INTRODUCTION
Lake Tanganyika is the largest and oldest of the East African lakes (Cohen et al. Reference Cohen, Lezzar, Tiercelin and Soreghan1997). With about 250 cichlid and 75 other fish species, it is not the most speciose lake (Snoeks, Reference Snoeks2000). However, its cichlid fauna displays the highest ecological and morphological diversity. The level of endemicity and the extent to which non-cichlid fishes developed into species flocks surpass the other major lakes in the region, Malawi and Victoria (Coulter, Reference Coulter and Coulter1991b; Snoeks, Reference Snoeks2000; Salzburger et al. Reference Salzburger, Meyer, Baric, Verheyen and Sturmbauer2002). Considering the substantial number of potential host species in tropical hotspots of biodiversity, such as the East African Great Lakes (Galis and Metz, Reference Galis and Metz1998; Snoeks, Reference Snoeks2000), it is anticipated that parasitological surveys will lead to the discovery of many new parasite species (e.g., Whittington, Reference Whittington1998).
Monogenea are very common flatworm parasites of bony fish (though other aquatic cold-blooded vertebrates, some invertebrate taxa, and Hippopotamus are possible hosts as well), of which they usually infect the gills and fins; they have a one-host life-cycle (Pugachev et al. Reference Pugachev, Gerasev, Gussev, Ergens and Khotenowsky2009). Within this class, several genera, of which Gyrodactylus von Nordmann, 1832 and Cichlidogyrus Paperna, 1960 are well-known examples, are pathogens of fish in aquaculture. The genus Gyrodactylus is expected to comprise tens of thousands of species when using the rather conservative estimate of, on average, 1 species per species of fish host (Bakke et al. Reference Bakke, Harris and Cable2002, Reference Bakke, Cable and Harris2007; Harris et al. Reference Harris, Shinn, Cable and Bakke2004). Hence, we can assume that only a small fraction of its global diversity has been described thus far. Although Gyrodactylus is expected to be so speciose, almost no species have been described from cichlids, in contrast to Cichlidogyrus. Pariselle and Euzet (Reference Pariselle and Euzet2009) recorded 71 species of Cichlidogyrus in Africa and the Middle East, mainly from cichlids. The number of Gyrodactylus species from African freshwater fish amounts to only 18, according to Christison et al. (Reference Christison, Shinn and van As2005), of which only 4 were collected from cichlids. Gyrodactylus thlapi Christison, Shinn and van As, Reference Christison, Shinn and van As2005 was described from the cichlid Pseudocrenilabrus philander philander (Weber, 1897) in Botswana. G. cichlidarum Paperna, 1968 was collected from various tilapiine hosts, G. haplochromii Paperna, 1973 was described from Haplochromis angustifrons Boulenger, 1914 in Lake George, and G. nyanzae Paperna, 1973 from Oreochromis variabilis (Boulenger, 1906) in Lake Victoria. The only record of Gyrodactylus from the Great Lake is by Blais et al. (Reference Blais, Rico, van Oosterhout, Cable, Turner and Bernatchez2007), yet without species assignment. According to Paperna (Reference Paperna1979), G. cichlidarum has the widest host range of the 3 species known at that time. Recently, G. ergensi was described by Přikrylová et al. (Reference Přikrylová, Matĕjusová, Musilová and Gelnar2009a) from Sarotherodon galilaeus (Linnaeus, 1758) and O. niloticus in Senegal. Two additional species parasitizing African cichlids were described by Cone et al. (Reference Cone, Arthur and Bondad-Reantaso1995) from cultured Nile tilapia in the Philippines, namely G. niloticus and G. shariffi. The former was reported by García-Vásquez et al. (Reference García-Vásquez, Hansen and Shinn2007) to be synonymous to G. cichlidarum. Despite the paucity of knowledge about African gyrodactylids, they may be economically important, as they have the potential to be notoriously pathogenic (Paperna, Reference Paperna1996), for example, in captive tilapia (García-Vásquez et al. Reference García-Vásquez, Hansen and Shinn2007).
Within the Gyrodactylidae, several morphological criteria, such as the distribution of marginal hooks, the presence of additional haptoral elements, and the arrangement of male copulatory organ (MCO) spines, are used to support new gyrodactylid genera and to hypothesize about the relationships among them (e.g., Luus-Powell et al. Reference Luus-Powell, Mashego and Khalil2003; Vianna et al. Reference Vianna, Boeger and Dove2007). However, Kritsky and Boeger (Reference Kritsky, Boeger, Combes and Jourdane2003) found several gyrodactylid genera to cluster among Gyrodactylus spp. in phylogenetic analyses, indicating that the latter is paraphyletic. Hence, the status of genera within the family, and their interrelationships, are far from understood. Africa has recently seen the discovery of several morphologically deviant gyrodactylid genera, such as Mormyrogyrodactylus Luus-Powell, Mashego and Khalil, Reference Luus-Powell, Mashego and Khalil2003 (MCO structure and armament, accessory bars) and Diplogyrodactylus Přikrylová, Matĕjusová, Musilová, Gelnar and Harris, 2009 (no dorsal bar, two types of marginal hooks, tubular MCO, a pair of muscular adhesive discs). The (re)descriptions mention some similarities with, among others, the African clawed toad parasite Gyrdicotylus Vercammen-Grandjean, 1960 and with Macrogyrodactylus Malmberg, 1957, a common parasite on African fish (see also Vianna et al. Reference Vianna, Boeger and Dove2007). Hence, further research into diversity and phylogeny of African Gyrodactylidae seems important.
Lake Tanganyika's cichlids are classified into tribes, based on Poll (Reference Poll1986). One of these tribes, the endemic Tropheini, comprises a highly diverse assemblage, mostly of rock-dwelling algae scrapers. They are maternal mouth-brooders with a range of trophical morphological adaptations (Poll, Reference Poll1986; Sturmbauer et al. Reference Sturmbauer, Hainz, Baric, Verheyen and Salzburger2003). Most of the representatives studied are host to Cichlidogyrus spp. attached to their gills and to few or no Gyrodactylus (nobis). An interesting exception is the genus Simochromis Boulenger, 1898. Its members, comprising 5 species, are grazers inhabiting shallow rocky habitats with a wide distribution throughout Lake Tanganyika (Meyer et al. Reference Meyer, Knowles and Verheyen1996; Koblmüller et al. Reference Koblmüller, Egger, Sturmbauer and Sefc2010). A considerable number of Gyrodactylus individuals were found on the largest species in the genus, S. diagramma (Günther, 1983), often living in co-existence on one host or in one gill chamber with Cichlidogyrus spp. The present study, including the description of 3 new species, represents the first record of Monogenea in Lake Tanganyika, and is the second paper to include molecular data for African Gyrodactylus sampled in their natural habitat.
