Hong, Swords and Inzana2007; Wu et al., Reference Wu, Labrie, Tremblay, Haine, Mourez and Jacques2013; Bello-Ortí et al., Reference Bello-Ortí, Deslandes, Tremblay, Labrie, Howell, Tucker, Maskell, Aragon and Jacques2014; Boukahil and Czuprynski, Reference Boukahil and Czuprynski2015). For several members of the Pasteurellaceae family, it has been suggested that biofilm formation is crucial for the persistence of these obligate inhabitants (Jin et al., Reference Jin, Zhou, Kang, Luo, Cai and Chen2006; Sandal et al., Reference Sandal, Hong, Swords and Inzana2007; Bello-Ortí et al., Reference Bello-Ortí, Deslandes, Tremblay, Labrie, Howell, Tucker, Maskell, Aragon and Jacques2014; Boukahil and Czuprynski, Reference Boukahil and Czuprynski2015). For example, non-virulent isolates of H. parasuis formed stronger and more robust biofilms than virulent isolates, suggesting that the biofilm phase favors colonization and the planktonic phase allows for the dissemination within the host (Jin et al., Reference Jin, Zhou, Kang, Luo, Cai and Chen2006; Bello-Ortí et al., Reference Bello-Ortí, Deslandes, Tremblay, Labrie, Howell, Tucker, Maskell, Aragon and Jacques2014).
A. pleuropneumoniae biofilms
The ability of A. pleuropneumoniae to form biofilms in vitro was first studied using a 96-well microtiter plate model (Coffey and Anderson, Reference Coffey and Anderson2014) (Fig. 1). Kaplan et al. (Reference Kaplan, Velliyagounder, Ragunath, Rohde, Mack, Knobloch and Ramasubbu2004) were the first to report that serotype 5b and 11 are producers of biofilms in vitro (Kaplan et al., Reference Kaplan, Velliyagounder, Ragunath, Rohde, Mack, Knobloch and Ramasubbu2004). A. pleuropneumoniae biofilms have also been assessed in glass tubes and under agitation. Biofilms form a ring at the air/liquid interface in this closed system model that incorporates shear force (Kaplan and Mulks, Reference Kaplan and Mulks2005). The ability to form biofilms appears to be common among A. pleuropneumoniae isolates because studies demonstrate that isolates from every serotype are able to produce biofilms in microtiter plates and/or glass tubes (Kaplan and Mulks, Reference Kaplan and Mulks2005; Labrie et al., Reference Labrie, Pelletier-Jacques, Deslandes, Ramjeet, Auger, Nash and Jacques2010). In the case of the newly reported serotype 16, the ability to form biofilms has yet to be studied.
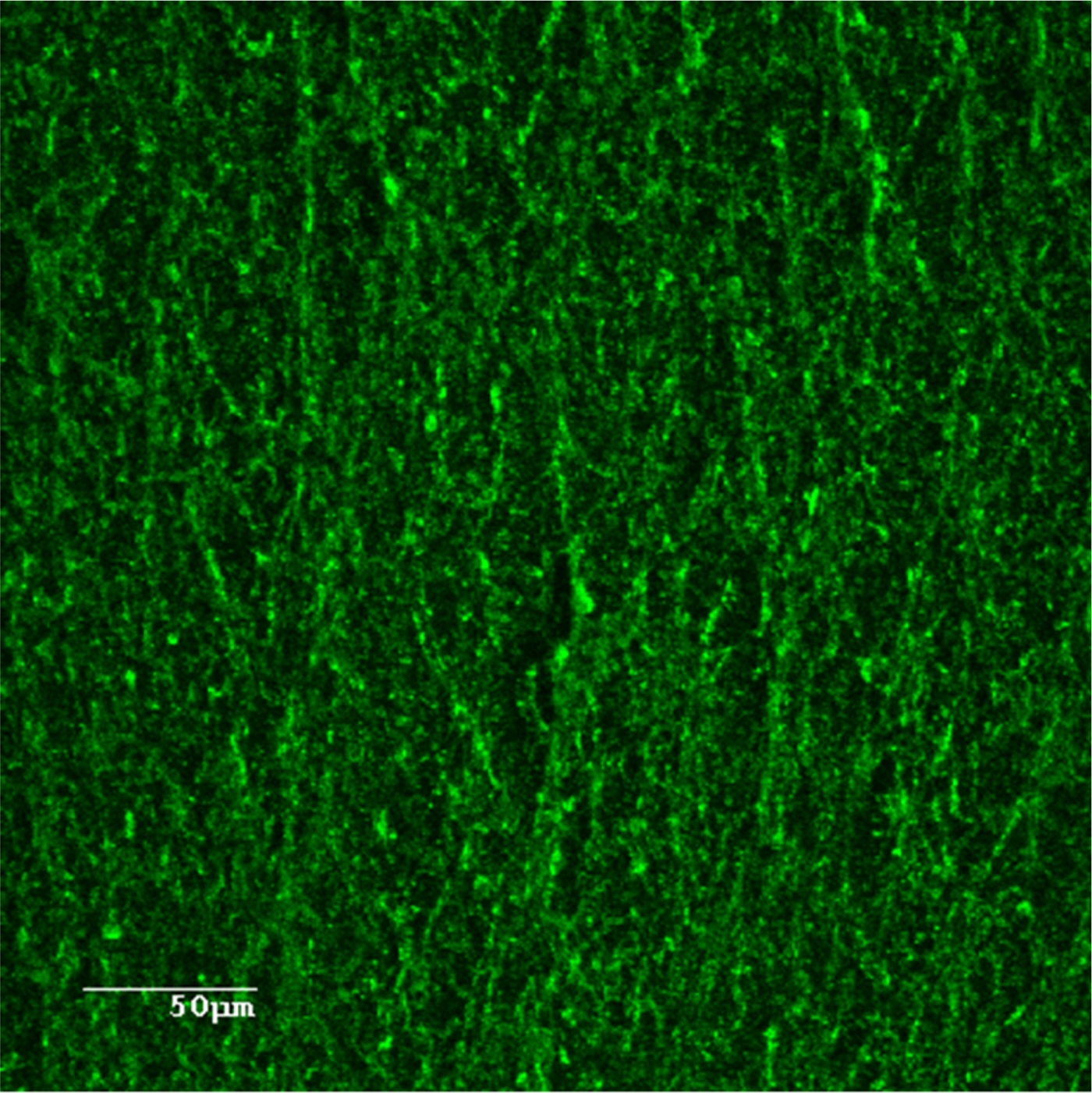
Fig. 1. Confocal laser scanning microscopy image of A. pleuropneumoniae 4074 biofilm stained with WGA-Oregon Green 488.
Biofilm formation in microtiter plates
In general, the production of biofilm by A. pleuropneumoniae in microtiter plates is described as a rapid process with the detection of biomass as early as 3 h for serotype 1 type strain S4074 and 6 h for serotype 5b type strain L20 and clinical isolates (Labrie et al., Reference Labrie, Pelletier-Jacques, Deslandes, Ramjeet, Auger, Nash and Jacques2010; Tremblay et al., Reference Tremblay, Deslandes and Jacques2013a). Interestingly, the biofilm cycle of serotype 1 type strain S4074 is completed within 8 h. Specifically, biomass becomes detectable after 3 h and reaches its peak at 5 h, which corresponds to the mature form of the biofilm (Tremblay et al., Reference Tremblay, Deslandes and Jacques2013a). Dispersion of the biofilm begins between 5 and 6 h and the biomass is no longer detectable after 8 h (Tremblay et al., Reference Tremblay, Deslandes and Jacques2013a) (Fig. 2). The biofilm persistence can be extended if the spent medium is removed and fresh culture medium is added to a 4-h old biofilm (i.e., a maturing biofilm). The change of growth medium can cause an increase in biomass and delay biofilm dispersion by 1 h. This suggests that depletion of the culture medium or the accumulation of one or several signals molecules can activate biofilm dispersal (Tremblay et al., Reference Tremblay, Deslandes and Jacques2013a). These observations provide a good example for the limitations of closed biofilm systems.

