INTRODUCTION
Malaria, a major problem associated with public health in tropical and subtropical countries has foremost impact on morbidity and mortality. More than 200 million new cases and ∼700 000 deaths due to malaria are reported annually. The disease is posing a great challenge to existing drug therapy (World Malaria Report, 2011).
The current arsenal of conventional antimalarials (chloroquine, mefloquine, QN, QND and pyrimethamine-sulfadoxin) is facing serious threat of discontinuation due to emerging drug resistance (and cross-resistance between closely related drugs), poor compliance and unacceptable toxicity. Chloroquine treatment is now ineffective in most areas of the world. The usual replacement, pyrimethamine-sulfadoxin, is rapidly losing efficacy in Southeast Asia and South America (Barat and Bloland, Reference Barat and Bloland1997). Furthermore, mefloquine has also developed resistance in Thailand, Myanmar and Cambodia and it is recommended in combination (World Health Organization, 2012). In all malaria endemic countries, artemisinin combination therapies (ACT) have become the backbone of treatment of uncomplicated malaria, a policy endorsed by the World Health Organization (2006). But recently, emergence of resistance against widely used artesunate has been confirmed in Pallin (Dondorp et al. Reference Dondorp, Nosten, Yi, Das, Phyo, Tarning, Lwin, Ariey, Hanpithakpong, Lee, Ringwald, Silamut, Imwong, Chotivanich, Lim, Herdman, An, Yeung, Singhasivanon, Day, Lindegardh, Socheat and White2009). The problem is further augmented by the expense and difficulty of maintaining a steady supply of ACT in resource-limited settings, rendering it unaffordable in the developing countries.
QN and QND are the main alkaloids of the bark of South American plant cinchona and have been commonly used as antimalarials for over many years (Achan et al. Reference Achan, Talisuna, Erhart, Yeka, Tibenderana, Baliraine, Rosenthal and D'Alessandro2011). However, resistance against these drugs due to mutation in PfMDR gene of the malaria parasite limits its administration to malaria patients (Wongsrichanalai et al. Reference Wongsrichanalai, Pickard, Wernsdorfer and Meshnick2002). Due to longer duration of treatment, poor patient compliance is another associated problem. To resolve these and to prolong the life of existing drugs it is necessary to devise new combinations based on the PK-PD properties of the partner drugs (Wongsrichanalai et al. Reference Wongsrichanalai, Pickard, Wernsdorfer and Meshnick2002; Sidhu et al. Reference Sidhu, Valderramos and David2005). These two drugs (QN/QND) are metabolized in the liver mainly by CYP3A4 (Ho et al. Reference Ho, Luo, Macauley, Grigor and Wanwimolruk1998; Nielsen et al. Reference Nielsen, Rasmussen, Flinois, Beaune and Brosen1999). During uncomplicated malaria, bioavailability of these antimalarials decreases significantly below a therapeutic level. Therefore it is recommended that in QN resistance areas it should be given in combination with tetracycline to maintain appropriate plasma concentration (Na-Bangchang and Karbwang, Reference Na-Bangchang and Karbwang2009). Tripathi et al. (Reference Tripathi, Pandey and Rizvi2011) also showed that CLTR, being the CYP inhibitor, potentiates the antimalarial properties of mefloquine. The current study evaluates the antimalarial combination of QN/QND with CLTR, an antibiotic of the macrolide family.
MATERIALS AND METHODS
In vitro parasite cultivation
Antimalarial assay was carried out against the CQ sensitive 3D7 clone of P. falciparum procured from NIMR, New-Delhi and maintained in our laboratory for the past 10 years. The parasite culture was maintained in vitro in RPMI-1640 (HEPES modification) medium supplemented with 0·5% AlbuMaxII, 0·2% glucose (w/v), 0·2% NaHCO3 (w/v) and additionally 15 μM hypoxanthine, incubated at 37 °C with 5% CO2, 5% O2 and 90% N2. The medium was changed every 24 h, parasite growth rate and stage was determined by examination of a Giemsa's stained thin smear of the parasitized RBCs.
In vivo parasite maintenance
Outbred Swiss mice of either sex weighing 20–22 g were procured from the animal facilities at the institute, maintained on commercial pellet diet and water ad libitum under standard housing conditions. Ethical guidelines on handling and use of experimental animals were followed during the conduct of the study. The rodent malaria parasite P. yoelii nigeriensis MDR, which is resistant to chloroquine (250 mg/kg/day for 4 days), MFQ (128 mg/kg/day for 4 days) and quinine (400 mg/kg/day for 4 days), was used in the study. These are the maximum tested doses for the particular antimalarials. The parasite was maintained in the animals through sequential passages from the blood of infected mice, obtained by cardiac puncture (Tripathi et al. Reference Tripathi, Pandey and Rizvi2011).
Preparation of drugs
For the assessment of in vitro antimalarial response of QN, QND and CLTR, stock solutions were prepared in DMSO and stored at 0 °C until use. Chloroquine was prepared freshly in milli Q water. For in vivo evaluation, drugs were prepared in Tween 80-water formulation (1:24).