MATERIALS AND METHODS
Sampling
Host cichlid fish were collected using hand and gill nets in the littoral rocky habitat at Kalambo Lodge (8°37′S, 31°12′E) (Zambia). They were identified to species level by C. Sturmbauer (Karl-Franzens University, Graz, Austria) and stored in pure ethanol by species. In the laboratory, the body, fins and gills, as well as the recipient's ethanol, were inspected for parasites under an Olympus SZX12 stereomicroscope. Monogenea were removed with a dissection needle. They were either stored in 5 μl of H20 for molecular work, or mounted on a slide for morphological analysis. On some specimens morphological and genetic data were collected simultaneously by severing the haptor from the body. In this case the anterior end was stored awaiting genetic processing, whereas the haptor was used for morphological research. In some cases the complete individual was used for genetic analysis, after photographing the animal, allowing a posteriori comparison between morphology and DNA sequencing results. Taxon and author names of fish in this study follow Eschmeyer and Fricke (Reference Eschmeyer and Fricke2009).
Morphometric analysis
Specimens were mounted on a slide in milli-Q water and fixed under a cover-slip using ammonium picrate-glycerine (Malmberg, Reference Malmberg1970). On some individuals, partial digestion through proteinase K treatment was carried out following Harris and Cable (Reference Harris and Cable2000) to render the hard parts more visible. Pictures and measurements were taken based on Shinn et al. (Reference Shinn, Hansen, Olstad, Bachmann and Bakke2004) using an Olympus BX50 microscope at a magnification of 100× (oil immersion, 10× ocular) and Olympus DP-Soft 3.2 software. Principal Component Analysis on the correlation matrix of the haptor morphometrics in Statistica 8.0 (StatSoft, Inc, 2008) allowed detection of clusters representing morphospecies. Because of intra-individual variation of the marginal hook shaft and the flexibility of the dorsal bar, the marginal hook total length and shaft length and the dorsal bar length were omitted from the PCA. Body size parameters were also omitted because of the ethanol fixation process (causing, for example, distortion), and because of the varying degree of flattening of the body. Unfortunately, the ethanol fixation we used in this study did not allow a reliable study of the soft body parts, although these are considered systematically informative (Malmberg, Reference Malmberg1970; Pugachev et al. Reference Pugachev, Gerasev, Gussev, Ergens and Khotenowsky2009). Hence, our morphological results are limited to the hard body parts.
Molecular and phylogenetic analysis
DNA was extracted after addition of 5μl of a double-concentrated lysis solution containing 1× PCR buffer (Eurogentec), 0·45% Tween 20 (Merck), 0·45% NP40 (Calbiochem) and 60 μg.L−1 proteinase K (Sigma). Enzymatic digestion was carried out at 65 °C for 25 min, followed by inactivation of the enzyme at 95°C for 10 min. Polymerase Chain Reaction (PCR) was performed using a GeneAmp PCR system 2700 thermocycler (Applied Biosystems). The reaction volume of 25 μl contained 2·5 μl of 10× PCR buffer (Eurogentec), 2·5 μl of dNTPs (2 mm) (Amersham Pharmacia Biotech), 1 μl of MgCl2 (50 mm) (Eurogentec), 0·2 μl of Taq Polymerase (Eurogentec), 1 μl of each primer (20 μ m) (Eurogentec), 1 μl of template DNA, topped up with milli-Q water. Primers used were ITS1A (5′-GTAACAAGGTTTCCGTAGGTG-3′) and ITS2 (5′-TCCTCCGCTTAGTGATA-3′) (Matĕjusová et al. Reference Matĕjusová, Gelnar, McBeath, Collins and Cunningham2001), spanning the first and second internal transcribed spacers (ITS-1 and ITS-2) and intervening 5·8S rDNA. This region has been proven to be useful in classifying and distinguishing Gyrodactylus spp. in agreement with species identification and delineation based on morphology (Matĕjusová et al. Reference Matĕjusová, Gelnar, McBeath, Collins and Cunningham2001; Meinilä et al. Reference Meinilä, Kuusela, Ziętara and Lumme2002; Ziętara et al. Reference Ziętara, Huyse, Lumme and Volckaert2002; Huyse et al. Reference Huyse, Audenaert and Volckaert2003, Reference Huyse, Malmberg and Volckaert2004; Ziętara and Lumme, Reference Ziętara and Lumme2004). After an initial denaturation for 3 min at 96°C, samples were subjected to 35 cycles of 50 s at 95°C, 50 s at 52°C and 50 s at 72°C. After a final elongation of 7 min at 72°C, samples were cooled to 4°C. PCR products were purified with NucleoFast (Macherey-Nagel), according to the manufacturer's guidelines, and sequenced applying a 1/8 dilution of the Big Dye Terminator 3.1 sequencing protocol (Applied Biosystems). Finally products were run on an ABI PRISM 3130 Avant Genetic Analyser automated sequencer (Applied Biosystems). In addition to the initial PCR primers, the internal primers ITSR3A (5′-GAGCCGAGTGATCCACC-3′) and ITS2F (5′-TGGTGGATCACTCGGCTCA-3′) (Matĕjusová et al. Reference Matĕjusová, Gelnar, McBeath, Collins and Cunningham2001) were used.
For the phylogenetic analyses, we retrieved the 3 currently known sequences from African Gyrodactylus species: G. cichlidarum isolated from captive United Kingdom Oreochromis niloticus niloticus (Linnaeus, 1758), G. ergensi from Sarotherodon galilaeus collected in Niokolo Koba National Park, Senegal and G. eyipayipi Vaughan, Christison, Hansen and Shinn, Reference Vaughan, Christison, Hansen and Shinn2010 from captive greater pipefish Syngnathus acus Linnaeus, 1758. Furthermore, in order to situate the new species within the known diversity of Gyrodactylus spp. and the Gyrodactylidae at large, representatives were included of each of the subgenera described by Malmberg (Reference Malmberg1970), with some extra taxa to include all clades retrieved by Ziętara and Lumme (Reference Ziętara and Lumme2004), including the 3 sequenced South American species. The other Gyrodactylidae genera of which sequences are available were also added (Table 1).
Table 1. List of published sequences used in this study

a Shown to be a member of a clade including members of the G. (Mesonephrotus), G. (Paranephrotus) and G. (Metanephrotus) subgenera, as well as previously unassigned species, by Ziętara and Lumme (Reference Ziętara and Lumme2004).
b Suggested to belong to this subgenus by Ziętara and Lumme (Reference Ziętara and Lumme2004) based on phylogenetic results.
c Member of the wageneri-group of G. (Limnonephrotus).
d Reported to cluster with the rugiensis-group of G. (Paranephrotus) in Ziętara and Lumme (Reference Ziętara and Lumme2004).
e Clustering together in Kritsky and Boeger (Reference Kritsky, Boeger, Combes and Jourdane2003) and Ziętara and Lumme (Reference Ziętara and Lumme2004).