Fig. 2. Coupon with A. pleuropneumoniae 4074 biofilm from Drip flow system.
Biofilm formation in models with biologically relevant parameters
To overcome the limitations of the microtiter plates, dynamic models are often used and these systems are thought to be more representative of the conditions encountered by bacteria in their natural environment (Coenye and Nelis, Reference Coenye and Nelis2010). For example, the ‘drip flow’ reactor is a continuous flow system that continuously irrigates biofilms with fresh medium and allows biofilms to form on a coupon of choice (e.g., glass, stainless steel, PVC) that is deposited inside a sealed chamber (Goeres et al., Reference Goeres, Hamilton, Beck, Buckingham-Meyer, Hilyard, Loetterle, Lorenz, Walker and Stewart2009). In this model, biofilms are formed at the air/liquid interface in the presence of low shear forces that mimic the environment found in the lung and oral cavities (Goeres et al., Reference Goeres, Hamilton, Beck, Buckingham-Meyer, Hilyard, Loetterle, Lorenz, Walker and Stewart2009; Schwartz et al., Reference Schwartz, Stephenson, Hernandez, Jambang and Boles2010). Unlike the results obtained with the microtiter plates, A. pleuropneumoniae S4074 is able to establish and maintain a biofilm for up to 48 h (Tremblay et al., Reference Tremblay, Deslandes and Jacques2013a). To grow biofilms under these conditions, the growth medium (Brain Heart Infusion [BHI] with NAD) is diluted to 50% and the flow can be set from 50 to 200 ml per hour per chamber (Tremblay et al., Reference Tremblay, Deslandes and Jacques2013a; Hathroubi et al., Reference Hathroubi, Hancock, Bossé, Langford, Tremblay, Labrie and Jacques2016a). After 24 h, A. pleuropneumoniae forms an important biomass on a glass slide that is visible with the naked eye (Fig. 2). This biofilm contains 109–1010 colonies forming units (CFU) per chamber with an average dry weight of 10 mg (Tremblay et al., Reference Tremblay, Deslandes and Jacques2013a; Hathroubi et al., Reference Hathroubi, Hancock, Bossé, Langford, Tremblay, Labrie and Jacques2016a). Although the ‘drip-flow’ reactor provides a dynamic environment that resembles the lung cavity, the surface used was a microscopic slide, a substrate that A. pleuropneumoniae would never encounter in vivo.
In order to see if a biotic surface could be used by A. pleuropneumoniae, Tremblay and colleagues al. (Reference Tremblay, Lévesque, Segers and Jacques2013b) investigated biofilm formation on a SJPL cell line by a non-hemolytic, non-cytotoxic mutant of strain S4074, called MBHPP147. This mutant has deletions in both the apxIC and apxIIC genes, which prevents the acylation (and hence activation) of the protoxins ApxIA and ApxIIA. As observed with strain S4074, MBHPP147 is able to form a biofilm on polystyrene in microtiter plates. Furthermore, a robust biofilm is observed after 24 and 48 h of contact with the SJPL cells (Tremblay et al., Reference Tremblay, Lévesque, Segers and Jacques2013b). These studies are consistent with the notion that A. pleuropneumoniae can form biofilms on biotic surfaces during host colonization.
Recently, A. pleuropneumoniae biofilm formation was studied using an embedded model created with 0.5% agarose. This porous substrate is thought to simulate the conditions found in the lungs during a natural infection (Tremblay et al., Reference Tremblay, Labrie, Chénier and Jacques2017). Biofilm formation in this model was tested with two clinical isolates of A. pleuropneumoniae (one serotype 5, and one serotype 7) that were previously shown to form biofilms in a 96-wells plate and aggregates in the lungs of naturally infected pigs. In the embedded models, both isolates developed aggregates ranging from 20 to 30 microns within the porous matrix formed by the agarose. The size of the aggregates and their structure were similar to those observed in the lungs of pigs naturally infected by either isolate (30–45 μm) (Tremblay et al., Reference Tremblay, Labrie, Chénier and Jacques2017). The use of this new model that mimics the pulmonary alveolus environment during an infection has a promising future and could provide a new platform to test the sensitivity of A. pleuropneumoniae biofilm to several antibiotics.
Factors involved in the formation and dispersion of A. pleuropneumoniae biofilms
Several strategies have been used to identify genetic factors associated with biofilm formation. For example, a library of mini-Tn10 transposon mutants in A. pleuropneumoniae S4074 was screened in a 96-well microplate assay and 16 genes affecting biofilm formation were identified (Grasteau et al., Reference Grasteau, Tremblay, Labrie and Jacques2011). Otherwise, microarrays have also been used to gain insight into the transcriptome of maturing or dispersing biofilms formed under static or dynamic conditions (Tremblay et al., Reference Tremblay, Deslandes and Jacques2013a). These approaches provide a different insight into the biofilm formation process. The results are summarized in the sections below.
Composition of the biofilm matrix
Poly-N-acetyl-glucosamine (PGA) is the major component and an essential element of the A. pleuropneumoniae biofilm matrix, regardless of the growth conditions and surfaces used (Fig. 1) (Izano et al., Reference Izano, Sadovskaya, Vinogradov, Mulks, Velliyagounder, Ragunath, Kher, Ramasubbu, Jabbouri, Perry and Kaplan2007; Bossé et al., Reference Bossé, Sinha, Li, O'Dwyer, Nash, Rycroft, Kroll and Langford2010; Labrie et al., Reference Labrie, Pelletier-Jacques, Deslandes, Ramjeet, Auger, Nash and Jacques2010; Tremblay et al., Reference Tremblay, Deslandes and Jacques2013a, Reference Tremblay, Lévesque, Segers and Jacquesb; Hathroubi et al., Reference Hathroubi, Fontaine-Gosselin, Tremblay, Labrie and Jacques2015, Reference Hathroubi, Hancock, Bossé, Langford, Tremblay, Labrie and Jacques2016a). The proteins responsible for PGA synthesis are encoded by the pgaABCD operon (Kaplan et al., Reference Kaplan, Velliyagounder, Ragunath, Rohde, Mack, Knobloch and Ramasubbu2004; Izano et al., Reference Izano, Sadovskaya, Vinogradov, Mulks, Velliyagounder, Ragunath, Kher, Ramasubbu, Jabbouri, Perry and Kaplan2007). This operon is highly prevalent among A. pleuropneumoniae serotypes and appears to have been preserved in every studied serotype (Izano et al., Reference Izano, Sadovskaya, Vinogradov, Mulks, Velliyagounder, Ragunath, Kher, Ramasubbu, Jabbouri, Perry and Kaplan2007). In the studies by Izano et al. (Reference Izano, Sadovskaya, Vinogradov, Mulks, Velliyagounder, Ragunath, Kher, Ramasubbu, Jabbouri, Perry and Kaplan2007), PCR analysis of the gene coding for the biosynthesis of PGA, pgaC, demonstrated that it was present in every reference strains investigated (serotypes 1–12) and in 76 of the 77 field isolates tested. The synthesis of PGA is essential for the biofilm formation process and deleting one gene in the operon, pgaC, completely abolishes the production of PGA and, thus, prevents biofilm formation (Izano et al., Reference Izano, Sadovskaya, Vinogradov, Mulks, Velliyagounder, Ragunath, Kher, Ramasubbu, Jabbouri, Perry and Kaplan2007; Bossé et al., Reference Bossé, Sinha, Li, O'Dwyer, Nash, Rycroft, Kroll and Langford2010; Hathroubi et al., Reference Hathroubi, Hancock, Bossé, Langford, Tremblay, Labrie and Jacques2016a).
