1. Introduction
The eastern margin of the Bohemian Massif represents the NE extremity of the Variscan belt in Europe where it borders the Brunovistulian (Dudek, Reference Dudek1980) or Brunia microplate (Zapletal, Reference Zapletal1932; Kröner et al. Reference Kröner, Štípská, Schulmann, Jaeckel, Franke, Haak, Oncken and Tanner2000), placed between the Variscan domain and the SW Baltica margin (Fig. 1; e.g. Pharaoh, Reference Pharaoh1999; Belka et al. Reference Belka, Ahrendt, Franke, Wemmer, Franke, Haak, Oncken and Tanner2000). The tectonostratigraphic record of Brunia is clearly separate from that revealed by other terranes exposed in the Bohemian Massif (e.g. Kalvoda et al. Reference Kalvoda, Melichar, Bábek and Leichmann2002). Therefore, Brunia is interpreted as a continental terrane separated during most of the Palaeozoic from terranes assembled in the Variscan belt (Pharaoh, Reference Pharaoh1999; Winchester et al. Reference Winchester2002). Based on macrofaunal affinities and palaeomagnetic data, Brunia was interpreted as a fragment of Gondwana-derived crust which crossed the Tornquist Sea and approached Baltica before the end of Cambrian times (e.g. Belka et al. Reference Belka, Ahrendt, Franke, Wemmer, Franke, Haak, Oncken and Tanner2000; Winchester et al. Reference Winchester2002; Nawrocki et al. Reference Nawrocki, Żylińska, Buła, Grabowski, Krzywiec and Poprawa2004; Żelaźniewicz et al. Reference Żelaźniewicz, Buła, Fanning, Seghedi and Żaba2009). Alternatively, Brunia may have already been attached to Baltica since Neoproterozic times (e.g. Pharaoh, Reference Pharaoh1999; Nawrocki et al. Reference Nawrocki, Żylińska, Buła, Grabowski, Krzywiec and Poprawa2004). On the other hand, micropalaeontology and geochronological data led to the conclusion that Brunia exhibits certain affinities to East Avalonia (Moczydłowska, Reference Moczydłowska1997; Friedl et al. Reference Friedl, Finger, McNaughton and Fletcher2000; Finger et al. Reference Finger, Hanžl, Pin, Quadt, Steyrer, Franke, Haak, Oncken and Tanner2000; Murphy et al. Reference Murphy, Pisarevsky, Nance and Keppie2004; Nance et al. Reference Nance, Murphy, Strachan, Keppie, Gutierrez-Alonso, Fernandez-Suarez, Quesada, Linnemann, D'Lemos, Pisarevsky, Nasser and Liégeois2008). These contrasting interpretations postulate derivation of Brunia from either Baltica or Gondwana and, in the latter case, a different time for its drift away from the northern Gondwana margin.

Figure 1. Location of Brunia at the eastern periphery of the Variscan belt. KLF – Kraków-Lubliniec Fault; MGCH – Mid-German Crystalline High; MO – Moldanubian; MS – Moravo-Silesian; RH – Rhenohercynian; ST – Saxothuringian; SU – Sudetes; TB – Tepla-Barrandian; TTZ – Teisseyre-Tornquist Zone; VF – Variscan front.
We use ion-microprobe and Pb/Pb evaporation zircon ages, geochemical characteristics and structural data to date and assess the affinity of various granitoid orthogneiss bodies scattered across part of the tectonic boundary dividing the Brunian microcontinent in the east from the Bohemian Massif in the west. The aim of our study is to check whether the distribution of different types of gneiss mirrors the tectonic contact between these two crustal domains. Using this approach, we attempt to verify the contrasting provenance and evolution of the Brunian microcontinent relative to components of the Variscan belt.
2. Geological setting and previous studies
The western margin of the Brunian terrane comprises Neoproterozoic ortho- and paragneisses, accompanied by minor metapelites which are unconformably overlain by Devonian sediments and volcanic rocks (e.g. Dudek, Reference Dudek1980; Cháb et al. Reference Cháb, Mixa, Vanecek and Žáček1994). These rocks are part of the Moravo-Silesian metamorphic nappe complex which straddles the eastern margin of the Bohemian Massif (Suess, Reference Suess1912; Matte et al. Reference Matte, Maluski, Rajlich and Franke1990; Schulmann et al. Reference Schulmann, Ledru, Autran, Melka, Lardeaux, Urban and Lobkowicz1991; Fritz & Neubauer, Reference Fritz and Neubauer1993; Schulmann & Gayer, Reference Schulmann and Gayer2000). These nappes were emplaced during the Early Carboniferous collision of Brunia with Bohemia which followed westward subduction of the Brunian passive margin below the eastern margin of the Bohemian Massif. In its northern segment, the collision zone is represented by the East Sudetic nappes, overridden from the west by the Orlica–Śnieżnik Massif and the Staré Město belt (Fig. 2) and exposed in a tectonic half-window. The whole area in question is characterized by a NNE–SSW structural trend usually attributed to the effects of final Variscan collision (e.g. Schulmann & Gayer, Reference Schulmann and Gayer2000).
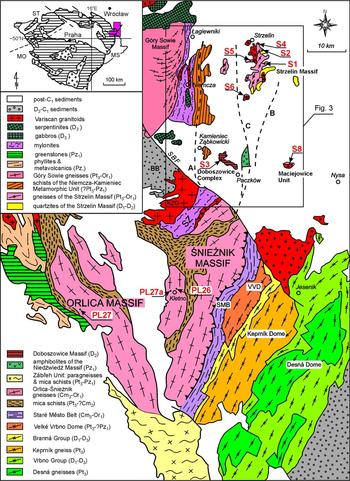
Figure 2. Geological sketch map of the boundary zone between the East and Central Sudetes showing location of analysed samples. BB – Bardo Basin; KZG – Kłodzko-Złoty Stok Granite; SBF – Sudetic Boundary Fault; SMB – Staré Město belt; VVD – Velké Vrbno Dome. Position of the tectonic contact between the Central and East Sudetes in the Fore-Sudetic Block according to: (A) Bederke (Reference Bederke1929); (B) Oberc (Reference Oberc1968); (C) Skácel (Reference Skácel1989).
The Orlica–Śnieżnik Massif is largely composed of amphibolite-grade granitoid orthogneisses and subordinate staurolite-grade variegated schists of presumably Neoproterozoic to Cambrian age, and it contains eclogite and granulite inclusions (e.g. Don et al. Reference Don, Dumicz, Wojciechowska and Żelaźniewicz1990). The emplacement age of the orthogneiss precursors was dated at about 490–520 Ma, using U–Pb and Pb–Pb zircon dating methods (Oliver, Corfu & Krogh, Reference Oliver, Corfu and Krogh1993; Turniak, Mazur & Wysoczanski, Reference Turniak, Mazur and Wysoczanski2000; Kröner et al. Reference Kröner, Štípská, Schulmann, Jaeckel, Franke, Haak, Oncken and Tanner2000, Reference Kröner, Jaeckel, Hegner and Opletal2001; Štípská, Schulmann & Kröner, Reference Štípská, Schulmann and Kröner2004). The hitherto published geochronological studies (Turniak, Mazur & Wysoczanski, Reference Turniak, Mazur and Wysoczanski2000; Lange et al. Reference Lange, Bröcker, Armstrong, Żelaźniewicz, Trapp and Mezger2005; Żelaźniewicz et al. Reference Żelaźniewicz, Nowak, Larionov and Presnyakov2006; Bröcker et al. Reference Bröcker, Klemd, Cosca, Brock, Larionov and Rodionov2009) reveal the occurrence of two populations of inherited zircon cores in the orthogneisses: (1) early Cambrian to late Neoproterozoic (c. 520–620 Ma), and (2) Palaeoproterozoic to late Archaean (c. 1780–2800 Ma). Such an inherited zircon age spectrum appears to be typical of the Armorican crust with North African affinities that is widespread throughout the Bohemian Massif (Friedl et al. Reference Friedl, Finger, McNaughton and Fletcher2000).