Sequences were aligned by MUSCLE (Edgar, Reference Edgar2004) using default distance measures and sequence weighting schemes. The resulting alignment was trimmed with trimAl v.1.2 (Capella-Gutiérrez et al. Reference Capella-Gutiérrez, Silla-Martínez and Gabaldón2009), making use of an automated trimming heuristic optimized for subsequent Maximum Likelihood (ML) phylogenetic tree reconstruction. The ITS region is challenging to align as a whole, especially due to ITS-1, which is known to display substantial length variation that limits meaningful alignments to subgenera of Gyrodactylus (Huyse and Volckaert, Reference Huyse and Volckaert2002; Ziętara and Lumme Reference Ziętara and Lumme2002, Reference Ziętara and Lumme2004). Due to the lack of signal in the ITS-1 region (and to a lesser extent ITS-2) when comparing distant Gyrodactylus species, some authors restrict the comparison to the coding and thus more conserved 5·8S rDNA. Hence, alignment and trimming (omitting all but a small portion of the spacer region) was carried out separately for the 5·8S rDNA gene and for ITS-2, retaining a dataset with a length of 157 and 275 bp, respectively. In subsequent analyses, 5·8S rDNA was combined with ITS-2, whereas ITS-1 was left out (following e.g. Ziętara et al. Reference Ziętara, Huyse, Lumme and Volckaert2002; Kritsky and Boeger, Reference Kritsky, Boeger, Combes and Jourdane2003). Using an ML optimized base tree, jModelTest 0.1.1 (Posada, Reference Posada2008; see also Guindon and Gascuel, Reference Guindon and Gascuel2003; Felsenstein, Reference Felsenstein2005) was used to estimate the optimal model of molecular evolution. Based on the corrected Akaike Information Criterion (AICc) (Hurvich and Tsai, Reference Hurvich and Tsai1989), the TVM (Posada, Reference Posada, Baxevanis, Davison, Page, Petsko, Stein and Stormo2003) + Γ model was selected for the 5·8S rDNA + ITS-2 dataset. In view of the subsequent implementation in phylogenetic software, we opted for the model with the second best corrected Akaike score, namely the GTR (Tavaré, Reference Tavaré and Miura1986; Rodriguez et al. Reference Rodriguez, Oliver, Marin and Medina1990) + Γ model, with a gamma-shape parameter of 0·42. Under this model, a maximum likelihood (ML) search was carried out in PhyML v.3.0 (Guindon and Gascuel, Reference Guindon and Gascuel2003), assessing nodal support through 1000 bootstrap samples using the nearest-neighbour interchange branch swapping algorithm. PAUP* v.4.01b (Swofford, Reference Swofford2001) with the PaupUp interface (Calendini and Martin, Reference Calendini and Martin2005) was used for the maximum parsimony method (MP), in which gaps were treated as a fifth character, and for calculating the genetic distances according to the model selected. The model was also used in Bayesian analysis, implemented in MrBayes v.3 (Huelsenbeck and Ronquist, Reference Huelsenbeck and Ronquist2001; Ronquist and Huelsenbeck, Reference Ronquist and Huelsenbeck2003). Posterior probabilities were calculated over 2.106 generations (after which stationarity of the Markov chain had been reached, indicated by a standard deviation of split frequencies of 5·10−3, absence of a trend in the probabilities plotted against the generations, and by a Potential Scale Reduction Factor (Gelman and Rubin, Reference Gelman and Rubin1992) converging towards 1), while sampling the Markov chain at a frequency of 100 generations. We discarded 25% of the samples as ‘burn-in’. Files containing alignments were converted using ALTER (Glez-Peña et al. Reference Glez-Peña, Gómez-Blanco, Reboiro-Jato, Fdez-Riverola and Posada2010).
RESULTS
Species descriptions
Gyrodactylus sturmbaueri n. sp. (Fig. 1 left; Fig. 2 (A, B, H); Table 2)
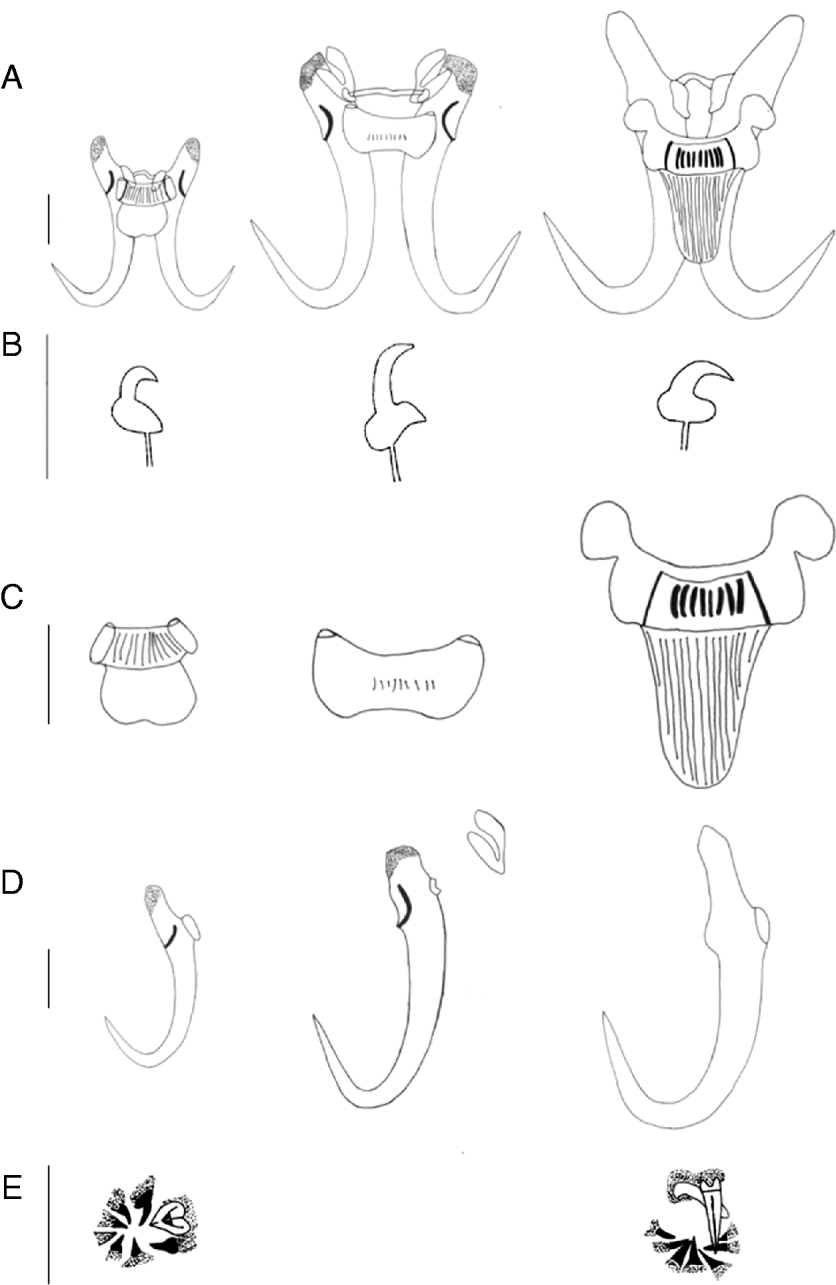
Fig. 1. Hard parts of Gyrodactylus sturmbaueri n. sp. (left), G. thysi n. sp. (middle) and G. zimbae (right). (A) Overview; (B) marginal hook sickle; (C) ventral bar; (D) hamulus; (E) MCO spines (not observed in G. thysi n. sp.). All scale bars=20 μm.