A. pleuropneumoniae can also control the degradation of the self-produced PGA polymers using a glycoside hydrolase, dispersin B (Izano et al., Reference Izano, Sadovskaya, Vinogradov, Mulks, Velliyagounder, Ragunath, Kher, Ramasubbu, Jabbouri, Perry and Kaplan2007). This enzyme can detach biofilms formed on difference surfaces, under different conditions and in different model systems (Izano et al., Reference Izano, Sadovskaya, Vinogradov, Mulks, Velliyagounder, Ragunath, Kher, Ramasubbu, Jabbouri, Perry and Kaplan2007; Labrie et al., Reference Labrie, Pelletier-Jacques, Deslandes, Ramjeet, Auger, Nash and Jacques2010; Tremblay et al., Reference Tremblay, Deslandes and Jacques2013a, Reference Tremblay, Lévesque, Segers and Jacquesb; Hathroubi et al., Reference Hathroubi, Fontaine-Gosselin, Tremblay, Labrie and Jacques2015, Reference Hathroubi, Hancock, Bossé, Langford, Tremblay, Labrie and Jacques2016a).
Other components, such as extracellular DNA (eDNA) and proteins, may also provide building blocks for the matrix. Proteins and eDNA have been stained and observed by confocal microscopy in the biofilm formed by A. pleuropneumoniae (Wu et al., Reference Wu, Labrie, Tremblay, Haine, Mourez and Jacques2013; Hathroubi et al., Reference Hathroubi, Hancock, Bossé, Langford, Tremblay, Labrie and Jacques2016a). Under most conditions tested, these components do not appear to be required for the integrity of the biofilm matrix, because proteinase K or DNase does not disperse pre-established biofilms (Grasteau et al., Reference Grasteau, Tremblay, Labrie and Jacques2011; Hathroubi et al., Reference Hathroubi, Hancock, Bossé, Langford, Tremblay, Labrie and Jacques2016a). However, eDNA might contribute to the integrity of the biofilm under certain conditions such as in the presence of sub-minimal inhibitory concentration of penicillin B or in multi-species biofilms (Loera-Muro et al., Reference Loera-Muro, Jacques, Avelar-González, Labrie, Tremblay, Oropeza-Navarro and Guerrero-Barrera2016; Hathroubi et al., Reference Hathroubi, Beaudry, Provost, Martelet, Segura, Gagnon and Jacques2016b).
Growth medium and other conditions inducing biofilm formation
The composition of the culture medium affects A. pleuropneumoniae biofilm formation. For example, Li and collaborators in 2008 demonstrated that the reference strain S4074 only produced a biofilm in TSB (Tryptic Soy Broth) medium in the absence of serum although the mechanism of this inhibition remains to be determined (Li et al., Reference Li, Zhou, Li, Kang, Wan, Xu and Chen2008). Later, Labrie et al. (Reference Labrie, Pelletier-Jacques, Deslandes, Ramjeet, Auger, Nash and Jacques2010) demonstrated that BHI medium favored biofilm formation of A. pleuropneumoniae S4074 when compared with TSB. Further, 54% of serotypes 1, 5, 7, and 15 strains produced biofilms in BHI, reinforcing the idea that BHI would be better for the study of biofilms in vitro. However, the source of the BHI medium also has an impact on biofilm formation. For example, BHI from Oxoid enhanced the production of robust biofilms, whereas BHI from Difco does not promote biofilm formation (Labrie et al., Reference Labrie, Pelletier-Jacques, Deslandes, Ramjeet, Auger, Nash and Jacques2010).
When the compositions of both media were analyzed, the concentration of zinc was identified as a key difference with higher levels in BHI-Difco than BHI-Oxoid (Labrie et al., Reference Labrie, Pelletier-Jacques, Deslandes, Ramjeet, Auger, Nash and Jacques2010). In support of these observations, researchers have shown that the addition of zinc to BHI-Oxoid inhibits biofilm formation in a dose-dependent manner without affecting bacterial growth (Labrie et al., Reference Labrie, Pelletier-Jacques, Deslandes, Ramjeet, Auger, Nash and Jacques2010; Wu et al., Reference Wu, Labrie, Tremblay, Haine, Mourez and Jacques2013). Thus, zinc appears to specifically inhibit the production of biofilm by A. pleuropneumoniae. A similar inhibitory effect has also been observed for other porcine pathogens such as Escherichia coli, Salmonella Typhimurium, Staphylococcus aureus and Streptococcus suis (Wu et al., Reference Wu, Labrie, Tremblay, Haine, Mourez and Jacques2013). In A. pleuropneumoniae, the presence of zinc might interfere with the expression or biosynthesis of the major polymer found in the biofilm matrix, PGA, because the expression of the pgaABCD operon is up-regulated in BHI-Oxoid (Labrie et al., Reference Labrie, Pelletier-Jacques, Deslandes, Ramjeet, Auger, Nash and Jacques2010) and zinc inhibits the activity of PgaB in E scherichia coli (Little et al., Reference Little, Poloczek, Whitney, Robinson, Nitz and Howell2012).
In addition to the growth medium, anaerobic conditions also appear to induce biofilm formation (Li et al., Reference Li, Zhu, Yang, Xu, Liu and Zhou2014). Indeed, exposure to anaerobic conditions results in an increase in biofilm formation that is associated with the up-regulation of the fine tangled pili major subunit gene (ftpA) and pgaA (Li et al., Reference Li, Zhu, Yang, Xu, Liu and Zhou2014).
Other growth conditions appear to induce the expression of biofilm-associated genes. For example, direct contact of A. pleuropneumoniae with epithelial cells results in an increased expression of the pgaABCD operon (Auger et al., Reference Auger, Deslandes, Ramjeet, Contreras, Nash, Harel, Gottschalk, Olivier and Jacques2009). Further, epinephrine and norepinephrine affect the expression of pgaB and Apa1, an auto-transporter adhesin (Li et al., Reference Li, Xu, Zhou, Sun, Liu, Chen and Zhou2012). However, only norepinephrine induces enhanced attachment to SJPL cells and neither catecholamine has an impact on biofilm formation (Li et al., Reference Li, Xu, Zhou, Sun, Liu, Chen and Zhou2012). It is conceivable that different factors play a role during the attachment of A. pleuropneumoniae to a biotic surface (e.g. SJPL cells) and an abiotic surface (e.g. polystyrene or glass). In support of this statement, A. pleuropneumoniae does not form a biofilm on polystyrene when grown in a cell culture medium (Dulbecco's modified Eagle's medium [DMEM]) and was only able to form biofilm in the presence of SJPL cells in DMEM (Tremblay et al., Reference Tremblay, Lévesque, Segers and Jacques2013b).