The Staré Město belt represents a suture zone between the Central and East Sudetes (Parry et al. Reference Parry, Štípská, Schulmann, Hrouda, Ježek and Kröner1997; Kröner et al. Reference Kröner, Štípská, Schulmann, Jaeckel, Franke, Haak, Oncken and Tanner2000; Štipská et al. Reference Štípská, Schulmann, Thompson, Ježek and Kröner2001) and also yielded protolith ages in the range of 500–505 Ma (Kröner et al. Reference Kröner, Štípská, Schulmann, Jaeckel, Franke, Haak, Oncken and Tanner2000). The emplacement of a bimodal volcanic suite, tonalite–trondhjemite granitoids and layered gabbros was associated, within the Staré Město belt, with HT/LP metamorphism, anatectic melting and extensional deformation. These phenomena were attributed by Kröner et al. (Reference Kröner, Štípská, Schulmann, Jaeckel, Franke, Haak, Oncken and Tanner2000) to an important rifting and underplating event at c. 500 Ma.
The boundary between the Orlica–Śnieżnik Massif and the East Sudetic nappe complex continues towards the north and northeast into the Fore-Sudetic Block, where their contact is not well-defined due to poor exposure of the crystalline basement (Fig. 2). Therefore, the location of this suture has been traced largely through geochronological evidence (Oberc-Dziedzic et al. Reference Oberc-Dziedzic, Klimas, Kryza and Fanning2003, Reference Oberc-Dziedzic, Kryza, Klimas, Fanning and Madej2005). This evidence is based on the widespread occurrence of latest Cambrian to early Ordovician orthogneisses in the Central and West Sudetes (e.g. Oliver, Corfu & Krogh, Reference Oliver, Corfu and Krogh1993; Kröner & Hegner, Reference Kröner and Hegner1998; Kröner et al. Reference Kröner, Jaeckel, Hegner and Opletal2001) and their scarcity in the East Sudetes. The subdivision into the West and Central Sudetes on one side and the East Sudetes on the other reflects the distinction between Armorica-derived crustal fragments (e.g. Aleksandrowski & Mazur, Reference Aleksandrowski, Mazur, Winchester, Pharaoh and Verniers2002) and the deformed margin of the Brunian terrane (Schulmann & Gayer, Reference Schulmann and Gayer2000), respectively. The results of U–Pb single zircon dating were used by Oberc-Dziedzic et al. (Reference Oberc-Dziedzic, Klimas, Kryza and Fanning2003, Reference Oberc-Dziedzic, Kryza, Klimas, Fanning and Madej2005) to delimit the westward extent of the Neoproterozoic basement in the eastern Fore-Sudetic Block. These authors dated zircons from two gneiss samples of the Strzelin Massif (Fig. 3), which yielded SHRIMP ages of 600–568 Ma and 500 Ma, respectively. The latter age is in agreement with the earlier result of Oliver, Corfu & Krogh (Reference Oliver, Corfu and Krogh1993), who obtained and age of 504±3 Ma by using a multigrain TIMS method for another textural variety of gneiss exposed in the same area (Fig. 3). The close coexistence of Neoproterozoic and early Palaeozoic gneisses was interpreted by Oberc-Dziedzic & Madej (Reference Oberc-Dziedzic and Madej2002) and Oberc-Dziedzic et al. (Reference Oberc-Dziedzic, Pin and Kryza2003, Reference Oberc-Dziedzic, Kryza, Klimas, Fanning and Madej2005) in terms of a sharp tectonic contact and was seen as the location of the Strzelin Thrust. This thrust is considered equivalent to the tectonic contact between the Central and East Sudetes and, on a larger scale, to the western limit of Brunia-derived rock complexes.

Figure 3. Geological sketch map of the eastern Fore-Sudetic Block that contains the contact between rocks derived from the Brunian microplate and the Central Sudetes. Location of cross-section of Figure 5 is indicated by line A–B.
3. Sample locations and description
We sampled major gneiss occurrences in a zone about 20 km wide which, according to the regional context (Fig. 2) and all previous studies (e.g. Bederke, Reference Bederke1929; Oberc, Reference Oberc1968; Skácel, Reference Skácel1989; Oberc-Dziedzic et al. Reference Oberc-Dziedzic, Klimas, Kryza and Fanning2003), should contain the contact between rock units derived from the Brunian basement and the Central Sudetes (Fig. 3). Four samples, dated by ion-microprobe, were collected from the Strzelin Massif, Doboszowice Massif and the metamorphic envelope of the Maciejowice granitoid (Figs 3, 4). Three more samples come from the Orlica–Śnieżnik Massif (Fig. 2) and represent the Śnieżnik and Gierałtów gneisses, two main textural varieties distinguished in that area. These samples have been analysed using the 207Pb/206Pb single zircon evaporation method. GPS coordinates of the sample locations are reported in Tables 1, A1 and A2. Photos of the dated samples (Figs A1, A2) are available in the online Appendix at http://journals.cambridge.org/geo.

Figure 4. Geological sketch maps of selected parts of the eastern Fore-Sudetic Block showing location of dated samples (see Figs 2 and 3 for regional setting). Stereonets in maps (a) and (b) show orientation of foliation (density contours) and lineation (black dots) in sampled outcrops. In both stereonets (Schmidt net, lower hemisphere) 0.5, 5 and 15% contours per 1% area. Structural data for outcrops (c) and (d) are presented in Figure 5.
Table 1. Chemical and normative data for granite gneisses from the eastern Fore-Sudetic Block

Sample locations: S1 = N50°43′46.3″, E17°07′29.5″; S2 = N50°45′32.5″, E17°06′30.2″; S3 = N50°30′32.3″, E16°56′42.1″; S4 = N50°46′26.7″, E17°05′21.0″; S5 = N50°31′31.1″, E17°06′10.6″; S6A = N50°39′10.4″, E17°03′00.4″; S6B = N50°39′06.5″, E17°03′05.7″; S7 = N50°31′31.1″, E17°06′10.6″; S8A, B = N50°30′00.4″, E17°09′25.7″; S9 = N50°30′32.3″, E16°56′42.1″
GPS coordinates are given in WGS 84. Detection limits for major elements are: 0.04% for SiO2, Fe2O3, K2O, 0.03% for Al2O3; 0.01% for CaO, MgO, Na2O, MnO, TiO2, P2O5; 0.001 for Cr2O3. Detection limits for trace elements are: 5 ppm for V, 0.5 ppm for Ba, Ga, Hf, Nb, Rb, Sr, Zr, La, Ce; 0.1 ppm for Cs, Ni, Pb, Ta, Th, U, Y, Sm; 0.01 ppm for Tb, Lu; 0.02 ppm for Pr; 0.05 ppm for Eu, Gd, Dy, Ho, Er, Tm, Yb.