Fig. 2. Hard parts pictures of Gyrodactylus sturmbaueri n. sp. (A) Hamuli and bars; (B) marginal hooks; (H) MCO G. thysi n. sp.; (C) hamuli, bars and additional structures; (D) marginal hooks; (E) detail of detached additional structures and G. zimbae n. sp.; (F) marginal hooks; (G) hamuli and ventral bar; (I) MCO. All scale bars=20 μm.
Table 2. Comparison of morphological body and haptor measurements (in μm, average±standard deviation with range in parentheses) of Gyrodactylus sturmbaueri n. sp., G. thysi n. sp. and G. zimbae n. sp.

a n=23;
b n=28;
c n=27;
d n=29;
e n=30;
f n=31;
g n=1;
h n=7;
i n=11;
j n=6;
k n=15;
l n=5;
m n=2;
n n=4.
Type-host: Simochromis diagramma (Günther, 1894).
Site of infection: gills, possibly fins or skin. Most specimens were attached to the gill filaments, but as some individuals were found detached in the preservation ethanol, we cannot exclude other infection sites.
Type-locality: Kalambo Lodge, Lake Tanganyika, Zambia (S 8°37′, E 31°12′).
Studied material: 32 mounted individuals were measured; of 5 of those, the anterior end of the body was used for molecular analysis. This, and partial proteolytic digestion of some of the mounted individuals, prevented measurements on the entire body in some specimens. Five additional specimens were sequenced.
Type-material: the holotype is deposited at the Natural History Museum, London, U.K. (2010.9.15.2). Paratypes are deposited at the Royal Museum for Central Africa, Tervuren, Belgium (37671) and at the South African Museum, Cape Town, Republic of South Africa (SAMCTA-29493).
Sequence data: a fragment of 900 bp was amplified and sequenced, containing the last 42 bp of the 18S rDNA gene, the ITS-1 (339 bp), the 5·8S rDNA gene (157 bp), the ITS-2 (303 bp) and the first 59 bp of the 28S rDNA gene. Three sites in ITS-2 were polymorphic. Sequences were deposited in GenBank (Accession numbers HQ214477-HQ214480).
Etymology: named after Professor Dr Christian Sturmbauer (Austria), specialist in the evolution of Tanganyika cichlids, and team leader of the expedition during which the host fish were caught.
Diagnosis (average (range), in micrometer): Cover-slip-flattened specimens with 2 anterior lobes, 455·9 (380·4–570·6) long and 216·8 (150·6–302·0) wide. MCO armed with a large apical spine of length 7·4 (6·1–9·2) and 7 smaller spines arranged in one row. One ‘medial’ spine of length 5·7 (4·3–6·7) and 2 ‘subterminal’ spines 6·1 (5·2–7·3) long, are more slender than the two 6·5 (5·2–8·3) long ‘terminal’ ones (terminology of García-Vásquez et al. Reference García-Vásquez, Hansen and Shinn2007) and than 2 spines positioned closer to the apical spine, with a length of 6·3 (4·8–8·2). Hamulus slender, 58·8 (55·8–63·9) long, with aperture 31·0 (29·0–33·3) and point of length 23·5 (20·9–29·3). Hamulus inner curve length is 1·6 (0·7–2·8). Hamulus shaft 43·0 (39·1–45·5) long, with a width of 9·6 (7·1–11·0) at the proximal and 5·5 (4·5–7·0) at the distal end. Root of hamulus 17·0 (14·5–19·9) long. Ventral bar 20·0 (16·3–23·6) long and 21·3 (18·0–23·9) wide in total. Median portion clearly striated, with a length of 8·4 (6·9–10·3). Ventral bar membrane 10·2 (7·1–13·6) long, two-lobed. Ventral bar processes small and stubby, 1·4 (1·0–2·0) long, process to mid-length 1·5 (0·9–2·3). Dorsal bar simple, 22·6 (16·4–32·5) long and 1·5 (1·2–1·9) wide. Marginal hooks with a total length of 39·0 (33·2–51·0) and a shaft length of 30·3 (24·8–42·7). Sickle of marginal hook 8·5 (7·0–10·1) long, with a proximal width of 6·7 (4·7–8·0) and a distal width of 4·6 (3·1–5·7). The foot of the marginal hook sickle comprises a considerable part of the total sickle length; toe 2·8 (2·3–3·6) long, with tip extending beyond sickle point. Marginal hook aperture 7·6 (6·2–8·8).
Gyrodactylus thysi n. sp. (Fig. 1 middle; Fig. 2 (C, D, E); Table 2)
Type-host: Simochromis diagramma (Günther, 1894).
Additional host: Simochromis cfr. diagramma (Günther, 1894).
Site of infection: probably fins or skin. All specimens were retrieved from the preservation ethanol, and none was found attached to the gills, making a location at the outer surface of the fish most likely.
Type-locality: Kalambo Lodge, Lake Tanganyika, Zambia (S 8°37′, E 31°12′).
Studied material: 5 mounted individuals were measured; the anterior end of the body of 2 of these was used for molecular analysis. This, and partial proteolytic digestion of 4 of the mounted individuals, allowed measurements of body size in only a single specimen.
Type-material: the holotype is deposited at the Natural History Museum, London, U.K. (2010.9.15.3). Paratypes (partially digested) are deposited at the Royal Museum for Central Africa, Tervuren, Belgium (37670) and at the South African Museum, Cape Town, Republic of South Africa (SAMCTA-29494).
Sequence data: a fragment of 898 bp was amplified and sequenced, containing the last 42 bp of the 18S rDNA gene, the ITS-1 (291 bp), the 5·8S rDNA gene (158 bp), the ITS-2 (348 bp) and the first 59 bp of the 28S rDNA. Both sequences were identical; the sequence was deposited in GenBank (Accession number HQ214481).