The biofilm transcriptome
The transcriptomes of maturing (static 4 h), mature (drip-flow), and dispersing (static 6 h) biofilms have been analyzed and compared with each other and to their planktonic counterparts. In a study by Tremblay et al. (Reference Tremblay, Deslandes and Jacques2013a), only 47 and 117 genes were differentially up- or down-regulated in static biofilms when compared with planktonic cells. For example, biofilm bacteria down-regulated the expression of their energy metabolism genes when compared with planktonic bacteria (Tremblay et al., Reference Tremblay, Deslandes and Jacques2013a). Indeed, the majority of energy metabolism genes such as the genes encoding the key enzymes of the anaerobic metabolism appeared to be repressed in the biofilm (Tremblay et al., Reference Tremblay, Deslandes and Jacques2013a).
Major differences have also been observed when the maturing biofilm is compared with a dispersing biofilm. Specifically, 456 genes were differently regulated when a maturing biofilm and a dispersing biofilm were compared (Tremblay et al., Reference Tremblay, Deslandes and Jacques2013a). Furthermore, the maturing biofilm appears to be under an iron-rich condition because several major genes in iron acquisition, including tbpB, are repressed in the maturing biofilm (Tremblay et al., Reference Tremblay, Deslandes and Jacques2013a).
Interestingly, a comparative analysis reveals that the transcriptome of drip-flow biofilms shares few differentially expressed genes with static biofilms. On the other hand, the drip-flow transcriptome has several genes that have also been identified in natural or experimental infections of pigs (Tremblay et al., Reference Tremblay, Deslandes and Jacques2013a). Transcriptome and cross-referencing analyses indicate that biofilms formed in drip-flow models require a different sub-set of genes than biofilms grown in microtiter plates (Tremblay et al., Reference Tremblay, Deslandes and Jacques2013a). Based on these results, it has been suggested that the drip-flow apparatus might provide a more relevant model to study biofilm formation by A. pleuropneumoniae (Tremblay et al., Reference Tremblay, Deslandes and Jacques2013a).
Regulators of biofilm formation
While environmental conditions and growth medium composition that are optimal for biofilm formation and induce production of PGA have been identified, other studies have identified potential regulators and molecular mechanisms associated with biofilm formation. In addition to growth conditions, the expression of the pgaABCD genes and, consequently, PGA production are regulated by the histone type H-NS (histone-like protein), which acts as a repressor of expression and hence a suppressor of biofilm production (Dalai et al., Reference Dalai, Zhou, Wan, Kang, Li, Li, Zhang and Chen2009; Bossé et al., Reference Bossé, Sinha, Li, O'Dwyer, Nash, Rycroft, Kroll and Langford2010; Grasteau et al., Reference Grasteau, Tremblay, Labrie and Jacques2011). Tn insertions in the hns gene of A. pleuropneumoniae serotype 1 results in a sharp increase in biofilm formation and a loss of virulence (Dalai et al., Reference Dalai, Zhou, Wan, Kang, Li, Li, Zhang and Chen2009). Indeed, H-NS specifically represses the expression of the operon by binding sequences upstream the pgaA gene (Bossé et al., Reference Bossé, Sinha, Li, O'Dwyer, Nash, Rycroft, Kroll and Langford2010). The importance of hns in repressing biofilm formation has also been independently confirmed in a screen that identified three Tn-mutants with an increase biofilm production (Grasteau et al., Reference Grasteau, Tremblay, Labrie and Jacques2011). Unlike H-NS, the alternative sigma factor RpoE (or σE) is a transcriptional activator of the pgaABCD operon (Bossé et al., Reference Bossé, Sinha, Li, O'Dwyer, Nash, Rycroft, Kroll and Langford2010).
Deletion of the gene encoding the negative regulator of the σ E factor, RseA (regulator of sigma-E), results in increased expression of the pgaABDC operon and higher biofilm production (Bossé, et al., Reference Bossé, Sinha, Li, O'Dwyer, Nash, Rycroft, Kroll and Langford2010). Additionally, expression of the pgaABCD operon is also under the control of the RNA chaperone Hfq (Subashchandrabosea et al., Reference Subashchandrabosea, Leveque, Kirkwoodc, Kiupela and Mulksa2013). Disruption of hfq decreases PGA production, biofilm formation, virulence and fitness (Subashchandrabosea et al., Reference Subashchandrabosea, Leveque, Kirkwoodc, Kiupela and Mulksa2013).
Deletion of the quorum-sensing (QS) gene also results in an increase in pgaABC expression, a strong increase in biofilm production and a decrease in virulence (Li et al., Reference Li, Zhou, Li, Kang, Wan, Xu and Chen2008, Reference Li, Xu, Zhou, Li, Sun, Chen and Zhou2011). S-ribosylhomocysteine lyase (LuxS), is a protein involved in the production of the auto-inducer type 2 (AI-2) and in the QS mechanism. QS is involved in the biofilm formation in many bacteria (Prouty et al., Reference Prouty, Schwesinger and Gunn2002; Merritt et al., Reference Merritt, Qi, Goodman, Anderson and Shi2003; Ethapa et al., Reference Ethapa, Leuzzi, Ng, Baban, Adamo, Kuehne, Scarselli, Minton, Serruto and Unnikrishnan2013). The increased biofilm production in A. pleuropneumoniae appears, however, to be independent of the production of AI-2, because the addition of AI-2 to the culture medium results in an increase biofilm production in the absence of LuxS (Li et al., Reference Li, Xu, Zhou, Li, Sun, Chen and Zhou2011). Enhanced biofilm formation has also been observed in a mutant lacking the relA, a gene encoding the stringent response regulatory protein responsible for the synthesis of (p)ppGpp (Li et al., Reference Li, Xie, Zhang, Bossé, Langford and Wang2015). This deletion results in the up-regulation of a fimbrial biogenesis protein and tight adherence protein; proteins thought are important for adhesion to surfaces (Li et al., Reference Li, Xie, Zhang, Bossé, Langford and Wang2015).
In addition to quorum sensing and the stringent response, the two-component regulatory system also controls biofilm formation in A. pleuropneumoniae. For example, deletion of the ArcA, which belongs to the ArcAB two-component system, causes a defect in autoaggregation and biofilm formation (Buettner et al., Reference Buettner, Maas and Gerlach2008). Furthermore, the expression of the cpxA, a gene encoding the histidine kinase of the CpxRA stress response system, is induced in bacteria grown in biofilm when compared with their planktonic counterparts (Tremblay, et al., Reference Tremblay, Deslandes and Jacques2013a). In E. coli, this system is induced during the biofilm maturation phase (Otto and Silhavy, Reference Otto and Silhavy2002) and the CpxRA system can be activated by mechanical pressure (Vogt and Raivio, Reference Vogt and Raivio2012). It has been suggested that such pressure could be encountered by bacteria during the initial attachment and biofilm formation, and could activate the CpxRA stress response. Interestingly, an O-antigen mutant, which lost its ability to produce a biofilm, exhibits reduced expression of cpxRA (Hathroubi et al., Reference Hathroubi, Hancock, Bossé, Langford, Tremblay, Labrie and Jacques2016a). Furthermore, enhanced biofilm production induced by a sub-minimal inhibitory concentration (MIC) of penicillin G is associated with increased cpxRA expression (Hathroubi et al., Reference Hathroubi, Fontaine-Gosselin, Tremblay, Labrie and Jacques2015). In both cases described above, the expression of pgaA is also affected in the same direction, suggesting a link between the CpxRA response and pgaABCD expression. Overall, activation of the A. pleuropneumoniae CpxRA system appears to occur during biofilm formation; however, the link between the CpxRA system, pgaABCD expression, and biofilm formation requires further investigation before this could be said definitively.
