INTRODUCTION
CARS, the Consortium for Anthelmintic Resistance SNPs, has the aim of identifying single nucleotide changes in parasitic nematodes that are associated with anthelmintic resistance. The molecular detection of resistance to benzimidazoles has been possible in some isolates of parasitic nematodes by looking for single nucleotide polymorphisms (SNPs) in β-tubulin genes (von Samson-Himmelstjerna et al. Reference von Samson-Himmelstjerna, Buschbaum, Wirtherle, Pape and Schnieder2003). A phenylalanine-to-tyrosine change at position 200 (F200Y) in the isotype 1 β-tubulin gene (TTC to TAC) has been associated with benzimidazole resistance in some isolates of Haemonchus contortus. We know, however, that not all benzimidazole resistance is associated with this specific change and other identified and unidentified SNPs are associated with this phenotype. The CARS aim, then, is to identify all SNPs associated with resistance to all types of anthelmintic in all significant parasitic nematodes. The major classes of anthelmintic which CARS is interested in are the benzimidazoles, the nicotinic anthelmintics and the avermectins.
In our review, we focus on cholinergic anthelmintics and review the mode of action of levamisole and pyrantel and use the C. elegans model to comment on possible mechanisms of resistance. We suggest that some forms of resistance are polygenic and resistance SNPs will be difficult to find; however, other forms of resistance might be related to E153G mutations in UNC-38 and Q57G mutations in UNC-63, possibilities which should be tested (Rayes et al. Reference Rayes, De Rosa, Bartos and Bouzat2004; Bartos, Rayes and Bouzat, Reference Bartos, Rayes and Bouzat2006).
We start with a car metaphor (Fig. 1); consider a Dodge Neon and its engine and Ascaris suum. The response of the engine to the ignition of fuel is affected by many factors, including the setting of the carburetor, the timing of ignition, and the timing of the opening of the fuel inlet and exhaust valves. In the same way, the contractile response of Ascaris muscle to levamisole or pyrantel is affected by the molecular settings of components of the muscle receptor signalling-contraction machinery. The point of the metaphor is that the response of a nematode to a cholinergic anthelmintic like levamisole or pyrantel depends on the settings of a large number of interconnecting components, as does the performance of the internal combustion engine. The response of the parasite will depend on the sensitivity of nicotinic acetylcholine (ACh) receptors (nAChRs) on muscle, and on how much depolarization and entry of Ca2+ levamisole/pyrantel produces, together with the sensitivity of the contractile proteins to Ca2+ (Fig. 2). If the muscle contraction response to the cholinergic anthelmintic is sufficiently reduced by any of a number of different components in the receptor signal-transduction pathway, the parasite will show reduced sensitivity (resistance) to the drug.

Fig. 1. CARs and a car metaphor for resistance. CARS, the Consortium seeking Anthelmintic Resistance Single nucleotide polymorphisms, and a car (Dodge Neon and engine). The engine of a car is a complex machine with many parts that can be adjusted to affect the performance of the engine in response to combustion of the fuel. Likewise, a nematode parasite like Ascaris suum has many component proteins that are responsible for the response to the cholinergic anthelmintics, levamisole and pyrantel.
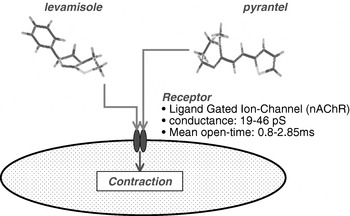
Fig. 2. A simplified but now out-dated model of the mode of action of levamisole and pyrantel. These two compounds act on nicotinic acetylcholine receptors of muscle to gate these channels open, to allow entry of calcium and to produce contraction and spastic paralysis.
USE OF LEVAMISOLE, PYRANTEL AND RELATED COMPOUNDS AGAINST PARASITIC NEMATODES
Pyrantel and levamisole are used for the control of hookworms and roundworms of humans, livestock and companion animals. They are used for treatment of animal and human faecally-transmitted parasitic nematodes, but they are used less often than the benzimidazoles or the avermectins. These drugs are referred to by the World Health Organization, WHO (2000), as essential drugs for treatment of intestinal nematodes. Levamisole and pyrantel are typically effective against roundworms (ascarids) in companion animals and humans, as well as hookworms (Necator americanus and Ancylostoma duodenale), but are not very effective against whipworms (Trichuris trichiura) (Albonico, Crompton and Savioli, Reference Albonico, Crompton and Savioli1999). The reduced efficacy of pyrantel against whipworms gave rise to the development of oxantel because of the need for a drug with a higher efficacy against Trichuris species. A combination of oxantel and pyrantel can be used and has the advantage of a broader spectrum of action (Albonico et al. Reference Albonico, Bickle, Haji, Ramsan, Khatib, Montresor, Savioli and Taylor2002).