Two of our samples represent the Strzelin Massif, a crystalline unit which emerges at the surface about 40 km south of Wrocław (Fig. 3). The massif mainly consists of gneisses, interlayered with minor mica schists and amphibolites, with the latter showing within-plate geochemical signatures (Szczepański & Oberc-Dziedzic, Reference Szczepański and Oberc-Dziedzic1998). These rocks are tectonically interleaved with quartzites (Oberc, Reference Oberc1966) similar to those known from the East Sudetes, and are therefore assumed to be of early to middle Devonian age (e.g. Bederke, Reference Bederke1935). The orthogneisses comprise several textural types including (1) a fine- to medium-grained porphyric variety (Strzelin gneiss), (2) a coarse-grained augen variety (Gościęcice gneiss) and (3) a migmatitic, sillimanite-bearing variety (Nowolesie gneiss).
The samples selected for zircon dating from the Strzelin Massif represent a coarse-grained augen gneiss variety (sample S4, Gościęcice gneiss) and a migmatitic, sillimanite-bearing gneiss (sample S6, Nowolesie gneiss). The former is similar to material dated by Oliver, Corfu & Krogh (Reference Oliver, Corfu and Krogh1993) at 504±3 Ma, whereas the latter resembles an anatectic derivative from the Strzelin gneiss. We dated zircons from sample S4 to confirm the occurrence of early Palaeozoic gneisses in the Strzelin Massif (Oliver, Corfu & Krogh, Reference Oliver, Corfu and Krogh1993), whereas the analysis of sample S6 was aimed at better constraining the possible extent and age of the Proterozoic orthogneiss suite.
The Gościęcice augen gneiss (Fig. 4a) is a coarse, weakly foliated rock with K-feldspar megacrysts. This major gneiss variety is locally transformed into a medium- to fine-grained laminated augen gneiss and/or fine-grained banded gneiss, produced by localized ductile strain operating under amphibolite-facies conditions. Although contacts of the Gościęcice gneiss with other rocks are entirely obscured beneath Cenozoic sediments, borehole data reveal mylonitization of the gneiss near its boundary with adjacent amphibolites (Oberc-Dziedzic & Madej, Reference Oberc-Dziedzic and Madej2002). The dated sample (S4) represents a foliated, but not layered, coarse-grained variety (Fig. A1a) composed of K-feldspar, oligoclase, quartz and biotite with minor muscovite and chlorite rarely replacing biotite. Zircon, apatite, sphene and opaque minerals are accessory phases.
The migmatitic Nowolesie gneiss (sample S6) is a fine-grained and locally porphyric rock composed of quartz, K-feldspar, plagioclase, biotite, garnet and sillimanite. Zircon (both euhedral and anhedral grains), apatite and opaque minerals are common accessory phases. Sillimanite needles are usually concentrated in small, up to 2 cm long, oval nodules, which are a characteristic feature of the rock. The Nowolesie gneiss records intense anatexis revealed by volumetrically significant melt patches and leucocratic leucosome layers unevenly dispersed across the sampled exposure at Skalice (Fig. 4b). The development of anatectic leucosomes was accompanied by heterogeneous deformation of the pre-metamorphic fabric which is manifested by fragmentation of dark patches with gneissic texture, surrounded by a slush of melt. Our sample selected for zircon dating (S6) was collected at Skalice crag (Fig. 4b). It is a well-layered granitoid gneiss in which large sillimanite needles grow across the main foliation and which is cut by unfoliated pegmatites. The sample represents a homogeneous porphyric textural variety of the Nowolesie gneiss (Fig. A1b) with both K-feldspar and plagioclase porphyroclasts which are apparently unaffected by widespread anatexis.
The Doboszowice Massif represents an isolated outcrop of crystalline basement emerging from beneath a thick Cenozoic cover to the north of the Orlica–Śnieżnik Massif (Figs 2, 3). It consists of orthogneisses showing an intrusive contact in the east against a varied succession of paragneisses, amphibolites and mica schists (Mazur, Puziewicz & Józefiak, Reference Mazur, Puziewicz and Józefiak1995). The Doboszowice gneiss is a leucocratic two-mica metagranite composed of quartz, K-feldspar and plagioclase, accompanied by minor muscovite and biotite and, locally, by garnet, albite and chlorite. Accessories are represented by apatite, zircon and allanite. Textural and petrographic features point to synkinematic emplacement of this orthogneiss under lower amphibolite-facies conditions and subsequent cooling during waning deformation (Mazur, Puziewicz & Józefiak, Reference Mazur, Puziewicz and Józefiak1995; Puziewicz & Rudolf, Reference Puziewicz and Rudolf1998). Diagnostic features are numerous in situ melt patches transecting the foliation and/or penetrating into ductile lock-up shear zones which indicate local anatectic mobilization of the granite material during high-temperature deformation. Synkinematic intrusion and cooling of the granitic precursor gave rise to a wide range of intimately associated textural varieties characteristic of the orthogneiss in the Doboszowice quarry (Fig. 4c). Massive rocks with granitic texture are commonly accompanied in this exposure by widespread augen and banded gneisses as well as ‘pencil gneisses’. The sampled variety of the orthogneiss (S3) represents a well-foliated medium-grained metagranite of homogeneous texture and average mineral composition (Fig. A1c).
The outcrop of the Maciejowice unit (Fig. 4d) is situated several kilometres east of the Strzelin Massif and Staré Město belt, in an area that is apparently part of the East Sudetes (Figs 2, 3). The Maciejowice granite intrudes a variegated assemblage of metamorphic rocks dominated by a leucocratic orthogneiss. The latter consists of quartz, K-feldspar and plagioclase, accompanied by minor biotite and, locally, by garnet and chlorite. Accessories are represented by apatite, zircon and rutile. A minor mesocratic variety contains abundant biotite. The textural variability of the orthogneiss from augen to banded and/or streaky varieties typically reflects a highly variable intensity of deformation. The orthogneiss is closely associated with paragneisses comprising variable quartz and plagioclase, accompanied by large amounts of biotite and muscovite, abundant garnet and minor kyanite, sillimanite and chloritoid. The sampled variety of the Maciejowice orthogneiss (S8) represents a coarse-grained leucocratic augen gneiss of homogeneous texture and typical mineral composition (Fig. A1d).
The granitic protoliths of the Orlica–Śnieżnik gneisses were transformed by deformation and amphibolite-facies metamorphism into two main textural varieties known as the Śnieżnik and Gierałtów gneisses (Fischer, Reference Fischer1936). Turniak, Mazur & Wysoczanski (Reference Turniak, Mazur and Wysoczanski2000) and Lange et al. (Reference Lange, Bröcker, Armstrong, Żelaźniewicz, Trapp and Mezger2005) had shown that the Śnieżnik and Gierałtów gneisses have virtually identical geochemistry and were therefore derived from identical or similar protoliths, and our field observations suggest that the Gierałtów gneiss is a strongly deformed and variably mylonitized textural variety of the Śnieżnik gneiss. We dated two samples of the Śnieżnik gneiss (PL26, PL27) and one sample of the Gierałtów gneiss (PL27a) to verify the similarity of their protoliths and provide reference ages for gneisses from the contact zone between the Central Sudetes and Brunia. The Śnieżnik gneiss is a coarse- to medium-grained rock with K-feldspar augen. It is composed of quartz, microcline, oligoclase, muscovite, biotite and accessory apatite, zircon, rutile, sphene, garnet and opaque minerals. Local transitions between homogeneous and well-layered textural varieties record variable strain intensities. In contrast, the Gierałtów gneiss is a fine-grained, approximately equigranular, often thinly laminated, two-mica rock of granitic composition similar to that of the Śnieżnik gneiss. Locally it displays a migmatitic texture, considerably different from the augen texture of the Śnieżnik gneiss. Several transitional textural varieties also occur between the two gneiss types.