Etymology: named after Professor Emeritus Dirk F.E. Thys van den Audenaerde (Belgium), eminent researcher of African fish, and honorary director of the Royal Museum for Central Africa, for his promotion of ichthyological research in Africa.
Diagnosis (average (range), in micrometer): Cover-slip-flattened specimen 472·6 long and 107·7 wide. MCO not observed. Hamulus elongate, 92·9 (90·5–94·6) long, with aperture 43·3 (39·3–52·7) and point of length 43·4 (41·7–44·5). Hamulus inner curve length 5·7 (4·0–7·4). Hamulus shaft 71·1 (67·3–73·3) long, with a width of 17·1 (16·3–18·7) at the proximal and 8·1 (7·2–9·9) at the distal end. Root of hamulus reduced, 18·5 (17·6–20·2) long. A somewhat U-shaped structure covers the dorsal bar attachment points and part of the hamulus root. Ventral bar 17·9 (15·5–21·7) long and 38·0 (27·9–46·3) wide, with a somewhat halter-shaped median portion of 12·3 (10·7–14·9) long. Ventral bar membrane not observed. Ventral bar processes relatively small and blunt, 2·6 (1·9–3·5) long, process to mid-length 4·7 (3·4–6·7). Dorsal bar 35·7 (32·3–40·9) long. The central part of the dorsal bar 2·7 (2·5–2·8) wide, relatively broad as compared to the attachment zones to the hamulus. Marginal hooks elongate, 46·6 (40·6–49·6) long with a shaft length of 32·8 (26·5–36·6). Outwards pointing sickle of marginal hook 11·6 (10·7–13·0) long, with a proximal width of 8·3 (7·5–9·0) and a distal width of 6·5 (5·8–7·9). Curved toe of marginal hook sickle 5·4 (4·2–6·6) long. Marginal hook aperture 13·4 (11·9–14·3).
Remarks: Some metrics from the 2 sequenced individuals are quite different. Since their sequences are identical, we treat them as the same species. The fact that we did not observe a ventral membrane is very likely an artifact of proteolytic digestion; we cannot confirm its absence based on the limited number of mounts in this study. The pair of U-shaped structures at the base of the hamuli has never been recorded before in any other Gyrodactylus species.
Gyrodactylus zimbae n. sp. (Fig. 1 right; Fig. 2 (F, G, I); Table 2)
Type-host: Simochromis diagramma (Günther, 1894).
Additional hosts: Simochromis cfr. diagramma (Günther, 1894), Ctenochromis horei (Günther, 1894).
Site of infection: mainly fins. Most studied specimens were either attached to the fins (pectoral, caudal and anal) or retrieved from the preservation ethanol. The single specimen on Ctenochromis horei was found on the filaments of the second gill arch.
Type-locality: Kalambo Lodge, Lake Tanganyika, Zambia (S 8°37′, E 31°12′).
Studied material: 12 mounted individuals were measured; of 2 of those, the anterior end of the body was used for molecular analysis. This, and partial proteolytic digestion of some of the mounted individuals prevented measurements of body size on some specimens. Five additional specimens were sequenced. Their pictures were taken before DNA extraction, allowing post-factum measurements to ensure their position within the morphometric range of Gyrodactylus zimbae. Those measurements were not included in the descriptive statistics.
Type-material: the holotype is deposited at the Natural History Museum, London, U.K. (2010.9.15.4) Paratypes are deposited at the Royal Museum for Central Africa, Tervuren, Belgium (37672), and at the South African Museum, Cape Town, Republic of South Africa (SAMCTA-29495).
Sequence data: a fragment of 1000 bp was amplified and sequenced, containing the last 42 bp of the 18S rDNA, the ITS-1 (372 bp), the 5·8S rDNA gene (158 bp), the ITS-2 (369 bp) and the first 59 bp of the 28S rDNA. All sequences were identical; the sequence was deposited in GenBank (Accession number HQ214482).
Etymology: named after Justina Kasabila Zimba (Zambia), research officer in charge of the Lake Tanganyika Research Station in Mpulungu, for her contributions at the field laboratory during the sampling of the fish and their parasites.
Diagnosis (average (range), in micrometer): Two anterior lobes. Considerable body size with a length of 1208·8 (610·2–2342·1) and width of 440·5 (201·8–771·3). MCO with a large apical spine of length 14·6 (13·6–16·8) with broad base, and seemingly associated with a blunt spine of comparable size range 13·1 (12·9–13·3). One row of 7 smaller MCO spines, each similarly thick, of length 6·2 (5·2–7·5) (two ‘terminal’ pairs), 6·3 (5·2–7·2) (‘subterminal’ pair) and 6·0 (5·5–6·8) (‘medial’ spine) (terminology based on García-Vásquez et al. Reference García-Vásquez, Hansen and Shinn2007). Hamulus firm, 105·1 (99·8–111·7) long, with aperture 43·9 (40·4–46·4) and point of 46·9 (43·6–49·3) long. Hamulus inner curve length 2·7 (1·2–4·7). Hamulus shaft 71·9 (70·3–74·8) long, with a width of 19·5 (17·1–21·7) at the proximal and 11·0 (9·2–13·7) at the distal end. Root of hamulus 35·3 (32·9–39·7) long. Ventral bar 49·9 (38·8–55·7) long and 58·8 (53·0–64·6) wide in total, with a median length of 12·5 (10·7–15·0) and an elongate membrane of length 33·7 (29·0–35·9). Both ventral bar and membrane with clear striae. Ear-shaped ventral bar processes 10·4 (9·0–12·0) long, processes to mid-length 13·3 (9·0–15·1). Dorsal bar 23·3 (19·7–29·1) long and 1·7 (1·4–2·0) wide, attachment zones to hamulus of irregular shape. Marginal hooks with a total length of 61·0 (58·2–67·0) and a shaft of 51·9 (48·5–58·6) long. Sickle of marginal hook 9·4 (8·6–10·7) long, with a proximal width of 8·9 (7·8–9·8) and a distal width of 7·9 (7·3–9·2). Sickle point extends beyond toe tip. Toe of marginal hook sickle blunt, 4·8 (4·3–5·1) long. Marginal hook aperture 9·0 (8·2–9·4).