Regrettably, resistance has developed following use of these compounds (Sangster and Dobson, Reference Sangster, Dobson and Lee2002) and is now recognized in parasites of both humans and domesticated animals. Resistance to cholinergic agonists may be produced by four general mechanisms: (1) changes in drug translocation (e.g. increased metabolism or excretion of the drug); (2) changes in receptor numbers (e.g. reduced number of receptors); (3) receptor modification (e.g. change in receptor binding sites due to amino acid substitution); or (4) post-receptor modification (e.g. change in the downstream pathway to contraction). All of these mechanisms could play a role in resistance to levamisole and pyrantel and so it is anticipated that resistance to these drugs is polygenic (Sangster and Dobson, Reference Sangster, Dobson and Lee2002).
GENERAL MODE OF ACTION OF CHOLINERGIC ANTHELMINTICS
Fig. 2 shows the chemical structures of the cholinergic anthelmintics levamisole and pyrantel, together with a model for their mode of action. In 1970, papers introducing the pharmacology of levamisole and pyrantel described effects of these compounds on the body muscle of A. suum (Aceves, Erlij and Martinez-Maranon, Reference Aceves, Erlij and Martinez-Maranon1970; Aubry et al. Reference Aubry, Cowell, Davey and Shevde1970). These authors showed that these compounds were cholinergic agonists that selectively produced depolarization and spastic paralysis of body muscle cells in parasitic nematodes. Electrophysiological responses to these anthelmintics can be observed in muscle cells of the nematode parasites A. suum and Oesophagostomum dentatum (Martin, Reference Martin1982; Pennington and Martin, Reference Pennington and Martin1990; Robertson and Martin, Reference Robertson and Martin1993; Evans and Martin, Reference Evans and Martin1996; Martin et al. Reference Martin, Murray, Robertson, Bjorn and Sangster1998; Robertson, Bjorn and Martin, Reference Robertson, Bjorn and Martin1999; Robertson et al. Reference Robertson, Clark, Burns, Thompson, Geary, Trailovic and Martin2002; Trailovic et al. Reference Trailovic, Clark, Robertson and Martin2002, Reference Trailovic, Robertson, Clark and Martin2005; Levandoski et al. Reference Levandoski, Robertson, Kuiper, Qian and Martin2005). These studies identified nAChRs over the nematode muscle cell surface that respond to these anthelmintics and have described, down to the single-channel level, the agonist action and channel-blocking effect of the anthelmintics.
Levamisole opens ACh-gated cation-selective ion-channels, which constitute the nAChRs, on nematode muscle membranes, producing depolarization and muscle contraction (Fig. 2). The ion-channels have conductances of 19–46 pS and mean open-times of 0·5–2·0 ms. Nematode muscle nAChRs have pharmacological and biophysical properties similar to those of vertebrate neuronal receptor channels, but there are also significant pharmacological differences. The pharmacological differences allow levamisole and pyrantel to have a selective therapeutic effect on the nematode muscle receptors, minimizing toxic effects on mammalian (host) muscle.
LEVAMISOLE AND PYRANTEL ACTIVATE N-, L- AND B-nAChR SUBTYPES
We have used muscle strips from the parasitic nematode A. suum (Fig. 3) for pharmacological experiments. We have obtained cumulative dose-response plots for a number of cholinergic agonists (Fig. 4) in the presence of competitive antagonists like paraherquamide, 2-desoxoparaherquamide and methyllycaconitine (Zinser et al. Reference Zinser, Wolf, Alexander-Bowman, Thomas, Davis, Groppi, Lee, Thompson and Geary2002; Robertson et al. Reference Robertson, Clark, Burns, Thompson, Geary, Trailovic and Martin2002). We have been able to identify three pharmacologically distinct nAChRs (Fig. 5) that are preferentially activated by the different cholinergic anthelmintics. These include: (1) the N-subtype, preferentially activated by methyridine and nicotine; (2) the L-subtype, preferentially activated by levamisole and pyrantel and antagonized by paraherquamide; and (3) the B-subtype, preferentially activated by bephenium and antagonized by paraherquamide and 2-desoxoparaherquamide.