Sample PL26 is a typical Śnieżnik augen-gneiss (Fig. A2a), as described by Don et al. (Reference Don, Dumicz, Wojciechowska and Żelaźniewicz1990) and Turniak, Mazur & Wysoczanski (Reference Turniak, Mazur and Wysoczanski2000), and was collected NE of Kletno (Fig. 2). Another variety of granite-gneiss was collected in the Zdobnice Valley, close to the Polish/Czech border (Fig. 2; sample PL27). This is a pink, coarse-grained rock (Fig. A2b) very similar in appearance to Śnieżnik-type gneisses on the Polish side of the Orlica–Śnieżnik Massif (Kröner et al. Reference Kröner, Jaeckel, Hegner and Opletal2001). Sample PL27a, collected NE of Jaworek (Fig. 2), is a light grey, fine-grained and strongly foliated Gierałtów granite gneiss displaying a thinly laminated flaser texture due to mylonitization (Fig. A2c).
4. Structural data
The cross-section in Figure 5 illustrates details of a contact zone between rock complexes derived from the Central Sudetes in the west and the Brunian microplate in the east. The area, except for the easternmost Maciejowice Unit, displays fairly uniform orientation of foliation, which dips to the west at a median angle of 40–45° and bears a mineral stretching lineation plunging to the SW (Fig. 5). Foliation parallels tectonic contacts while approaching the high strain zones. The presumed tectonic suture between the Central Sudetes and the Brunian microplate corresponds to a thick amphibolite body (Fig. 2; Niedźwiedź Massif; Skácel, Reference Skácel1989) that is additionally constrained by two deep boreholes (Cymerman & Jerzmański, Reference Cymerman and Jerzmański1987; Fig. 5). The moderate dip of this suture is analogous to that reported from the Staré Mĕsto belt in the mountainous part of the East Sudetes (Schulmann & Gayer, Reference Schulmann and Gayer2000). The present-day geometry of foliation along the cross-section (Fig. 5) seems to preclude the existence of low-angle thrusts and related gneissic outliers rooted in the Central Sudetes and preserved as tectonic caps. The situation might be more complex further north in the Strzelin Massif where the occurrence of the Strzelin Thrust has been reported (Oberc-Dziedzic & Madej, Reference Oberc-Dziedzic and Madej2002; Oberc-Dziedzic et al. Reference Oberc-Dziedzic, Klimas, Kryza and Fanning2003). The thrust is thought to separate the Gościęcice orthogneiss that crops out among the Strzelin gneisses (Figs 3, 4a).

Figure 5. Schematic E–W section across the eastern Fore-Sudetic Block (for location see Fig. 3) to show the structure of a contact zone between rock units derived from the Brunian microplate and the Central Sudetes. Foliation and lineation data for the Kamieniec Unit, Doboszowice Massif, Niedźwiedź Massif and Maciejowice Unit are presented directly above and below the cross-section. All stereonets (Schmidt net, lower hemisphere) display 0.5, 5 and 15% contours per 1% area. For further details see Mazur & Józefiak (Reference Mazur and Józefiak1999).
The Gościęcice gneiss shows N-dipping foliation and NNW- to N- and NE-plunging stretching lineation, the effects of top-to-the-N–NNE shearing (Oberc-Dziedzic et al. Reference Oberc-Dziedzic, Kryza, Klimas, Fanning and Madej2005). This pervasive fabric was interpreted by Oberc-Dziedzic et al. (Reference Oberc-Dziedzic, Kryza, Klimas, Fanning and Madej2005) as a product of ductile north–northeastward thrusting. However, the orientation of foliation and lineation in the outcrop of Gościęcice gneiss (Fig. 4a), combined with the occurrence of top-to-the-north kinematic indicators, suggests a low-angle dip-slip normal shear zone rather than a ductile thrust (Strzelin Thrust) as postulated by Oberc-Dziedzic et al. (Reference Oberc-Dziedzic, Kryza, Klimas, Fanning and Madej2005). The former possibility is additionally supported by a contrastingly higher metamorphic grade of amphibolites occurring in the footwall of the assumed thrust (Oberc-Dziedzic & Madej, Reference Oberc-Dziedzic and Madej2002). Therefore, the Strzelin Thrust might have been subsequently reactivated and undergone a complex structural evolution. It is also not totally ruled out that the Strzelin Thrust utilized a former intrusive contact between the magmatic precursor to the Gościęcice gneiss and its country rocks. This may be suggested by the present-day outcrop pattern (Fig. 4a) and the relatively local scale of the shear zone described at the contact of the Gościęcice gneiss (Oberc-Dziedzic & Madej, Reference Oberc-Dziedzic and Madej2002).
5. Analytical methods
Chemical analyses were performed to verify the genetic variation amongst the various gneiss types of the eastern Fore-Sudetic Block (Fig. 3). Eleven samples were collected from different gneiss varieties and analysed for major and trace elements using combined ICP-ES and ICP-MS techniques (Table 1). Six samples come from the Strzelin Massif (S1, S2, S4, S5, S6A, S6B), two from the Doboszowice Massif (S3, S9), one from the Lipniki metagranite (S7) and two from the Maciejowice orthogneiss representing its light and dark varieties (S8A and S8B, respectively). Major element concentrations were determined by ICP-ES following lithium metaborate fusion and nitric acid digestion of a 0.2 g sample. Loss on ignition (LOI) was determined after ignition at 1000°C. Trace elements were analysed by ICP-MS following a decomposition technique similar to that for major elements. Accuracy is within 0.01% for most major elements with the exception of SiO2, Al2O3, FeOt and K2O, for which it was 0.04%. For most trace elements, including LREE, the detection limit was 0.5 ppm, whereas for MREE and HREE it was 0.05 ppm. The analyses were supplied by ACME Analytical Laboratories Ltd, Canada.
The U–Pb analyses of the zircons were made using a SHRIMP-II ion microprobe (Centre of Isotopic Research, VSEGEI, St Petersburg, Russia). Zircon grains were hand-selected and mounted in epoxy resin together with chips of the TEMORA (Middledale Gabbroic Diorite, New South Wales, Australia; Black et al. Reference Black, Kamo, Allen, Aleinikoff, Davis, Korsch and Foudoulis2003) and 91500 (Geostandard zircon; Wiedenbeck et al. Reference Wiedenbeck, Allé, Corfu, Griffin, Meier, Oberli, von Quadt, Roddick and Spiegel1995) reference zircons. The grains were sectioned approximately in half and polished. Reflected and transmitted light photomicrographs and cathodoluminescence (CL) SEM images were prepared for all zircons. The CL images were used to decipher the internal structures of the sectioned grains and to target specific areas for analysis within these zircons. Each analysis consisted of five scans through the mass range, the spot diameter was about 25 μm, and primary beam intensity was about 4 nA. The data have been reduced following Williams (Reference Williams, McKibben, Shanks III and Ridley1998 and references therein), using the SQUID Excel Macro of Ludwig (Reference Ludwig2000). Pb/U ratios were normalized relative to a value of 0.0668 for the 206Pb/238U ratio of the TEMORA reference zircons, equivalent to an age of 416.75 Ma (Black et al. Reference Black, Kamo, Allen, Aleinikoff, Davis, Korsch and Foudoulis2003). Common Pb was corrected using measured 204Pb and the relevant Pb composition of Stacey & Kramers (Reference Stacey and Kramers1975). Uncertainties given for individual analyses (ratios and ages) are at the 1σ level; however, uncertainties in calculated concordia ages are reported at the 2σ level. Ahrens-Wetherill concordia plots (Wetherill, Reference Wetherill1956) were prepared using ISOPLOT/EX (Ludwig, Reference Ludwig1999).