Surface-associated proteins and polysaccharides
Proteins and polysaccharides located at the bacterium/surface interface are crucial for facilitating attachment, microcolony formation or subsequent maturation of the biofilm. Several proteins and polysaccharides have been identified and characterized as important for biofilm formation. In addition to the biofilm matrix polysaccharides, other surface polysaccharides have an impact on biofilm formation. For example, inactivation of galU results in an increase biofilm production (Grasteau et al., Reference Grasteau, Tremblay, Labrie and Jacques2011). The galU gene encodes an UTP-α-D-glucose-1-phosphate uridylyltransferase, an enzyme involved in the biosynthesis of the lipopolysaccharide core oligosaccharide in A. pleuropneumoniae (Ramjeet et al., Reference Ramjeet, Cox, Hancock, Mourez, Labrie, Gottschalk and Jacques2008). Further, the wecABD operon and the genes encoding proteins involved in the biosynthesis of lipopolysaccharide O antigen are induced in a mature biofilm (Tremblay et al., Reference Tremblay, Deslandes and Jacques2013a).
Recently, it was demonstrated that the absence of the O antigen markedly reduces the ability of A. pleuropneumoniae to form a mature biofilm. This decrease is associated with a reduction in pgaA expression and, consequently, PGA production (Hathroubi et al., Reference Hathroubi, Hancock, Bossé, Langford, Tremblay, Labrie and Jacques2016a). Interestingly, LPS and O-antigen-truncated LPS specifically bind PGA, suggesting that interactions between LPS and PGA may help bacterial cells attached to the biofilm matrix. Taken together, these observations reinforce the idea that LPS may play a role in biofilm formation of A. pleuropneumoniae. Several studies have shown the importance of O chains in biofilm formation by other Gram-negative bacteria such as Stenotrophomonas maltophilia (Huang et al., Reference Huang, Somers and Wong2006), Xanthomonas citri ssp. citri (Li and Wang, Reference Li and Wang2011), Xanthomonas oryzae pv. oryzicola (Wang et al., Reference Wang, Vinogradov and Bogdanove2013) and Xylella fastidiosa (Clifford et al., Reference Clifford, Rapicavoli and Roper2013). Although LPS may have a key role in biofilm formation, the capsule polysaccharides do not appear to affect biofilm formation despite an increase in adherence to epithelial cells and polystyrene by a capsule mutant (Rioux et al., Reference Rioux, Galarneau, Harel, Kobisch, Frey, Gottschalk and Jacques2000; Hathroubi et al., Reference Hathroubi, Hancock, Bossé, Langford, Tremblay, Labrie and Jacques2016a). The capsule may mask critical adhesion factors such as adhesins. Several surface proteins have been associated with biofilm formation in A. pleuropneumoniae. For example, deletion of the autotransporter serine protease, AasP, results in increased adherence and biofilm formation (Tegetmeyer et al., Reference Tegetmeyer, Fricke and Baltes2009). The outer membrane protein VacJ is also involved in biofilm formation and outer membrane integrity (Xie et al., Reference Xie, Li, Zhang, Zhang, Zhou, Liu, Liu and Wang2016a); deletion of this gene reduces the ability of A. pleuropneumoniae to form biofilms. Interestingly, outer membrane efflux proteins, such as TolC or a TolC-like homolog, have also been associated with biofilm formation. Moreover, it has been observed that the deletion of tolC1 causes a reduction in surface adherence, autoaggregation, and biofilm production but the second tolC homolog, tolC2, does not have any effect on biofilm formation (Li et al., Reference Li, Cao, Zhang, Yuan, Lau, Wen, Wu, Zhao, Huang, Yan, Huang and Wen2016a, Reference Li, Cao, Zhang, Lau, Wen, Wu, Zhao, Huang, Yan, Huang and Wenb). The cell hydrophobicity is also changed in the tolC1 deletion mutant and pgaA and cpxR expression is down-regulated in the mutant (Li et al., Reference Li, Cao, Zhang, Yuan, Lau, Wen, Wu, Zhao, Huang, Yan, Huang and Wen2016a). As a side note, the tolC2 gene is up-regulated in dispersing biofilms and it has been suggested that this protein with MacAB-like proteins could mediate secretion of a dispersal signal (Tremblay et al., Reference Tremblay, Deslandes and Jacques2013a). Interestingly, the efflux pump inhibitor, phenylalanine-arginine beta-naphthylamide (PAβN), is able to repress biofilm formation of A. pleuropneumoniae and enhance the inhibitory effect of several antibiotics on pre-established biofilms (Li et al., Reference Li, Cao, Zhang, Lau, Wen, Wu, Zhao, Huang, Yan, Huang and Wen2016b).
Two trimeric autotransporter adhesins, Apa1 and Apa2, are also involved in autoaggregation and biofilm formation of A. pleuropneumoniae (Xiao et al., Reference Xiao, Zhou, Sun, Feng, Du, Gao, Ji, Yang, Wang, Han, Langford and Lei2012; Wang et al., Reference Wang, Qin, Zhang, Bao, Zhang, Che, Sun, Gu, Feng, Du, Han, Richard and Lei2016). In the case of Apa1, the adhesion functional domain located at the head of the protein is required for autoaggregation, biofilm formation and adherence to SJPL (Wang et al., Reference Wang, Qin, Yang, Zhai, Zhou, Sun, Pan, Ji, Wang, Gu, Feng, Du, Han, Langford and Lei2015). Apa1 is a Hsf-like trimeric autotransporter adhesin that has been identified to be differentially regulated under several conditions. For example, Apa1, also identified as APL_0443, is up-regulated when A. pleuropneumoniae is cultured in a growth medium favoring biofilm formation (Labrie et al., Reference Labrie, Pelletier-Jacques, Deslandes, Ramjeet, Auger, Nash and Jacques2010), in the presence of norepinephrine (Li et al., Reference Li, Xu, Zhou, Sun, Liu, Chen and Zhou2012) and in the presence of porcine bronchoalveolar lavage fluid (Lone et al., Reference Lone, Deslandes, Nash, Jacques and Macinnes2009) while it is down-regulated in A. pleuropneumoniae attached to SJPL cells (Auger et al., Reference Auger, Deslandes, Ramjeet, Contreras, Nash, Harel, Gottschalk, Olivier and Jacques2009), in a maturing biofilm (Tremblay et al., Reference Tremblay, Deslandes and Jacques2013a) and in the presence of epinephrine (Li et al., Reference Li, Xu, Zhou, Sun, Liu, Chen and Zhou2012). Based on these observations, it was suggested that APL_0443 is involved in the early reversible attachment step during biofilm formation of A. pleuropneumoniae (Tremblay et al., Reference Tremblay, Deslandes and Jacques2013a).
Other factors identified
Factors involved in biofilm formation are not limited to regulators and structures at the bacteria/surface interface; the periplasm and cytoplasm have also been identified as the location of key processes for biofilm formation. For example, ClpP, a protease of the CLP (caseinolytic protease) family, plays an important role in biofilm formation of A. pleuropneumoniae. Indeed, a clpP deletion mutant has been shown to have a defect in biofilm production (Xie et al., Reference Xie, Zhang, Li, Liu and Wang2013). Other proteases also influence biofilm formation by A. pleuropneumoniae. Specifically, two homologs of the Lon proteases, LonA and LonC, have been identified but only the deletion of LonA results in decreased biofilm production (Xie et al., Reference Xie, Li, Zhang, Zhou, Liu, Liu and Wang2016b). The Lon proteases belong to a family of ATP-dependent proteases involved in the degradation of abnormal proteins created when bacteria are exposed to environmental stresses.