Fig. 3. Ascaris suum muscle strip preparation cumulative-dose response relationship to a cholinergic anthelmintic. Ascaris suum are collected from the packing plant and a 2 cm half-body flap (muscle strip) is prepared from behind the head region and placed in a tissue bath with an isometric transducer. The nicotinic anthelmintic is added cumulatively, without washout, to produce the concentration-contraction-response.

Fig. 4. Paraherquamide-antagonism of cumulative-contraction response anthelmintic concentration plots for nicotine, levamisole, pyrantel and bephenium. The concentration-response plots were produced in the absence of paraherquamide and in the presence of increasing concentrations of paraherquamide. Note that paraherquamide acts as a competitive antagonist but that nicotine is not well antagonised, while the other anthelmintics are. The difference in the dose-ratios produced by the antagonist shows that the receptors activated by nicotine are not the same as those activated by levamisole and pyrantel.

Fig. 5. Dendrogram showing the similarity for different cholinergic anthelmintics when antagonised by paraherquamide, 2-desoxyparaherquamide and methyllycaconitine. pA 2 values for 3 antagonists are compared using cluster analysis and this separates 3 receptor subtypes in Ascaris muscle. The three types of receptor are: N-, L- and B-types. The N-type is preferentially activated by oxantel, methyridine and nicotine. The L-type is preferentially activated by levamisole and pyrantel. The B-type is preferentially activated by bephenium.
We have also been able to distinguish the three subtypes at the single-channel level (Qian, Martin and Robertson, Reference Qian, Martin and Robertson2006) using cell-attached patch recordings from somatic muscle vesicles (Fig. 6). These recordings have allowed a comparison of the effects of levamisole and bephenium at the single-channel level and the examination of the effects of the novel anthelmintics paraherquamide and 2-desoxoparaherquamide (Zinser et al. Reference Zinser, Wolf, Alexander-Bowman, Thomas, Davis, Groppi, Lee, Thompson and Geary2002) that act as competitive antagonists (Robertson et al. Reference Robertson, Clark, Burns, Thompson, Geary, Trailovic and Martin2002). These observations emphasize the different selectivities of the cholinergic anthelmintics among the subtypes of cholinergic receptor. In levamisole-resistant isolates of O. dentatum (Fig. 7), we have found a reduction in the sensitivity to levamisole in larval migration assays (Fig. 8). This reduction in sensitivity is also observed with pyrantel but not with nicotine and methyridine (Martin et al. Reference Martin, Clark, Trailovic and Robertson2004). When we examined the single-channel properties of levamisole-sensitive and -resistant isolates, we found that the G35 pS channel of O. dentatum (corresponding to the L-subtype of A. suum) was absent from the levamisole-resistant isolates (Robertson et al. Reference Robertson, Bjorn and Martin1999). These observations confirm that there are differences in the nAChR subtype selectivities among the cholinergic anthelmintics and that, at least in some types of levamisole-resistance, there is a loss of the L-subtype (Fig. 9). The experiments also suggest that in some cases, it may be possible to overcome levamisole resistance by using cholinergic agonists (methyridine: N-subtype) with different subtype selectivities or an antagonist (2-desoxoparaherquamide, B-subtype) with selectivity for other nAChR subtypes.

Fig. 6. Single-channel properties of N-, L- and B-types of channel. A: The conductance of the N-subtype channel is ∼25 pS, the L-type is ∼35 pS, and the B-type is ∼45 pS. Representative openings from the same patch at +75 mV. B: Current amplitude histogram of the same patch recording with the 3 separate N-, L- and B-subtypes. C: Summary diagram of the channel properties and agonist and antagonist selectivities of the N-, L- and B-subtypes.
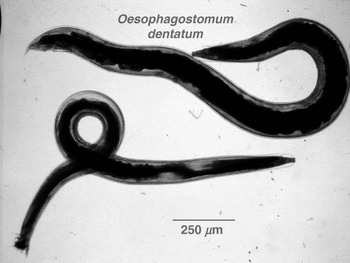
Fig. 7. Unstained photograph of an adult male and female Oesophagostomum dentatum.