The Pb–Pb analyses of the zircons were performed using the zircon evaporation dating method developed by Kober (Reference Kober1987). Our laboratory procedures, as well as comparisons with conventional and ion-microprobe zircon dating, are detailed in Kröner, Byerly & Lowe (Reference Kröner, Byerly and Lowe1991) and Kröner & Hegner (Reference Kröner and Hegner1998). Isotopic measurements were carried out on a Finnigan-MAT 261 mass spectrometer at the Max-Planck-Institut für Chemie in Mainz. The calculated ages and uncertainties are based on the means of all ratios evaluated and their 2σ mean errors. Mean ages and errors for several zircons from the same sample are presented as weighted means of the entire population. During the course of this study we have repeatedly analysed fragments of large zircon grains from the Palaborwa Carbonatite, South Africa. These magmatic zircons, used as an internal standard, are completely homogeneous when examined under cathodoluminescence. Conventional U–Pb analyses of six separate grain fragments from this sample yielded a 207Pb/206Pb age of 2052.2±0.8 Ma (2σ) (W. Todt, unpub. data), whereas the mean 207Pb/206Pb ratio for 18 grains, evaporated individually over a period of 12 months, is 0.126634±0.000026 (2σ error for the population), corresponding to an age of 2051.8±0.4 Ma, identical to the U–Pb age. The above error is considered the best estimate for the reproducibility of our evaporation data and approximately corresponds to the 2σ (mean) error reported for most individual analyses in this study. In the case of pooled analyses the 2σ (mean) error may become very low, and whenever this error was less than the reproducibility of the internal standard we have used the latter value, that is, an assumed 2σ error of 0.000026.
6. Geochemistry
Based on systematic differences in trace element patterns, in particular due to light to heavy REE fractionation, the analysed gneisses are subdivided into two types: (G1) Strzelin and Nowolesie gneisses (S1, S2, S5, S6A, S6B) and (G2) Gościęcice, Lipniki, Doboszowice and Maciejowice gneisses (S3, S7, S8A, S8B, S9). Chondrite-normalized multi-element patterns (Fig. 6) revealed by samples of type G1 are characterized by high LREE/HREE ratios ((La/Lu)N = 12.8–27.8) which are typical of granites formed in destructive margin settings. A pronounced negative P-anomaly as revealed on primitive mantle-normalized trace element plots indicates apatite fractionation. Type G2 is moderately LREE enriched ((La/Lu)N = 3.9–12.2) and shows negative Eu- (±Sr) and Ti-anomalies, characteristic of evolved magmas and indicative of probable plagioclase and Ti-rich phase fractionation and removal. On the other hand, a negative Sr anomaly may reflect mobility of these elements during metamorphism. All samples also display distinct negative Ta- and Nb-anomalies which suggest a likely contribution of lower crustal material. Input of upper crustal material is apparent only in type G2, as demonstrated by positive Th- and/or U-anomalies. Classification and discrimination diagrams for the studied rocks (Figs A3, A4, A5) are available in the online Appendix at http://journals.cambridge.org/geo.

Figure 6. (a) Chondrite-normalized REE patterns for gneisses from the eastern Fore-Sudetic Block (chondritic values from Nakamura, Reference Nakamura1974). (b) Primitive mantle-normalized trace element variation diagram (primitive mantle values from Sun & McDonough, Reference Sun, McDonough, Saunders and Norry1989).
The analysed gneisses are mostly of granitic composition as confirmed by normative mineral proportions and trace element concentrations (Fig. A3a, b). Interestingly, gneisses of type G1 display relatively high Nb/Y ratios and, on the Nb/Y–Zr/TiO2 variation diagram, partly plot in the monzonite field (Fig. A3b). On the Ab–An–Or diagram, the same samples, comparatively enriched in albite, are dispersed between the granite and trondhjemite fields (Fig. A3a). This may be the result of K- and Na-mobility during metamorphism, as well as a consequence of the original composition. The peraluminous character of all orthogneisses is indicated by the molar proportions A/CNK and A/NK ranging between 1.12–1.33 and 1.17–1.68, respectively. However, the value of A/CNK and A/NK parameters is limited due to Ca and alkali mobility. On the MgO–Al2O3 diagram (not shown here), the analysed samples project well into the orthogneiss field. Based on CIPW normative corundum values and the A/CNK ratio, it is likely that their magmatic precursors were produced by melting of sedimentary rocks.
Gneisses of type G1 display two distinct features on a ORG (ocean-ridge granites)-normalized diagram (not shown here): (a) a sloping pattern for HFS elements (from Ce to Yb) below the ORG mean value, and (b) distinctly higher values for LIL elements and thorium as compared to ORG. These features resemble volcanic arc granites (VAG; Pearce, Harris & Tindle, Reference Pearce, Harris and Tindle1984), although LIL elements may be highly mobile during post-magmatic processes. On discrimination diagrams of Pearce, Harris & Tindle (Reference Pearce, Harris and Tindle1984; Fig. A4), rocks of type G1 cluster well within the VAG field. Their general geochemical features as peraluminous and calc-alkaline rocks are also in line with a probable subduction-related setting (cf. Barbarin, Reference Barbarin1999).
Samples of type G2 are also dispersed mostly within the VAG field but close to (or straddling) the boundary with ORG and WPG. On the Al2O3 versus SiO2 and FeOT/(FeOT+MgO) versus SiO2 (Fig. A5a, b) discrimination diagrams, utilizing relatively immobile major elements, the analytical points representing type G2 gneisses cluster within the post-orogenic granitoid (POG) field and are well separated from gneisses of type G1. In addition, gneisses of type G2 display flat HREE patterns, only moderate LREE-enrichment (Fig. 6) and smooth patterns for HFS elements close to the mean value on the ORG-normalized diagram. Therefore, their chemical characteristics are difficult to interpret in terms of a specific geodynamic environment, similar to the early Palaeozoic gneisses of the Central and West Sudetes (cf. Aleksandrowski et al. Reference Aleksandrowski, Kryza, Mazur, Pin and Zalasiewicz2000).
Since the gneisses of the eastern Fore-Sudetic Block are exposed in the boundary zone between the Central and East Sudetes, we tested their geochemical affinity to these adjoining domains. Trace elements including REE are normalized against the composition of Orlica–Śnieżnik and Keprnik gneisses typical of the Central and East Sudetes, respectively (Fig. 7). This comparison demonstrates a geochemical affinity of the Strzelin and Nowolesie gneisses (type G1) to the Keprnik gneiss (Fig. 7a), whereas several gneiss varieties of type G2 are generally similar to the Orlica–Śnieżnik gneisses (Fig. 7b).