Furthermore, mutations in genes such potD2, a dihydrouridine tRNA that binds polyamine/spermidine, and rpmF, a ribosomal L32 protein, caused a decrease in the production of A. pleuropneumoniae biofilm (Grasteau et al., Reference Grasteau, Tremblay, Labrie and Jacques2011). Homologs of these genes have been associated with Pseudomonas aeruginosa biofilm and their mutations decrease biofilm production (Musken et al., Reference Musken, Di Fiore, Dotsch, Fischer and Haussler2010). Other genes such as pyrF (decarboxylase orotidine-5-phosphate), ptsI (phosphotransferase), and ribA (synthesis of riboflavin), are also associated with a decrease in biofilm formation in A. pleuropneumoniae (Grasteau et al., Reference Grasteau, Tremblay, Labrie and Jacques2011). Also, riboflavin synthesis appears to be an important element in biofilm formation since the expression of certain genes in this pathway are modulated during biofilm formation (Tremblay et al., Reference Tremblay, Deslandes and Jacques2013a).
Biofilms: advantages and benefits for A. pleuropneumoniae
It is recognized that biofilms provide various advantages to bacteria including survival in harsh environments and resistance to stresses such as the presence of antibiotics or disinfectants (Jefferson, Reference Jefferson2004; Nadell et al., Reference Nadell, Drescher, Wingreen and Bassler2015; Olsen, Reference Olsen2015; Hathroubi et al., Reference Hathroubi, Mekni, Domenico, Nguyen and Jacques2017). For example, A. pleuropneumoniae grown as a biofilm is less sensitive to antibiotics, and concentrations 100–30 000 times higher than the MIC required to kill planktonic cells (Archambault et al., Reference Archambault, Harel, Gouré, Tremblay and Jacques2012). This decrease in sensitivity has been observed with antibiotics frequently used in pig farms, including ampicillin, florfenicol, tiamulin, and tilmicosin (Archambault et al., Reference Archambault, Harel, Gouré, Tremblay and Jacques2012). It has been suggested that a decrease in sensitivity to antibiotics is due to the sequestration of antibiotics by extracellular matrix components such as PGA, which is found in the biofilm matrix of A. pleuropneumoniae (Nadell et al., Reference Nadell, Drescher, Wingreen and Bassler2015; Olsen, Reference Olsen2015; Hathroubi et al., Reference Hathroubi, Mekni, Domenico, Nguyen and Jacques2017). Indeed, pretreatment of biofilms with dispersin B increases the sensitivity of A. pleuropneumoniae cells to ampicillin suggesting that PGA can limit the diffusion of this antibiotic (Izano et al., Reference Izano, Sadovskaya, Vinogradov, Mulks, Velliyagounder, Ragunath, Kher, Ramasubbu, Jabbouri, Perry and Kaplan2007). In addition to decreasing antibiotic sensitivity, biofilms can also protect against the immune response or decrease the inflammatory response. With A. pleuropneumoniae, pro-inflammatory genes are down-regulated in porcine pulmonary alveolar macrophages exposed to biofilm cells when compared with planktonic cells (Hathroubi et al., Reference Hathroubi, Beaudry, Provost, Martelet, Segura, Gagnon and Jacques2016b). Furthermore, biofilm bacteria reduce the proliferation of porcine peripheral blood mononuclear cells. Interestingly, biofilm cells modify their lipid A structures, and these modifications are absent in planktonic cells. Overall, the immune response towards cells isolated from A. pleuropneumoniae biofilms is weaker and this change could be partially driven by lipid A modification (Hathroubi et al., Reference Hathroubi, Beaudry, Provost, Martelet, Segura, Gagnon and Jacques2016b).
The advantages conferred by biofilm formation might not be limited to stress resistance. During an infection or colonization, biofilms are generally formed as a mixed population of several microorganisms resulting in competitive or mutualistic relationships (Peters, et al., Reference Peters, Jabra-Rizk, O'May, Costerton and Shirtliff2012; Willems et al., Reference Willems, Xu and Peters2016). In some cases, polymicrobial interactions in mixed biofilms can provide fertile ground for the exchange of resistance genes or increased survival and persistence (Harriott and Noverr, Reference Harriott and Noverr2009; De Brucker et al., Reference De Brucker, Tan, Vints, De Cremer, Braem, Verstraeten, Michiels, Vleugels, Cammue and Thevissen2015; Hathroubi et al., Reference Hathroubi, Mekni, Domenico, Nguyen and Jacques2017). Recently, it was demonstrated that A. pleuropneumoniae is able to form mixed biofilms with other swine pathogens such as Streptococcus suis, Bordetella bronchiseptica and P. multocida (Loera-Muro et al., Reference Loera-Muro, Jacques, Avelar-González, Labrie, Tremblay, Oropeza-Navarro and Guerrero-Barrera2016). In this situation, A. pleuropneumoniae does not require the addition of the essential co-factor NAD to the medium for growth and biofilm formation. Furthermore, S. suis, B. bronchiseptica and P. multocida form a weak biofilm that is at near the detection limit of the assay in BHI and in the absence of A. pleuropneumoniae. The association of A. pleuropneumoniae with other swine pathogens appears to benefit both partners. The swine pathogens provide an essential co-factor to A. pleuropneumoniae and, in exchange, A. pleuropneumoniae could provide components for the biofilm structure (e.g., PGA, eDNA, proteins, or lipids) (Loera-Muro et al., Reference Loera-Muro, Jacques, Avelar-González, Labrie, Tremblay, Oropeza-Navarro and Guerrero-Barrera2016).
The benefits of biofilm formation may not be limited to the host environment. Indeed, as an obligate parasite of the porcine respiratory tract, A. pleuropneumoniae can only survive for a very short period of time outside its host and is unable to survive in the farm environment. However, a recent study detected A. pleuropneumoniae in biofilms from the drinking water found on swine farms in Mexico (Loera-Muro et al., Reference Loera-Muro, Jacques, Tremblay, Avelar-González, Loera-Muro, Ramírez, Medina, González and Guerrero-Barrera2013).
A. pleuropneumoniae biofilms may also be advantageous for other microorganisms such as important viral pathogens of pigs. Recently, it was demonstrated that the porcine reproductive and respiratory syndrome virus and type 2 porcine circovirus can persist inside an A. pleuropneumoniae biofilm for several days (Jacques et al., Reference Jacques, Grenier, Labrie, Provost and Gagnon2015).
On a final thought, biofilm may be a contributing factor, to some extent, to the high prevalence of A. pleuropneumoniae in both Canadian domestic pigs (70%) (MacInnes et al., Reference MacInnes, Gottschalk, Lone, Metcalf, Ojha, Rosendal, Watson and Friendship2008) and feral pigs in the USA (69.7%) by favoring persistent infections (Baroch et al., Reference Baroch, Gagnon, Lacouture and Gottschalk2015).
Management of A. pleuropneumoniae outbreaks
A wide variety of antimicrobial agents are used to treat A. pleuropneumoniae: β-lactams (amoxicillin, penicillin, ampicillin, and ceftiofur), tetracyclines (tetracycline and doxycycline), florfenicol, trimethoprim/sulfamethoxazole, tiamulin, lincomycin/spectinomycin, fluoroquinolones (danofloxacin and enrofloxacin), and gentamicin (Dayao et al., Reference Dayao, Gibson, Blackall and Turni2014, Reference Dayao, Gibson, Blackall and Turni2016). In recent years, isolates with different levels of antibiotic resistance have started to arise worldwide (Archambault et al., Reference Archambault, Harel, Gouré, Tremblay and Jacques2012; Dayao et al., Reference Dayao, Gibson, Blackall and Turni2014; Bossé et al., Reference Bossé, Li, Atherton, Walker, Williamson, Rogers, Chaudhuri, Weinert, Holden, Maskell, Tucker, Wren, Rycroft and Langford2015).