Fig. 8. Photograph O. dentatum larvae used in migration assays. Plots: Percent inhibition larval migration assays by levamisole, pyrantel, methyridine and nicotine for levamisole sensitive (SENS) and levamisole resistant (LEV-R) L3 of O. dentatum. There is a significant change in the inhibition curves of the two strains for levamisole and pyrantel, but not nicotine and methyridine.

Fig. 9. Summary diagram showing the loss of the L-subtype of receptors associated with levamisole resistance in Oesophagostomum dentatum.
MUSCLE CONTRACTION RESPONSES TO CHOLINERGIC AGENTS CAN BE MODIFIED
Larval migration assays, muscle contraction assays and electrophysiological experiments have revealed considerable variation in responses in individual animals, even when using the same preparation and the same species of parasite. This variation was initially frustrating until we developed the view that the variation could be physiologically important. If it was better understood, it might be possible to exploit it to increase the size of responses to cholinergic anthelmintics. Neuropeptides are, at least in part, responsible for this variation. Fig. 10 illustrates effects of the neuropeptide AF2 (KHEYLRF-NH2) on Ascaris muscle electrophysiological responses and muscle-contraction responses to ACh (Trailovic et al. Reference Trailovic, Clark, Robertson and Martin2005). Brief exposure to AF2 produces long-lasting potentiation of ACh responses, which are likely to extend to all cholinergic anthelmintics. Thus, other receptors, for example receptors for the neuropeptides, may be responsible for and influence the sensitivity and resistance to cholinergic anthelmintic agents. Changes in expression or properties of an AF2 receptor might be associated with some types of levamisole resistance.

Fig. 10. AF2 potentiation of responses to electrophysiological responses acetylcholine of Ascaris muscle. A: Current-clamp recording of membrane potential and conductance during short pulses of acetylcholine before and after a 2-minute application of AF2. The post-AF2 responses are increased in size. B: Ascaris muscle strip contraction responses to acetylcholine before and after AF2 application.
THE C. ELEGANS MODEL OF THE LEVAMISOLE RECEPTOR, E153 AND Q57
Understanding of the structure and function of nematode AChRs has been advanced by the study of C. elegans, the model soil nematode that has undergone detailed genetic and genomic analysis. Three genes encoding nAChR subunits in C. elegans, associated with strong levamisole resistance, were recognized initially (Fleming et al. Reference Fleming, Squire, Barnes, Tornoe, Matsuda, Ahnn, Fire, Sulston, Barnard, Sattelle and Lewis1997): unc-38, unc-29 and lev-1. Unc-38 encodes α-like subunits, while unc-29 and lev-1 encode non-α subunits. The levamisole-responsive UNC-38 subunit homologues in the parasitic nematodes A. suum (ASAR-1: Access no. AJ011382), Trichostrongylus colubriformis (Wiley et al. Reference Wiley, Weiss, Sangster and Li1996) and Haemonchus contortus (Hoekstra et al. Reference Hoekstra, Visser, Wiley, Weiss, Sangster and Roos1997) are about 90% identical to C. elegans, implying that information derived from the model nematode will be a useful guide for parasitic nematodes. We caution that there are expected to be significant differences between C. elegans and other parasitic nematodes because of the enormous evolutionary distance between some of the species (Blaxter et al. Reference Blaxter, De, Garey, Liu, Scheldeman, Vierstraete, Vanfleteren, Mackey, Dorris, Frisse, Vida and Thomas1998). With the completion of the C. elegans genome, the size of the nematode nAChR gene family has expanded significantly. Analysis by a consortium found that more than 50 predicted open reading frames had substantial similarity to nAChR subunits of other organisms. Transcription of 17 of the predicted nAChR subunit genes has been confirmed and the genes have been designated using acr (acetylcholine receptor) numbers. There are 27 AChR subunit genes (Jones and Sattelle, Reference Jones and Sattelle2004), the largest number of AChR subunit genes in a single species. Homology has allowed these 27 subunits to be divided into five groups named: DEG-3-like; ACR-16-like; ACR-8-like; UNC-38-like; and UNC-29-like. The UNC-29-like subunits are non-α subunits, while the others are mostly α subunits. The possible combinations of α and non-α subunits remains to be determined, but the theoretical possibilities are enormous.