Figure 7. Analysed gneisses from the eastern Fore-Sudetic Block, normalized against: (a) average composition of the Keprnik gneiss (from Souček, Jelínek & Bowes, Reference Souček, Jelínek, Bowes and Kukal1992); (b) average composition of the Orlica-Śnieżnik gneiss (from Turniak et al. Reference Turniak, Mazur and Wysoczanski2000 and Lange et al. Reference Lange, Bröcker, Mezger and Don2002, Reference Lange, Bröcker, Armstrong, Żelaźniewicz, Trapp and Mezger2005). G1 – Strzelin and Nowolesie gneisses (samples S1, S2, S5, S6A, S6B); G2 – Lipniki, Doboszowice, Gościęcice and Maciejowice gneisses (samples S3, S4, S7, S8A, S8B, S9).
7. SHRIMP II zircon ages and interpretation
The analytical data for samples S3, S4, S6 and S8 are included in Table A1 (online Appendix at http://journals.cambridge.org/geo). Cathodoluminescence images of zircons with SHRIMP analytical spots are shown in Figure 8 and, in higher resolution, in Figure A6 (online Appendix at http://journals.cambridge.org/geo). Results of SHRIMP II zircon analyses are presented in the concordia diagrams of Figure 9. The majority of zircons in sample S6 are near-idiomorphic, normal- to long-prismatic with colours ranging from dark brown to colourless and transparent (Figs 8, A6). Their morphology is dominated by {211} and {110} with minor {101} and {100} forms. Oscillatory zoning is interpreted to indicate crystallization from a melt. A few grains with similar morphology and dark colour have rounded terminations. Table A1 shows the results of 24 analyses performed on 17 grains (Figs 8, A6). The isotopic data for 12 spots define a discordia line (MSWD = 0.48) with an upper concordia intercept at 576±19 Ma and a similar weighted mean 207Pb/206Pb age of 575±15 Ma (Fig. 9a). In view of the magmatic nature of the zircons, we interpret the upper intercept age to reflect the time of emplacement of the precursor granite. Our results are in accord with SHRIMP ages in the range of 600–590 Ma obtained for the Nowolesie gneiss by Klimas (Reference Klimas2008). Some data scatter along the concordia curve, visible for this age component, and this probably results from Pb loss. Most analysed zircons contain rounded or angular cores of different age, reflecting a detrital character in the magma source. Inherited grains are usually more discordant than newly formed magmatic zircons. The calculated 207Pb/206Pb ages (not shown in Fig. 9a) mostly fall into the Mesoproterozoic age range: c. 1200 Ma (two cores), c. 1350 Ma (one core), c. 1500–1600 Ma (four cores). Only one core yielded an older age of c. 1750 Ma (Table A1).

Figure 8. Cathodoluminescence images of zircons with SHRIMP analytical spots from gneiss samples S6, S8, S4 and S3.

Figure 9. Concordia diagrams showing some results of SHRIMP II zircon analyses from gneiss samples S6 (a), S8 (b), S4 (c) and S3 (d) in the eastern Fore-Sudetic Block.
Zircons from sample S8 are generally clear, idiomorphic, normal- to long-prismatic with {110} and {211} forms better developed than {100} and {101}. Most zircons contain inherited cores mantled by magmatic rims, the latter showing oscillatory zoning (Figs 8, A6). SHRIMP dating of 14 domains representing magmatic components produced a Concordia age of 556.9±9.3 Ma (Table A1, Fig. 9b). Four cores yielded Meosoproterozoic 207Pb/206Pb ages ranging from 1314±18 Ma to 1552±12 Ma. The oldest cores are Palaeoproterozoic with ages at 1705±23 Ma, 1979±12 and 2377±7 Ma (Table A1).
Zircons in sample S4 are clear to light grey, stubby to long-prismatic, and most grains are idiomorphic or have slightly rounded terminations, whereas a few grains have somewhat more rounded ends (Figs 8, A6). Many grains contain ovoid cores, sometimes with preserved oscillatory zoning. A mean 206Pb/238U age of 500±12 Ma was obtained from nine analyses (Table A1; Fig. 9c). The result shows poor MSWD at about 15, due to negative discordance that is caused by common lead overcorrection resulting from negligible 204Pb overmeasurement. If 204Pb is nulled the data are concordant, producing and age of 511.6±7.7 Ma (2σ, MSWD = 2.0, probability of concordance = 0.16). It is worth noting that three zircons (6.1, 10.1, 12.1; Figs 8, A6) give a mean 206Pb/238U age of 453±14 Ma (Table A1; not shown in Fig. 9c). The geological meaning of this age remains unknown. Three analyses of inherited cores (not shown in Fig. 8) yielded 207Pb/206Pb ages in the range of approximately 1640–1830 Ma, and one core has an Archaean age of c. 2900 Ma. One spot with 238U/206Pb ages of c. 590 Ma (Table A1) possibly reflects a Neopoterozoic crustal source that was involved in the generation of the Gościęcice gneiss precursor.
Zircons from sample S3 are predominantly idiomorphic, short- to normal-prismatic, colourless and transparent (Figs 8, A6). Their morphologies are dominated by {211} and {110} with subordinate {101} and {100} forms. Three components of different age can be recognized (Table A1): (1) inherited cores (not shown in Fig. 9d; one spot with a 207Pb/206Pb age of 2012±37 Ma), (2) magmatic zircons with a mean age of 488.4±6.2 Ma (Fig. 9d), reflecting the age of protolith emplacement, and (3) the outer, high-uranium rim (dark in CL) of one grain with a significantly younger 238U/206Pb age of 279.1±4.4 Ma (not shown in Fig. 9d). Both the zircon characteristics and the c. 488 Ma age for the gneiss protolith strongly resemble those known from the Orlica–Śnieżnik granite-gneisses. The only difference is a significantly younger age for the high-uranium outer rim of unknown origin, possibly related to fluid activity.
The presence of zircon xenocrysts in all the above samples clearly documents the involvement of older crust in the generation of the protolith granitoid magmas and they therefore classify as crustal melts. In the case of samples S6 and S8, a Mesoproterozoic to latest Palaeoproterozoic basement, with some additions of Palaeoproterozoic and Archaean components, was involved and probably exists at the lower crustal levels. The occurrence of latest Palaeoproterozoic zircon cores in sample S4 needs further verification due to the high discordance of obtained results (Table A1).
8. Pb/Pb ages and interpretation
The analytical data are presented in Table A2 (online Appendix at http://journals.cambridge.org/geo), and most 207Pb/206Pb spectra are shown in the histograms of Figure 10, which permit visual assessment of the data distribution from which the ages are derived. The evaporation technique only provides Pb isotopic ratios, and there is no a priori way to determine whether a measured 207Pb/206Pb ratio reflects a concordant age. Thus, principally, all 207Pb/206Pb ages determined by this method are necessarily minimum ages. However, many studies have demonstrated that there is a very strong likelihood that these data represent true zircon crystallization ages (1) when the 207Pb/206Pb ratio does not change with increasing temperature of evaporation and/or (2) when repeated analyses of grains from the same sample at high evaporation temperatures yield the same isotopic ratios within error. Comparative studies by single grain evaporation, conventional U–Pb dating and ion-microprobe analysis have shown this to be correct (e.g. Kröner, Byerly & Lowe, Reference Kröner, Byerly and Lowe1991; Kröner et al. Reference Kröner, Wilde, O'Brien, Li, Passchier, Walte and Liu2005 and references cited therein).