The direct link between biofilm formation and levels of antibiotic resistance in A. pleuropneumoniae is still unclear. However, it is worth mentioning that sub-MIC levels of penicillin G may enhance biofilm production via the induction of PGA expression (Hathroubi et al., Reference Hathroubi, Fontaine-Gosselin, Tremblay, Labrie and Jacques2015). Because antibiotics are often used in North America at sub-therapeutic doses for growth promotion and prevention, and A. pleuropneumoniae biofilms are more tolerant to antibiotics (Archambault et al., Reference Archambault, Harel, Gouré, Tremblay and Jacques2012), the judicious use of antibiotics in pig production is highly advised.
Currently, antibiotics represent the most effective measure for controlling A. pleuropneumoniae outbreaks (Gottschalk, Reference Gottschalk2015). The A. pleuropneumoniae biofilm should be taken into consideration for the development of new effective treatment strategies. These strategies should combine antimicrobials with anti-biofilm molecules such as zinc (Wu et al., Reference Wu, Labrie, Tremblay, Haine, Mourez and Jacques2013) or PAβN (Li et al., Reference Li, Cao, Zhang, Lau, Wen, Wu, Zhao, Huang, Yan, Huang and Wen2016b) to overcome persistent infections and reduce the cost of treatment.
Prevention and vaccine strategies against A. pleuropneumoniae
In the last decade, several vaccines have been developed to protect against A. pleuropneumoniae infections. Most of the vaccines are based on recombinant Apx toxins and membrane proteins (such as OMP and type 4 fimbrial proteins) and provide protection against some but not all serotypes (Shao et al., Reference Shao, Wang, Wang, Guo, Peng, Liu, Li, Liu and Liu2010; Lu et al., Reference Lu, Li, Chen, Chu, Lin and Yang2011; Shin et al., Reference Shin, Jung, Lee, Choi, Jang and Yoo2011; Sadilkova et al., Reference Sadilkova, Nepereny, Vrzal, Sebo and Osicka2012; Li et al., Reference Li, Sun, Yang, Yang, Feng, Gu, Han, Langford and Lei2013; Reference Li, Shin, Singh, Maharjan, Park, Kang, Yoo, Hong, Cho and Choi2016c; Hur and Lee, Reference Hur and Lee2014; Yang et al., Reference Yang, Ma, Lei, Huang, Ji, Zhai, Wang, Wang, Li, Sun, Feng and Han2014; Hur et al., Reference Hur, Eo, Park, Choi and Lee2016; Kim et al., Reference Kim, Kim and Yang2016; To et al., Reference To, Nagai, Iwata, Koyama, Oshima and Tsutsumi2016). Inactivated/whole A. pleuropneumoniae cell-based vaccines are also used in many countries to prevent porcine pleuropneumonia (Shao et al., Reference Shao, Wang, Wang, Guo, Peng, Liu, Li, Liu and Liu2010; Lu et al., Reference Lu, Li, Chen, Chu, Lin and Yang2011; Lee et al., Reference Lee, Lee, Chae and Ryu2014; Lopez-Bermudez et al., Reference Lopez-Bermudez, Quintanar-Guerrero, Lara-Puente, Tórtora-Perez, Suárez, Ciprián-Carrasco and Mendoza2014). These vaccines are widely distributed. However, these vaccines do not provide complete protection against all serotypes of A. pleuropneumoniae.
Bacterins are typically prepared from bacteria grown as planktonic cells. Because biofilm cells are known to exhibit phenotypes that are different than their planktonic counterparts (Stewart and Franklin, Reference Stewart and Franklin2008; O'May et al., Reference O'May, Jacobsen, Longwell, Stoodley, Mobley and Shirtliff2009) and A. pleuropneumoniae form biofilm aggregates during an infection (Tremblay et al., Reference Tremblay, Labrie, Chénier and Jacques2017), the vaccines described above may not provide full protection against A. pleuropneumoniae infections. Bacterins may help the vaccinated pig develop a significant memory response against the planktonic form of A. pleuropneumoniae, but the antigenic nature of some targets are modified during growth as biofilms. For example, the A. pleuropneumoniae lipid A molecular structure is modified according to the mode of growth (Hathroubi et al., Reference Hathroubi, Beaudry, Provost, Martelet, Segura, Gagnon and Jacques2016b). Indeed, cells grown as a biofilm have unique lipid A structures that are absent in planktonic cells, including an increase in higher molecular weight lipid A entities (Hathroubi et al., Reference Hathroubi, Beaudry, Provost, Martelet, Segura, Gagnon and Jacques2016b). Accordingly, it would likely be best to create bacterins using both planktonic and biofilm cultures to provide better protection against A. pleuropneumoniae infections by presenting a larger set of antigens that could be biologically relevant.
As with bacterins, commercially available recombinant vaccines based on Apx toxins and/or other proteins have failed to provide a complete protection against every A. pleuropneumoniae isolate (Sjölund and Wallgren, Reference Sjölund and Wallgren2010; Del Pozo-Sacristán et al., Reference Del Pozo-Sacristán, Michiels, Martens, Haesebrouck and Maes2014). The development of new vaccines based on antigens specifically associated with A. pleuropneumoniae biofilms in combination with the Apx toxins and other antigens could help improve the protection but further investigations are required to identify relevant molecules expressed in biofilms and during infection.
Such strategies have been successful in the development of new vaccines against other pathogens. For example, a proteomic analysis of Bordetella pertussis biofilm and planktonic cells identified a biofilm-derived membrane protein called BipA as a potential vaccine antigen (de Gouw et al., Reference de Gouw, Serra, de Jonge, Hermans, Wessels, Zomer, Yantorno, Diavatopoulos and Mooi2014). Vaccination of mice with this antigen showed promising results that included induction of a specific antibody response and a significant reduction in the colonization of lungs by B. pertussis (de Gouw et al., Reference de Gouw, Serra, de Jonge, Hermans, Wessels, Zomer, Yantorno, Diavatopoulos and Mooi2014). Moreover, anti-BipA antibodies have been detected in the serum of convalescent whooping cough patients (de Gouw et al., Reference de Gouw, Serra, de Jonge, Hermans, Wessels, Zomer, Yantorno, Diavatopoulos and Mooi2014). In another example, Gil et al. (Reference Gil, Solano, Burgui, Latasa, García, Toledo-Arana, Lasa and Valle2014) performed an intradermal administration of an exoproteome extract derived from an exopolysaccharide-dependent biofilm to develop an efficient antibiofilm vaccine against Staphylococcus aureus. The biofilm exoproteome induced a humoral immune response and elicited the production of interleukin (IL) 10 and IL-17 in mice. Furthermore, vaccination with the exoproteome extract significantly reduced the number of bacteria within biofilms and surrounding tissue in an in vivo mesh-associated biofilm infection model (Gil et al., Reference Gil, Solano, Burgui, Latasa, García, Toledo-Arana, Lasa and Valle2014).