For a variety of developmental and physiological reasons, expression of particular subunits and combinations is restricted to particular nerve cells or muscle cells. UNC-38, UNC-29, UNC-63, LEV-1, ACR-8, ACR 16 and ACR-13 (=LEV-8) GFP-tagged protein subunits have been found in somatic muscle cells of C. elegans (Fleming et al. Reference Fleming, Squire, Barnes, Tornoe, Matsuda, Ahnn, Fire, Sulston, Barnard, Sattelle and Lewis1997; Richmond and Jorgensen, Reference Richmond and Jorgensen1999; Culetto et al. Reference Culetto, Baylis, Richmond, Jones, Fleming, Squire, Lewis and Sattelle2004; Gottschalk et al. Reference Gottschalk, Almedom, Schedletzky, Anderson, Yates and Schafer2005; Towers et al. Reference Towers, Edwards, Richmond and Sattelle2005). Multiple pharmacological AChR subtypes present on somatic muscle of C. elegans are revealed by null mutation experiments and contraction assays. Null mutants of unc-38, unc-29, unc-63 produce very high level resistance to levamisole; a null mutant of acr-13 (lev-8) reduces sensitivity to levamisole; a null mutant of acr-16 confers nicotine resistance with little effect on sensitivity to levamisole; and electrophysiological responses to levamisole but not nicotine are abolished in unc-38, unc-29, unc-63 null mutants and reduced (but not abolished) in lev-1 and acr-13 (lev-8) null mutants (Richmond and Jorgensen, Reference Richmond and Jorgensen1999; Culetto et al. Reference Culetto, Baylis, Richmond, Jones, Fleming, Squire, Lewis and Sattelle2004; Towers et al. Reference Towers, Edwards, Richmond and Sattelle2005); responses to ACh but not levamisole were abolished by acr-16 null mutants, and a phenotype was not observed with acr-8 null mutants (Touroutine et al. Reference Touroutine, Fox, Von Stetina, Burdina, Miller and Richmond2005).
As a minimal model, there are at least two types of nAChR present on the somatic muscle cell of C. elegans: (1) the N-type receptor composed of 5 ACR-16 subunits and most sensitive to nicotine; and (2) the L-type, most sensitive to levamisole (Richmond and Jorgensen, Reference Richmond and Jorgensen1999) and composed of UNC-38, UNC-29, UNC-63, LEV-1 and LEV-8. We have pointed earlier to the presence of N-, L- and B-subtypes of nAChR in A. suum; at this stage, we do not know if there are more than two subtypes of muscle nAChR in C. elegans or if their single-channel properties match those of the parasitic nematodes. We anticipate that there will be similarities as well as significant differences between the C. elegans receptors and those of parasitic nematodes.
Fig. 11 shows a putative structure for the L-type receptor that contains UNC-38, UNC-63 and LEV-8 (ACR-13) α-subunits and UNC-29 and LEV-1 non-α subunits. Information on the ordering of the subunits is unknown. The putative structure is based on the comprehensively studied Torpedo california nAChR (Changeux et al. Reference Changeux, Benoit, Bessis, Cartaud, Devillers-Thiery, Fontaine, Galzi, Klarsfeld, Laufer and Mulle1990). It is assumed (but not tested) that nematode nAChRs are very similar in structure. The Torpedo nAChR is composed of two α- and three non-α subunits (one β-, one γ- and one δ-subunit) arranged in a 5-membered ring around the central pore that forms the transmembrane ion-channel. The pore of the ion-channel is lined by the second transmembrane region (TM2) of each of the 5 subunits. The binding sites of the agonists are formed by 6 loops of amino acids: 3 loops (A, B and C) from the α-subunit and 3 loops (D, E and F) from the adjacent non-α subunit (Corringer et al. Reference Corringer, Bertrand, Bohler, Edelstein, Changeux and Bertrand1998; Brejc et al. Reference Brejc, van Dijk, Klaassen, Schuurmans, van Der, Smit and Sixma2001). The C loop contains two adjacent cysteines that define a subunit as an α-subunit. Since the putative structure of the C. elegans levamisole receptor has 3 α subunits, there are 3 or more non-identical ligand binding sites on each receptor channel: (1) between UNC-38 and an adjacent subunit; (2) between ACR-13 and an adjacent subunit; and (3) between UNC-63 and an adjacent subunit. These non-equivalent sites may bind levamisole with differing affinities. A significant role for UNC-38 is likely, with E153 on loop B being necessary for a selective effect of levamisole (Rayes et al. Reference Rayes, De Rosa, Bartos and Bouzat2004); point mutation of glutamate at position 153 reduces the potency of levamisole. Many point mutations in UNC-38, including mutations in the pore-forming M2 region, may alter the opening of the receptor channel in the presence of levamisole. If equivalent changes were to occur in parasitic nematodes, there would be a loss of sensitivity to levamisole and perhaps pyrantel. This loss in sensitivity to levamisole may be associated with a loss of fitness if there is a concurrent loss of the response to the normal neurotransmitter, ACh.