Morphometric analysis
In addition to the Gyrodactylus spp. found on Simochromis diagramma and S. cfr. diagramma, we recorded 4 other congeners in Lake Tanganyika (Table 4). The specimen on Ctenochromis horei was identified as Gyrodactylus zimbae n. sp. from genetic data, confirming its position in a PCA plot (Fig. 3, visualizing the resemblance of the specimens found on other host species to the species described in this study). The specimens on Cyathopharynx furcifer (Boulenger, 1898) and Lobochilotes labiatus (Boulenger, 1898) strongly resemble Gyrodactylus zimbae n. sp. (Fig. 3). None of those specimens was included in the species diagnosis above, since we cannot assess with certainty whether they fall within the normal size range of the species under study, display phenotypic plasticity as adaptation to a different host, or represent a new species. Unfortunately, no material of these specimens was available for molecular analysis. The parasite infecting the gills of Tropheus moorii Boulenger, 1898 clusters close to Gyrodactylus sturmbaueri, but clear qualitative differences (e.g. relatively longer hamulus root) were observed, leading us to conclude that it belongs to a different species.

Fig. 3. Plot of principal component analysis (horizontal axis: PC 1, vertical axis: PC 2) on measurements of 32 Gyrodactylus sturmbaueri n. sp., 5 G. thysi n. sp. and 12 G. zimbae n. sp. on the type-hosts, including 4 Gyrodactylus spp. on other host species. Body size, marginal hook total length, shaft length, and dorsal bar length were not included in the PCA.
Molecular and phylogenetic analysis
BLAST searches came up with 2 close matches spanning the complete ITS-1, 5·8S and ITS-2 region, for G. sturmbaueri n. sp., displaying 92% overall identity to G. ergensi and 91% to G. cichlidarum. These are the only sequenced Gyrodactylus species from cichlid hosts and from African freshwater fish. Genetic distances (based on 432 bp of 5·8S rDNA and ITS-2) between all species in the analysis are shown in Table 3. The distance between G. sturmbaueri n. sp. (and its 2 close relatives) and G. thysi n. sp. is similar to the distance to African gyrodactylid genera Macrogyrodactylus and Gyrdicotylus, to most of the other Gyrodactylus spp., and to Fundulotrema (namely 30–41%). The genetic distance to G. zimbae n. sp. is 41–45%. The difference between the 3 new species and the other genera ranges from 41 to 49% for Diplogyrodactylus martini and is above 45% for Gyrodactyloides and Acanthoplacatus.
Table 3. Gamma-corrected pairwise genetic distances (in %) between the taxa included in the phylogenetic analysis, for a fragment of 432 bp (5·8S rDNA and partial ITS-2)

Table 4. Records of Gyrodactylus spp. in Lake Tanganyika on hosts other than Simochromis diagramma or S. cfr. diagramma

A phylogenetic reconstruction is shown in Fig. 4. The 2 known tilapiine parasites firmly group together with G. sturmbaueri n. sp. in all analyses. The sequence of G. zimbae n. sp. seems to be more distinct, as it shows a higher similarity to that of various non-African representatives than the other African species, especially in ITS-2. It seems to cluster with species of G. (Paranephrotus), G. (Mesonephrotus) and G. (Metanephrotus), as well as Fundulotrema foxi and the South American Gyrodactylus spp. The other African species, G. eyipayipi, appears to be most closely related to members of the rugiensis group of G. (Paranephrotus) and G. (Neonephrotus), which in turn clusters together with G. (Limnonephrotus), confirming the findings of Huyse et al. (Reference Huyse, Audenaert and Volckaert2003) and Ziętara and Lumme (Reference Ziętara and Lumme2004). Acanthoplacatus sp. is basal to this clade. The representatives of G. (Gyrodactylus) cluster together, with strong support, but the position of this subgenus and that of Gyrodactyloides bychowskii vary according to the method of phylogenic reconstruction, and remained unresolved.

Fig. 4. Phylogram constructed from 5·8S rDNA (157 bp) and partial ITS-2 sequences (275 bp) for gyrodactylid genera, and subgenera and selected species of Gyrodactylus. Statistical node support is consequently shown as follows: Bayesian posterior probability/maximum likelihood bootstrap/maximum parsimony bootstrap. Branch lengths correspond to the expected number of substitutions per site under Bayesian inference.
DISCUSSION
Although the African Great Lakes are acknowledged hotspots of biodiversity, especially because of the well-studied cichlid species flocks (Galis and Metz, Reference Galis and Metz1998; Snoeks, Reference Snoeks2000), they have been almost entirely neglected in parasite research. Among all African cichlids, 69 Cichlidogyrus species have been described, but only 5 Gyrodactylus spp. (Christison et al. Reference Christison, Shinn and van As2005; Přikrylová et al. Reference Přikrylová, Matĕjusová, Musilová and Gelnar2009a). Apart from studies on a few Digenea, Cestoda and Acanthocephala (Prudhoe, Reference Prudhoe1951), the helminths of Lake Tanganyika have been greatly overlooked or ignored. The 3 species descriptions represent the first monogenean records from Lake Tanganyika.
Comparisons with other Gyrodactylus spp
Based on molecular data, G. sturmbaueri n. sp. appears relatively closely related to G. cichlidarum from Oreochromis niloticus and to G. ergensi from Sarotherodon galilaeus and O. niloticus. Even though these are the only available sequences of African species so far, an overall identity of over 90% in the ITS rDNA region, and an identical 5·8S rDNA gene, do suggest a very close evolutionary relationship between the 3 species. Morphologically, however, they are quite distinct: G. cichlidarum displays slightly smaller haptoral elements, a more slender hamulus, a more curved ventral bar, and a more pointed marginal hook sickle, with the point extending beyond the toe. G. sturmbaueri n. sp. is also distinct from G. ergensi, for instance, in the longer and more slender hamulus and the shorter marginal hook shafts of the latter species. The number of small MCO spines also differs between the 3 species (7 in G. sturmbaueri n. sp. versus 6 in G. cichlidarum and 5 in G. ergensi). G. zimbae n. sp. seems relatively large in comparison to the known African Gyrodactylus fauna, as it is twice as large as most species described so far (Christison et al. Reference Christison, Shinn and van As2005). The presence of a blunt, spine-like structure whose basis is associated with the large apical spine (it was observed both next to and partly under the other large spine, possibly as an artifact of flattening) is not known from other gyrodactylids.