Figure 10. Histograms showing distribution of radiogenic lead isotope ratios derived from evaporation of single zircons from granite-gneisses derived from early Palaeozoic protoliths in the Orlica–Śnieżnik Massif, western Poland. (a) Spectrum for three grains from Śnieżnik gneiss sample PL26, integrated from 330 ratios. (b) Spectrum for five grains from Gierałtów gneiss sample PL27a, integrated from 530 ratios. (c) Spectrum for three grains from pink granite gneiss-gneiss sample PL27, integrated from 336 ratios.
The zircon population in sample PL26 is predominantly euhedral, long-prismatic and clear to light yellow-brown, and some grains display slight rounding at their terminations. Three grains yielded similar Pb isotopic ratios that can be combined to a mean 207Pb/206Pb age of 500.2±1.0 Ma (Table A2, Fig. 10a), which we interpret to approximate the age of protolith emplacement. This is identical, within error, to the SHRIMP zircon age of 495±7 Ma as reported by Turniak, Mazur & Wysoczanski (Reference Turniak, Mazur and Wysoczanski2000).
Zircons in sample PL27a are euhedral, stubby to long-prismatic and colourless to light grey, rather similar to those in sample PL26. Evaporation of five zircons produced identical isotopic ratios with a mean 207Pb/206Pb age of 501.0±1.0 Ma (Table A2, Fig. 10b), which is identical to the age of Śnieżnik sample PL26 and reflects the time of protolith emplacement.
Zircons from sample PL27 are clear to yellow, idiomorphic and long-prismatic, and evaporation of three grains yielded a mean 207Pb/206Pb age of 503.5±1.0 Ma (Table A2, Fig. 10c). This age, interpreted to reflect emplacement of the gneiss precursor, is identical to those of other granitoid gneisses of the Orlica–Śnieżnik Massif (e.g. Oliver, Corfu & Krogh, Reference Oliver, Corfu and Krogh1993; Kröner et al. Reference Kröner, Jaeckel, Hegner and Opletal2001; Štípská, Schulmann & Kröner, Reference Štípská, Schulmann and Kröner2004) and those reported above.
9. Discussion
The protoliths of granitoid orthogneisses from the contact zone between the Central and East Sudetes can be classified into two age groups: (1) late Neoproterozoic granites, presumably derived from the basement of the Brunian microplate and (2) early Palaeozoic c. 500 Ma granites, comparable to those in the Orlica–Śnieżnik Massif. This subdivision does not correspond with the main geochemical diversity among the calc-alkaline gneisses that include magmas emplaced in a supra-subduction setting (type G1) and peraluminous orthogneisses of uncertain geodynamic affinity (type G2).
The Strzelin and Nowolesie orthogneisses with some geochemical features resembling volcanic arc granites (type G1) belong to the Proterozoic age group. They are geochemically similar to the Neoproterozoic Keprnik gneisses of the East Sudetes, which also show considerable zircon inheritance (Kröner et al. Reference Kröner, Štípská, Schulmann, Jaeckel, Franke, Haak, Oncken and Tanner2000) and constitute a nappe derived from the Brunian basement (Schulmann & Gayer, Reference Schulmann and Gayer2000). In agreement with earlier studies of the Strzelin and Keprnik gneisses (Van Breemen et al. Reference van Breemen, Aftalion, Bowes, Dudek, Misař, Povondra and Vrana1982; Kröner et al. Reference Kröner, Štípská, Schulmann, Jaeckel, Franke, Haak, Oncken and Tanner2000; Oberc-Dziedzic et al. Reference Oberc-Dziedzic, Klimas, Kryza and Fanning2003), our data confirm their late Neoproterozoic age. The geochemical characteristics of the Strzelin, Nowolesie and Keprnik gneisses are consistent with data for Neoproterozoic gneisses of the Brno Massif (Fig. 1), representing the surface exposure of the Brunian microplate (Dudek, Reference Dudek1980). Following the interpretation of Neoproterozoic magmatism in the Brno Massif (Hanžl & Melichar, Reference Hanžl and Melichar1997; Finger et al. Reference Finger, Hanžl, Pin, Quadt, Steyrer, Franke, Haak, Oncken and Tanner2000), the Nowolesie gneisses could have been derived from the supra-subduction emplacement of calc-alkaline magmas. Generation and emplacement of their protolith were presumably related to Neoproterozoic subduction of an oceanic domain beneath the active Gondwana margin (Nance & Murphy, Reference Nance, Murphy, Nance and Thompson1996).
The Maciejowice orthogneiss, despite its Neoproterozoic age (560 Ma), reveals geochemical characteristics (type G2) similar to those of the Gościęcice and Doboszowice gneisses, the magmatic precursors of which are dated at c. 500 Ma. At the same time, the geochemical signature of the Maciejowice gneiss differs from that which is shown by only somewhat older Strzelin and Nowolesie gneisses (575–600 Ma). One possible explanation could be a change of tectonic regime between 575 and 560 Ma from supra-subduction to post-collisional since the Maciejowice gneiss displays some resemblance to post-orogenic granites (Figs A4, A5). If this was the case, the age of the Maciejowice orthogneiss would provide an upper time limit for the amalgamation of the Brunia microplate that is postulated to have occurred because of a terrane collision (Finger et al. Reference Finger, Hanžl, Pin, Quadt, Steyrer, Franke, Haak, Oncken and Tanner2000). On the other hand, the apparent diversity among the Neoproterozoic gneisses may indicate the inability of the geochemical method applied to successfully discriminate between tectonic settings of magma generation.
The c. 500 Ma old gneisses (Doboszowice, Gościęcice, Orlica–Śnieżnik Massif) are similar in terms of age and geochemical signature to early Palaeozoic orthogneisses which are widespread throughout the Variscan belt (cf. Aleksandrowski et al. Reference Aleksandrowski, Kryza, Mazur, Pin and Zalasiewicz2000). The protoliths of the latter are related to supra-subduction (Oliver, Corfu & Krogh, Reference Oliver, Corfu and Krogh1993; Kröner & Hegner, Reference Kröner and Hegner1998) or rift-related tectonic settings (e.g. Oberc-Dziedzic, Pin & Kryza, Reference Oberc-Dziedzic, Kryza, Klimas, Fanning and Madej2005), based on geological and geochemical evidence. We do not attempt to resolve this controversy, since the c. 500 Ma gneisses reveal complex geochemical characteristics and may be derived from a mixture of different crustal components. Nevertheless, we stress the resemblance between the Gościęcice gneiss and those in the Central and West Sudetes, such as in the Orlica–Śnieżnik Massif.