Fig. 11. Agonist binding sites of the putative levamisole receptor from C. elegans. The subunits are composed of 5 subunitsUNC-38, UNC-63, ACR-13 (LEV-8), UNC-29, and LEV-1. ▸ represents the agonist binding sites formed at the junction between an alpha subunit and an adjacent unit. The agonist binding site between UNC-38 and UNC-63 shows the A, B, C loops of UNC-38 and D, E and F loops of UNC-63. Two amino acids, E153 (Rayes et al. Reference Rayes, De Rosa, Bartos and Bouzat2004) and Q57 (Brown et al. Reference Brown, Jones, Buckingham, Mee and Sattelle2006) are predicted to be required for selectivity of levamisole (E153) and pyrantel (E153 and Q57).
Pyrantel has additional structural requirements (Bartos et al. Reference Bartos, Rayes and Bouzat2006). Pyrantel requires the presence of a glutamine in position 57 on the complimentary subunit binding site (Fig. 11, loop D, UNC 63): pyrantel requires E153 and Q53, but levamisole requires only E153. The different amino-acid requirements for levamisole and pyrantel could explain why O. dentatum and O. quadrispinulatum can acquire resistance to pyrantel but not to Levamisole (Bjorn et al. Reference Bjorn, Roepstorff, Waller and Nansen1990). The lowest sensitivity to levamisole and pyrantel would occur in a parasite nAChR that contained glycine at positions 153 and 57, similar to the mammalian muscle nAChR. Bartos et al. (Reference Bartos, Rayes and Bouzat2006) have suggested that Glu153 may be provided by UNC-38 and Gln57 provided by UNC-63; this combination of subunits would thus lead to a receptor very sensitive to pyrantel.
OTHER C. ELEGANS GENES ARE INVOLVED IN RESISTANCE TO LEVAMISOLE
Forward and reverse mutation experiments in C. elegans starting with Lewis et al. (Reference Lewis, Wu, Berg and Levine1980) have identified 16 genes (Table 1, Fig. 12) that are associated with resistance to levamisole (Lewis et al. Reference Lewis, Wu, Berg and Levine1980, Reference Lewis, Elmer, Skimming, McLafferty, Fleming and McGee1987; Benian, L'Hernault and Morris, Reference Benian, L'Hernault and Morris1993; Kagawa, Takuwa and Sakube, Reference Kagawa, Takuwa and Sakube1997; Fleming et al. Reference Fleming, Squire, Barnes, Tornoe, Matsuda, Ahnn, Fire, Sulston, Barnard, Sattelle and Lewis1997; Culetto et al. Reference Culetto, Baylis, Richmond, Jones, Fleming, Squire, Lewis and Sattelle2004; Gally et al. Reference Gally, Eimer, Richmond and Bessereau2004; Jones and Sattelle, Reference Jones and Sattelle2004; Touroutine et al. Reference Touroutine, Fox, Von Stetina, Burdina, Miller and Richmond2005; Towers et al. Reference Towers, Edwards, Richmond and Sattelle2005; Jones, Buckingham and Satelle, Reference Jones, Buckingham and Sattelle2005; Wormbase, www.wormbase.org). These genes encode nAChR subunits (UNC-38, UNC-29, UNC63, LEV-1 and LEV-8) and interacting proteins, kinases and phosphatases (NRA-1, SOC-1, TAX-6 and TPA-1), the ryanodine receptor (UNC-68), contractile elements (UNC-22 and LEV-11) and levamisole receptor subunit processing proteins (UNC-50, RIC-3 and UNC-74). It seems very likely that other genes will be identified that encode proteins that can be associated with resistance to levamisole in C. elegans.