The strategy of using biofilm-specific antigen is not limited to B. pertussis and S. aureus; others have begun to use similar strategies against bacterial pathogens of importance in veterinary and human health. These pathogens include: S. aureus (Speziale et al., Reference Speziale, Pietrocola, Foster and Geoghegan2014; Gogoi-Tiwari et al., Reference Gogoi-Tiwari, Williams, Waryah, Eto, Tau, Costantino, Tiwari and Mukkur2015), Campylobacter jejuni (Theoret et al., Reference Theoret, Cooper, Zekarias, Roland, Law, Curtiss and Joensa2012), Mycobacterium tuberculosis-complex (Flores-Valdez, Reference Flores-Valdez2016), Streptococcus mutans (Huang et al., Reference Huang, Xu, Liu, Fan and Li2013), Staphylococcus epidermidis (Shahrooei et al., Reference Shahrooei, Hira, Khodaparast, Khodaparast, Stijlemans, Kucharíková, Burghout, Hermans and Elderea2012; Speziale et al., Reference Speziale, Pietrocola, Foster and Geoghegan2014), Bacillus subtilis (Vogt et al., Reference Vogt, Schraner, Aguilar and Eichwald2016), Acinetobacter baumannii (Fattahian et al., Reference Fattahian, Rasooli, Gargari, Rahbar, Astaneh and Amani2011) and Streptococcus equi ssp. zooepidemicus (Yi et al., Reference Yi, Wang, Ma, Lin, Xu, Grenier, Fan and Lu2016) (Table 1).
Table 1. Examples of vaccines based on biofilm-specific antigens produced by pathogenic bacteria of importance in veterinary and human health
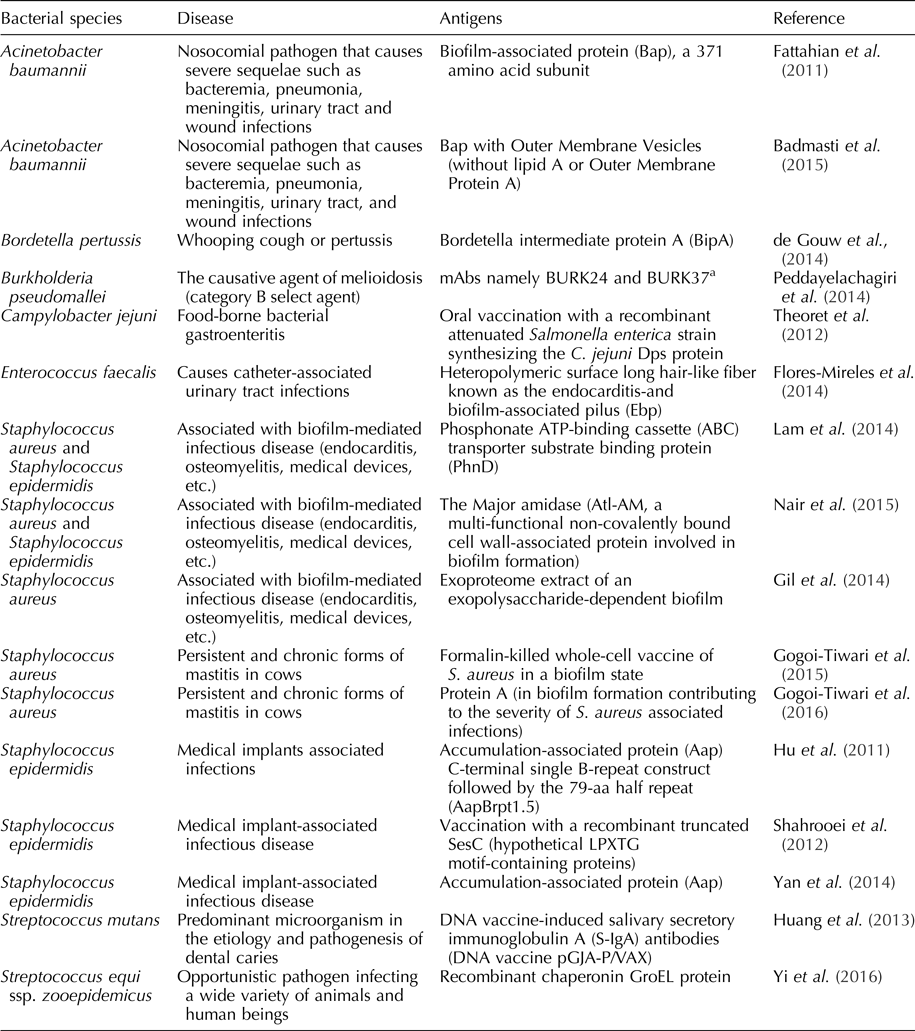
a Murine Monoclonal Antibodies (mAbs) against Burkholderia pseudomallei biofilms.
In the context of biofilm infections, two different types of antigens exist: bacterial cells within the biofilm and the biofilm matrix. The biofilm matrix may be composed of polysaccharides, proteins and extracellular DNA, and the composition of the matrix is dependent on the bacterial genera, species and strains (Harro et al., Reference Harro, Peters, O'May, Archer, Kerns, Prabhakara and Shirtliff2010). Different studies have focused on identifying antigens from the bacteria, the matrix or both as the best strategy for the development of effective vaccines (Table 1).
Another factor that must be considered is that biofilm consortia typically exist as communities of bacteria, viruses, protozoans and fungi, and the overall biofilm architecture is affected by specific intermicrobial and host interactions (Harro et al., Reference Harro, Peters, O'May, Archer, Kerns, Prabhakara and Shirtliff2010). These consortia can allow colonization and subsequent infection by opportunistic pathogens that exploit unique niches found in these polymicrobial environments, resulting in the development of polymicrobial infections.
Finally, vaccine research and design should take advantage of the new techniques such as RNA sequencing, bioinformatics, proteomics and lipidomics to identify molecules specifically expressed or secreted during biofilm formation. In our opinion, this should greatly improve the efficacy of future vaccines and ensure better protection of pigs against A. pleuropneumoniae.
Conclusion and future challenges
Despite different strategies and years of prevention and control, A. pleuropneumoniae remains one of the main respiratory pathogens of pigs and is responsible for great economic loses to the worldwide pork industry. Although some countries, such as the USA and Canada, can manage A. pleuropneumoniae, this pathogen remains present in farms and, thus, a resurgence in new outbreaks is always possible. Such new outbreaks could emerge from isolates with increased resistance to antibiotics. Great efforts have been made to prevent infections with this pathogen through optimal farm management and through major investments in research and development of new and better vaccines. However, neither management nor vaccines have been 100% effective at controlling A. pleuropneumoniae infections. Fortunately, new research is shedding light on the pathogenesis of A. pleuropneumoniae, which is improving our understanding of this old acquaintance. Importantly, recent studies have revealed that A. pleuropneumoniae forms biofilm aggregates in the lung (Tremblay et al., Reference Tremblay, Labrie, Chénier and Jacques2017) and can form multi-species biofilms with other respiratory pathogens (Loera-Muro et al., Reference Loera-Muro, Jacques, Avelar-González, Labrie, Tremblay, Oropeza-Navarro and Guerrero-Barrera2016). Using these new findings, it will be possible to identify novel vaccine candidates to improve the next generation of vaccines and to develop better strategies to control A. pleuropneumoniae. These new developments could hopefully help prevent the persistent problems caused by this pathogen in the worldwide production of pigs for the last 50 years.
Acknowledgments
This review was supported by a Discovery Grant (RGPIN-2016-04203 to MJ) from the Natural Sciences and Engineering Research Council of Canada (NSERC) and by CONACYT, Mexico (Cátedras CONACYT Program to ALM).