The haptor morphology of G. thysi n. sp. is quite similar to G. thlapi described on Pseudocrenilabrus philander philander. This is especially true for the elongated marginal hook with a broad, poorly angling point, a curved foot with pointed toe, a long, rounded heel and the position of the origin of the marginal hook shaft. The simple dorsal bar with relatively small attachment zones and the shape of the ventral bar remind of G. thlapi. The dimensions of G. thlapi are, however, smaller for all haptoral elements. An interesting feature is the reduced hamulus root. Christison et al. (Reference Christison, Shinn and van As2005) stated that, although the influence of this reduction in root length on attachment to the host surface is unknown, it may be compensated for by the longer attachment planes of the ventral bar, allowing sufficient rotation of the hamuli over the ventral bar (see Shinn et al. (Reference Shinn, Bron, Sommerville and Gibson2003) for the mechanism of attachment and the role of the different haptoral elements). Although still a matter of speculation, the U-shaped structures we observed near the hamulus roots of G. thysi might also have a role in compensating for the short root, perhaps in association with the muscular retraction of the hamuli towards the body of the worm. In one of our mounts, the haptor became detached from the body, while the U-shaped structures remained attached to the body. This might suggest a function in the interplay between haptoral parts and body (musculature). In any case, additional haptoral elements of such shape have not been recorded before, though other Gyrodactylus spp. seem to have structures associated with the hamulus root (Pugachev et al. Reference Pugachev, Gerasev, Gussev, Ergens and Khotenowsky2009). More species descriptions and molecular data are needed to clarify the interrelationships with other species, and to establish whether the observed similarities represent true relationships or parallel evolution.
Phylogenetic positioning within the gyrodactylid family
Genetic distances suggest that species currently assigned to Gyrodactylus differ as much from each other as from other gyrodactylid genera. Together with the phylogenetic reconstructions, this supports the view of Kritsky and Boeger (Reference Kritsky, Boeger, Combes and Jourdane2003) that Gyrodactylus is not a natural grouping. Representatives of Acanthoplacatus, Gyrdicotylus, Gyrodactyloides and Fundulotrema cluster within Gyrodactylus spp. both in our analyses and in the study of Kritsky and Boeger (Reference Kritsky, Boeger, Combes and Jourdane2003). However, the ITS region is not ideal for phylogenetic analysis at this level, because of its apparent rapid evolution (Hillis et al. Reference Hillis, Mable, Moritz, Hillis, Moritz and Mable1996). Therefore, artificial groupings due to long-branch attraction cannot be excluded.
The position of G. thysi n. sp. is not well supported in the 5·8S rDNA+ITS-2 analysis, but, based on the pairwise genetic distances, it is most closely related to Macrogyrodactylus. The limited amount of data needs to be considered, but it is noteworthy that the aforementioned genus is characterized by additional haptoral parts, as is G. thysi n. sp. Other genera considered to be related to Macrogyrodactylus on the basis of the haptoral arrangement have recently been described both in Africa (Mormyrogyrodactylus) and America (Scutalatus Vianna, Boeger and Dove, Reference Vianna, Boeger and Dove2007) (Luus-Powell et al. Reference Luus-Powell, Mashego and Khalil2003; Vianna et al. Reference Vianna, Boeger and Dove2007). It would be interesting to investigate their relationship with less rapidly evolving markers and to test the monophyly of Gyrodactylus. Indeed, to cite Kritsky and Boeger (Reference Kritsky, Boeger, Combes and Jourdane2003), this genus as used to date could be a ‘catch-all’ taxon for species lacking distinctive morphological traits.
The position of the G. sturmbaueri n. sp., G. ergensi and G. cichlidarum clade is not well resolved, but they cluster firmly, and share an identical 5·8S sequence. They could belong to 1 subgenus, as this gene is believed to be useful to distinguish among the 6 subgenera of Gyrodactylus that were described by Malmberg (Reference Malmberg1970) based on the excretory system (Ziętara et al. Reference Ziętara, Huyse, Lumme and Volckaert2002; Huyse et al. Reference Huyse, Audenaert and Volckaert2003), and is also used by García-Vásquez et al. (Reference García-Vásquez, Hansen and Shinn2007) for the subgenus affiliation of G. cichlidarum. Morphological analysis of soft parts is paramount to formally investigate whether raising this clade to subgenus level would be justified. The most divergent new species is G. zimbae. It clusters, mainly supported by BI, together with members of a diverse clade identified by Ziętara and Lumme (Reference Ziętara and Lumme2004). In contrast to the study of Ziętara and Lumme (Reference Ziętara and Lumme2004), but corroborating Kritsky and Boeger (Reference Kritsky, Boeger, Combes and Jourdane2003), the South American Gyrodactylus spp. are also related to this group, as is Fundulotrema. Hence, the geographical and ecological diversity of this clade could be larger than already put forward by Ziętara and Lumme (Reference Ziętara and Lumme2004). No affinities where shown between the 3 Tanganyika species and G. eyipayipi, the only African marine species known so far (Vaughan et al. Reference Vaughan, Christison, Hansen and Shinn2010). This species clustered closely with members of the rugiensis-group which infects North Atlantic gobies.
The origin of the Gyrodactylus species on Simochromis cichlids
The large genetic distances between the 3 new Gyrodactylus species (in comparison to species sharing 1 host in the studies of Matĕjusová et al. Reference Matĕjusová, Gelnar, McBeath, Collins and Cunningham2001; Ziętara and Lumme, Reference Ziętara and Lumme2002; Huyse et al. Reference Huyse, Audenaert and Volckaert2003), as well as the phylogenetic reconstructions, suggest that they belong to distinct lineages within the genus Gyrodactylus, and that they cannot have diverged within their present type-host. Moreover, applying a molecular clock for Gyrodactylus of 5·5%.my−1 (Ziętara and Lumme, Reference Ziętara and Lumme2002), the origin of the 3 Gyrodactylus spp. has been dated at more than 4 MYA (based on the highest distance in the 5·8S rDNA + ITS-2 fragment, but this could be an underestimation due to our inability to align the entire ITS region). Consequently, it predates the split between the Tropheini and the other ‘modern haplochromines’, which was dated at 2.8 million years ago (Koblmüller et al. Reference Koblmüller, Egger, Sturmbauer and Sefc2010).
How can the occurrence of such highly distinct Gyrodactylus lineages on a single host be explained? One of the following scenarios or a combination of them can be put forward.
(1) Lake Tanganyika as an evolutionary reservoir for ancient lineages
Due to the lake's depth and stability, it harbours several lineages of thalassoid gastropods that became extinct elsewhere in Africa, serving as a reservoir for subsequent colonizations (Wilson et al. Reference Wilson, Glaubrecht and Meyer2004). This function also holds for the major cichlid lineages (Nishida, Reference Nishida1991; Salzburger et al. Reference Salzburger, Meyer, Baric, Verheyen and Sturmbauer2002) and therefore for Monogenea possibly too.