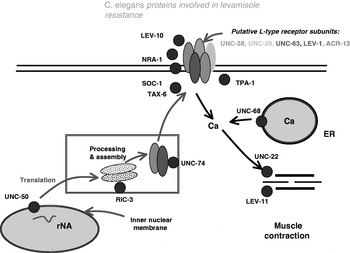
Fig. 12. Summary diagram of the proteins and their functions known to be associated with levamisole resistance in C. elegans. The proteins are involved in the signal-transduction process and expression of levamisole receptors on the muscle membrane surface.
Table 1. C. elegans genes associated with levamisole resistance.

Wormbase 1D and function of proteins encoded by C. elegans genes associated with levamisole resistance.
LIMITATIONS OF THE C. ELEGANS MODEL FOR PARASITIC NEMATODES
The C. elegans model is expected to be a useful, but not complete model for a range of nematode parasites, just as mice are for humans. There are at least two limits to the value of the C. elegans model for parasitic nematodes. (1) Parasitic nematodes have to be able to complete their life-cycle and maintain adequate movement to reproduce and survive in hostile environments like the intestine, and must elude or defend against attack by the host immune system. The repertoire of genetic mutations that may be available to parasitic nematodes for resistance to levamisole and pyrantel is expected to be more limited if the parasite has to retain sufficient fitness to survive in these hostile conditions. Paralysed C. elegans can survive on agar plates seeded with E. coli for food, but parasitic nematodes with compromised motility cannot complete their life-cycles. (2) There is also a difference in the concentration of levamisole used experimentally for C. elegans resistance experiments and therapeutically for parasitic nematodes. Very high concentrations of levamisole, >10−4 M, will produce toxic effects in the mammalian host animal and so cannot be used therapeutically, but concentrations as high as 1 mm are used on agar plates in C. elegans levamisole resistance experiments. Thus, there may be a 10-fold difference in the level of levamisole resistance when considering observations from C. elegans and from parasitic nematodes.
Thus, genes that can produce lower levels of resistance in parasitic nematodes but do not compromise the physiology and movement of the parasitic nematode may be significant clinically but have not yet been revealed by C. elegans experiments. These two different perspectives should be recognized.
Sequencing of parasite genomes has progressed enormously since the lead that was set by C. elegans. The Brugia malayi genome is complete (Ghedin et al. Reference Ghedin, Wang, Foster and Slatko2004) and additional nematode projects are underway at the Genome Sequencing Center, St Louis, USA; the Sanger Institute Hixton, UK; Edinburgh University, Scotland, UK and other sites (see www.nematode.net). As of this writing, some genomic information is available for 43 species of nematodes, including animal, plant and human parasites, and 2,353,560, ESTs from 28 animal parasites. Among these nematodes, 30–70% of the ESTs are nematode specific (Mitreva et al. Reference Mitreva, Blaxter, Bird and McCarter2005), suggesting the possibility that these specific genes could be useful drug targets. Note, however, that levamisole and pyrantel act on drug receptors that are not unique to the nematodes but are present broadly in invertebrates and vertebrates. The small but significant differences in the amino acid sequences of the anthelmintic targets give rise to the unique pharmacology of the drug targets in parasites that have been exploited (benzimidazole anthelmintics have a selective effect on nematode β-tubulins and levamisole has a selective effect on the nAChRs of nematodes). It is also important to point out that 23% of the ESTs observed so far are unique to the nematode species from which they are derived (Mitreva et al. Reference Mitreva, Blaxter, Bird and McCarter2005). This observation, along with the two limitations (life-cycle requirement for parasitic nematodes and limiting concentrations of levamisole), suggests that some of the genes associated with resistance in parasitic nematodes will not be the same as those genes associated with levamisole resistance in C. elegans (Fig. 12, Table 1).
Despite these comments, we remain positive about the value of the C. elegans model for understanding levamisole and pyrantel resistance in parasitic nematodes, particularly as each of the nAChR receptor subunits for the nematode parasite sequences have close homology to one of the C. elegans nAChR subunit families (Brown et al. Reference Brown, Jones, Buckingham, Mee and Sattelle2006). We should expect to find similar principles but different details. The identification of SNPs associated with levamisole resistance is expected to involve a number of genes and a number of alleles of those genes, some of which will be species-specific.
Acknowledgment
Supported by NIH RO1 AI04719406A1.