The Nowolesie (S6) and Maciejowice (S8) gneisses contain inherited material, which implies the contribution from a Mesoproterozoic basement to the source of granite magma within the Brunian microplate. Consequently, our results indicate an affinity of the Brunian microcontinent to terranes which contain a record of Mesoproterozoic tectono-thermal activity (e.g. Amazonian Craton, West Avalonia; Priem et al. Reference Priem, Kroonenberg, Beolrijk and Hebeda1989; Restrepo-Pace et al. Reference Restrepo-Pace, Ruiz, Gehrels and Cosca1997; Nance & Murphy, Reference Nance, Murphy, Nance and Thompson1996; Keppie, Davis & Krogh, Reference Keppie, Davis and Krogh1998; Ruiz et al. Reference Ruiz, Tosdal, Restrepo, Murrillo-Muneton, Ramos and Keppie1999). The East Avalonian affinity of Brunia is also consistent with the palaeomagnetic results of Nawrocki et al. (Reference Nawrocki, Żylińska, Buła, Grabowski, Krzywiec and Poprawa2004), despite the different palaeogeographic interpretation favoured by these authors (J. Nawrocki, pers. comm.). In contrast, gneisses occurring in the Central Sudetes are devoid of Mesoproterozoic inherited material, as is shown by the Doboszowice (S3) and Gościęcice (S4) orthogneisses or gneisses of the Orlica–Śnieżnik Massif (Turniak, Mazur & Wysoczanski, Reference Turniak, Mazur and Wysoczanski2000; Lange et al. Reference Lange, Bröcker, Armstrong, Żelaźniewicz, Trapp and Mezger2005; Żelaźniewicz et al. Reference Żelaźniewicz, Nowak, Larionov and Presnyakov2006; Bröcker et al. Reference Bröcker, Klemd, Cosca, Brock, Larionov and Rodionov2009). The lack of the Mesoproterozoic inheritance emphasizes the dissimilarity of the Variscan domain and the Brunia microplate.
If the Brunia microplate is an Avalonian-type terrane, its Cambrian separation from Gondwana seems unlikely. Indeed, East Avalonia did not drift away from Gondwana before the middle Arenigian (480 Ma; e.g. Prigmore, Butler & Woodcock, Reference Prigmore, Butler and Woodcock1997; Pollock, Hibbard & Sylvester, Reference Pollock, Hibbard and Sylvester2009). Therefore, even if the intrusive contact between the Gościęcice gneiss (c. 500 Ma) and its Neoproterozoic country rocks cannot be ruled out based on the structural data available, this will not obstruct our interpretation. The magmatism associated with rifting of Avalonia could have affected the western margin of Brunia before its separation from Gondwana. Such a possibility is even suggested by the occurrence of latest Palaeoproterozoic zircon cores in the analysed sample of the Gościęcice gneiss (S4). The inherited zircon component of this age is not typical of the Armorican terranes (Friedl et al. Reference Friedl, Finger, McNaughton and Fletcher2000), but due to the high discordance of the above mentioned analyses, much confidence cannot be attached to them. Nevertheless, the fingerprints of c. 500 Ma tectonothermal activity were also found by Kröner et al. (Reference Kröner, Štípská, Schulmann, Jaeckel, Franke, Haak, Oncken and Tanner2000) in the Brunian basement exposed further south in the East Sudetes (Desná Dome; Fig. 2).
Following this line of reasoning, c. 500 Ma magmatism can be assumed to have taken place simultaneously with the separation of Brunia from the adjacent peri-Gondwana terrane(s) along an incipient rift (Fig. 11a), analogous to the Staré Město rift of Kröner et al. (Reference Kröner, Štípská, Schulmann, Jaeckel, Franke, Haak, Oncken and Tanner2000). The synkinematic emplacement of a magmatic precursor to the Doboszowice orthogneiss (Mazur, Puziewicz & Józefiak, Reference Mazur, Puziewicz and Józefiak1995) may suggest migmatization and deformation coeval with this tectonic event. The expansion of the Staré Město rift or its more westerly equivalent led to separation of the Brunian microplate from the Variscan realm. The Staré Město oceanic tract separating Brunia from Armorican terranes of the Variscan belt was closed by the end of Devonian times (Fig. 11b). The Brunia microcontinent was ultimately juxtaposed against the Variscan belt during Early Carboniferous collision (Schulmann & Gayer, Reference Schulmann and Gayer2000), which resulted in a thrust contact between these two contrasting domains, dominated respectively by Proterozoic and early Palaeozoic crust (Fig. 11c).

Figure 11. Hypothetical geodynamic scenario for Palaeozoic evolution of the western margin of Brunia and related magmatism (much simplified). (a) Early Ordovician (c. 500 Ma) incipient rifting (in a back-arc setting according to Kröner et al. Reference Kröner, Štípská, Schulmann, Jaeckel, Franke, Haak, Oncken and Tanner2000) and associated granitoid magmatism within attenuated continental crust; initial separation of Brunia from other peri-Gondwana terranes. (b) Latest Devonian closure of the oceanic tract separating Brunia from Armorican terranes of the Variscan belt. (c) Central Sudetes juxtaposed against Brunia along partly cryptic suture.
10. Conclusions
The occurrence of Mesoproterozoic inherited material in the granitoids intruded in the Proterozoic basement of Brunia supports earlier interpretations suggesting an East Avalonian affinity for this microcontinent (Moczydłowska, Reference Moczydłowska1997; Friedl et al. Reference Friedl, Finger, McNaughton and Fletcher2000; Finger et al. Reference Finger, Hanžl, Pin, Quadt, Steyrer, Franke, Haak, Oncken and Tanner2000). This evidence is based on the occurrence of comparable Mesoproterozoic crustal components in the Avalon terrane and in the Amazonian Craton (Restrepo-Pace et al. Reference Restrepo-Pace, Ruiz, Gehrels and Cosca1997; Nance & Murphy, Reference Nance, Murphy, Nance and Thompson1996; Keppie, Davis & Krogh, Reference Keppie, Davis and Krogh1998; Ruiz et al. Reference Ruiz, Tosdal, Restrepo, Murrillo-Muneton, Ramos and Keppie1999) which bordered one another before the Early Palaeozoic break-up of the northern Gondwana margin. The Avalonian affinity of Brunia precludes large-scale Cambrian separation between the Brunian microcontinent and other peri-Gondwanan terranes as deduced from biogeographic and palaeomagnetic arguments (e.g. Belka et al. Reference Belka, Ahrendt, Franke, Wemmer, Franke, Haak, Oncken and Tanner2000; Winchester et al. Reference Winchester2002; Nawrocki et al. Reference Nawrocki, Żylińska, Buła, Grabowski, Krzywiec and Poprawa2004).
The occurrence of gneisses derived from Late Cambrian to earliest Ordovician granitic protolith and devoid of inherited Mesoproterozoic zircon cores confirms the Armorican affinity of the Variscan domain adjacent to the Brunia microplate from the west. The suture zone between Brunia and the Variscan belt, probably equivalent to the Rheic suture, is located between the East and Central Sudetes, where it is constrained by the distribution of orthogneisses having or lacking Mesoproterozoic inheritance, respectively.
Acknowledgements
A. K. acknowledges mass spectrometer analytical facilities in the Max-Planck-Institut für Chemie in Mainz. The research of S. M., J. S. and K. T. was funded by the University of Wrocław grants 1017/S/ING and 2022/W/ING. We thank Prof. J. Don for providing samples PL26, 27 and 27a. This is a contribution to IGCP-Project 497 and contribution no. 44 of the Geocycles Cluster of the Johannes-Gutenberg-Universität Mainz, funded by the State of Rhineland-Palatinate. Reviews by M. Bröcker and an anonymous reviewer helped to clarify our presentation and are much appreciated. We are thankful to F. Finger and an anonymous reviewer for comments on an earlier version of the manuscript.