(2) Host migration and parasite exchange within Lake Tanganyika
The possibility of viviparous Gyrodactylidae to change hosts as adults (Cable et al. Reference Cable, Scott, Tinsley and Harris2002) is an important trigger for diversification (Boeger et al. Reference Boeger, Kritsky and Pie2003) and a common, if not their most important, mode of speciation (Harris, Reference Harris1993; Ziętara and Lumme, Reference Ziętara and Lumme2002). Due to an increased chance of parasite encounters, host geographical range and vagility has been suggested to be positively correlated with parasite species richness (Gregory, Reference Gregory1990; Mwita and Nkwengulila, Reference Mwita and Nkwengulila2008). Because of the high mobility of Simochromis and given their limited level of stenotopy, such a phenomenon seems possible for Simochromis hosts as well. This could also explain the near-absence of geographical races in Simochromis (Brichard, Reference Brichard1978; Meyer et al. Reference Meyer, Knowles and Verheyen1996; Konings, Reference Konings1998). However, due to a complete lack of other Gyrodactylus material from the Tanganyika basin, it is at present impossible to hypothesize whether such host-switches took place in the present-day Simochromis species, or in ancestral lineages.
(3) Host migration and parasite exchange with riverine species
The Gyrodactylus diversity on Simochromis might also originate to some extent from colonizations from outside the lake, as is the case in Tanganyika crustacean fish parasites, for which multiple invasions into the lake are probable (Coulter, Reference Coulter and Coulter1991a). It is interesting to remember the morphological resemblances between G. thysi n. sp. and the Pseudocrenilabrus philander parasite G. thlapi that occurs in the Okavango Delta, Botswana. Fish dispersal pathways must have existed at some point in time between African waterways in general and the Great Lakes in particular (Greenwood, Reference Greenwood, Sims, Price and Whalley1983; Salzburger et al. Reference Salzburger, Mack, Verheyen and Meyer2005). Moreover, Tropheini are derived from a generalist riverine ancestor, and Pseudocrenilabrus is ancestral to the Tropheini and the modern Haplochromini (Salzburger et al. Reference Salzburger, Mack, Verheyen and Meyer2005). It would be interesting to investigate, with molecular data, the influence of phylogenetic or ecological affinities of the hosts on the evolution of their parasites (as Barson et al. (Reference Barson, Přikrylová, Vanhove and Huyse2010) did for Macrogyrodactylus).
Host switching might also explain the close relation between G. sturmbaueri n. sp. and G. ergensi and G. cichlidarum, parasites of various Tilapiini occurring in the Levant, North, West and West-Central Africa (Trewavas, Reference Trewavas1983). The typical Simochromis habitat is the rocky littoral, but it is also abundant in the shallow muddy habitats, often associated with nearby river estuaries. It shares these habitats, and its herbivorous substrate grazing lifestyle, with Oreochromis tanganicae (Günther, 1894). This is the only Oreochromis in the lake proper, though congeners like O. karomo (Poll, 1948) and O. niloticus also occur in the Tanganyika basin (Brichard, Reference Brichard1978; Konings, Reference Konings1998). Based on the limited data available, it is impossible, however, to infer or reconstruct host-switching pathways.
Host and site specificity
Our sampling strategy did not allow a clear distinction between fin and gill parasites, but based on haptor morphology we suspect a difference in infection sites: fins for Gyrodactylus zimbae n. sp. and G. thysi n. sp., and gills for G. sturmbaueri n. sp. A smaller haptor is characteristic for gill species (Malmberg, Reference Malmberg1970). It is interesting that G. zimbae n. sp. was found on the gills of a non-Simochromis host, namely Ctenochromis horei. We might be observing an accidental host and site-switch, and as a wide range of host specificity exists within Gyrodactylus (Bakke et al. Reference Bakke, Harris and Cable2002, Reference Bakke, Cable and Harris2007), niche change may happen relatively quickly between closely related Gyrodactylus species (Huyse et al. Reference Huyse, Audenaert and Volckaert2003) and even within a species (e.g. G. arcuatus (Raeymaekers et al. Reference Raeymaekers, Huyse, Maelfait, Hellemans and Volckaert2008)). Although the identification of G. zimbae n. sp. on C. horei was confirmed molecularly and morphologically, the specimens on C. furcifer and Lobochilotes labiatus, resembling G. zimbae n. sp., were not available for molecular analysis. Although Paperna (Reference Paperna1979) stated that some African Gyrodactylus exhibit low host-specificity, we might be dealing with cryptic species, as similar-looking specimens from different hosts have already been shown to be different species (Geets et al. Reference Geets, Appleby and Ollevier1999; Ziętara and Lumme, Reference Ziętara and Lumme2002; Huyse and Malmberg, Reference Huyse and Malmberg2004; Kuusela et al. Reference Kuusela, Ziętara and Lumme2008). Therefore we need molecular confirmation, before inferring a host-switch to the quite distantly related cichlid Cyathopharynx furcifer that belongs to the tribe Ectodini (Poll, Reference Poll1986).
CONCLUSIONS
These are the first records of Monogenea in Lake Tanganyika. The Tropheini cichlid Simochromis diagramma was found to be infected with 3 new Gyrodactylus species, highly distinct in morphology and DNA sequence. Except for genetic similarity between G. sturmbaueri n. sp., G. ergensi and G. cichlidarum, and some morphological resemblance between G. thysi n. sp. and G. thlapi, no close affiliations were found with other Gyrodactylus species. The divergence between the 3 species, of which some display morphological features unknown from the genus, and their position in a molecular phylogeny of Gyrodactylidae, suggests affinities with other genera and the non-monophyly of the genus Gyrodactylus. The reservoir function of Lake Tanganyika for ancient lineages, in combination with ecological transfers from unrelated hosts (within the lake proper, or from or towards riverine systems) could be responsible for this unusual combination of Gyrodactylus species on a single host species. A more specific scenario, however, cannot be inferred based on the present data. Other fish species from Lake Tanganyika and surrounding river systems should be investigated for the presence of Gyrodactylus, in order to compile sufficient data for meaningful comparisons and to infer the phylogenetic relationships of gyrodactylids in Africa.
ACKNOWLEDGEMENTS
Our sincere gratitude goes to T. Veall (Rift Valley Tropicals), J.K. Zimba and L. Makasa (Lake Tanganyika Research Station, Department of Fisheries, Ministry of Agriculture and Co-operatives), C. Katongo (University of Zambia) and their technical staff for assistance with collecting, and for logistic support. C. Sturmbauer, staff and students (especially T. Wurzinger and P. Schober for the Simochromis cfr. diagramma specimen) of the Karl-Franzens University of Graz are gratefully acknowledged. We thank our colleagues C. Gillardin for the specimens from Tropheus moorii (museum specimen MRAC 95-096-P-3010) and Ctenochromis horei, and J. Raeymaekers for comments and suggestions. Three anonymous referees contributed to improving this study.
FINANCIAL SUPPORT
Fieldwork was funded by a travel grant to M.P.M.V. from the Research Foundation, Flanders (FWO-Vlaanderen) and from the King Leopold III Fund for Nature Exploration and Conservation. M.P.M.V. and T.H. respectively are in receipt of a Ph.D. and a Post-doctoral Fellowship of the Research Foundation, Flanders.