In vitro antimalarial activity of QN, QND and CLTR
Two-fold serial dilutions of drugs (50 μl per well) were prepared in 96-well microtitre plates. Infected erythrocytes (50 μl per well with 4% haematocrit and 1–2% parasitaemia) were added to these wells. Plates were incubated in a CO2 incubator maintained at 37 °C, 5% CO2, 5% O2 and 90% N2. After 72 h of incubation, 100 μl of lytic buffer (20 mM Tris, pH 7·5, 5 mM EDTA, 0·008% saponin, and 0·08% Triton X-100) containing SYBR green dye (2X), were added to each well and incubated for 4 h at room temp in dark. Plates were read under a fluorescence reader at excitation 485 nm, emission 535 nm (Johnson et al. Reference Johnson, Richard, Lucia, Miriam, Norma and Norman2007).
The 50% inhibitory concentration (IC50) was obtained from plots of the percentage inhibition versus concentration of the drug.
Assessment of in vitro antimalarial interaction of QN and QND with CLTR
The in vitro interactions of QN and QND with CLTR were determined according to the method described by Bhattacharya et al. (Reference Bhattacharya, Mishra, Sharma, Awasthi and Bhasin2009) with some modifications. Briefly, 11 combinations of QN and QND with CLTR (1:1, 1:2, 1:3, 1:4, 1:5, 2:1, 3:1, 4:1, 5:1, 2:3, 3:2,) were prepared in 96-well microtitre plates and serially diluted. Asynchronous culture of P. falciparum (1–2% parasitaemia and 4% haematocrit), was exposed for 72 h to these dilutions (37 °C, 5% CO2, 5% O2 and 90% N2). The IC50 values were determined as described earlier. Fractional inhibitory concentration (FIC) was interpreted by the following formula and subsequent isobologram was plotted.

The sum FIC value for each of the preparations determined by the following formula was used to classify the drug–drug interaction.

Σ FIC <0·5 represents substantial synergism, Σ FIC <1 represents synergism, Σ FIC 1 and <2 represents additive interaction, Σ FIC ⩾ 2 and <4 represents slight antagonism whereas Σ FIC ⩾ 4 represents marked antagonism.
Evaluation of in vivo antimalarial interaction of QN/QND with CLTR
Different groups of 5–10 mice were inoculated with 5 × 105P. yoelii nigeriensis MDR-infected erythrocytes. At 2–4 h after infection, these groups were treated with daily doses of QN and QND alone (given orally once a day), CLTR alone (given orally twice a day) and with various combination groups of QN and QND with CLTR. Mice in the control group were injected with parasites and received vehicle only. Parasitaemia was monitored by Giemsa-stained thin blood smears on pre-determined days and survival of the mice was duly recorded. To obtain numeric values for the interactions, results were expressed as the sum of the fractional inhibitory concentrations (sum FIC) at the given effective concentrations by the formula-
[ECx (effective concentration) of agent A in the mixture/ECx of agent A alone]+ [ECx of agent B in the mixture/ECx of agent B alone]. ‘Synergistic’ if sum FIC < 1; ‘fully additive’ if sum FIC = 1; ‘partially additive’ if sum FIC < 2 provided that both contributory FICs < 1; ‘antagonistic’ if any FIC > 1 (Tripathi et al. Reference Tripathi, Pandey and Rizvi2011).
Assessment of liver and kidney toxicity
Blood was withdrawn from mice of different experimental groups by cardiac puncture and allowed to stand undisturbed for 30 min. Serum was separated and levels of urea, alanine transaminase (ALT), aspartate aminotransferase (AST), blood urea nitrogen (BUN), total bilirubin (T BIL) and creatinine (CRT) were estimated using a fully automated biochemical analyser (Merck-selectra junior).
Statistical analysis
The 50% inhibitory concentration (IC50) of tested drugs was obtained by transferring the data into a graphic program (e.g. Excel) and expressed as the percentage of the untreated controls and then evaluated by Logit regression analysis using pre-programmed Excel spreadsheet obtained from the MMV group at Swiss Tropical Institute, Basel, Switzerland (Singh et al. Reference Singh, Srivastava, Srivastava, Puri and Srivastava2011).
The mean or mean ± S.D. were used for statistical calculations, FICs were calculated as per the formula given in the Materials and Methods section. Results were confirmed by repeated experiments (Each in vivo experiment was repeated twice, while in vitro it was carried out 4 times).
RESULTS
In vitro antimalarial activity of QN, QND and CLTR
QN, QND, CLTR and CQ were assayed for their in vitro antimalarial activity against P. falciparum 3D7 strain by SYBR green-I based fluorescence assay. The IC50 values of QN, QND, and CQ were found to be 49·2 ± 7·24 nM, 26·8 ± 3·87 nM and 14·17 ± 2·42 nM respectively, while for CLTR, the observed value was 19·05 ± 4·26μ m.
In vitro interaction between QN-CLTR and QND-CLTR
Antimalarial activity of QN and QND was investigated in combination with CLTR against P. falciparum 3D7. Results indicate that both QN-CLTR and QND-CLTR interact synergistically against the malaria parasite having sum FIC values ⩽1 in all tested ratios (Fig. 1A, B). The median sum of the FICs for the antimalarial interaction between QN and CLTR was 0·85 (0·69–1·0) while it was 0·64 (0·52–0·84) for the QND-CLTR combination. Results suggest that both the combinations have synergistic antimalarial potential; however, the QND-CLTR combination produced stronger antimalarial potential than QN-CLTR (Table 1).
Table 1. Sum of FICs for the interactions of QN-CLTR and QND-CLTR against 3D7 clones of Plasmodium falciparum (Mean±s.d.)

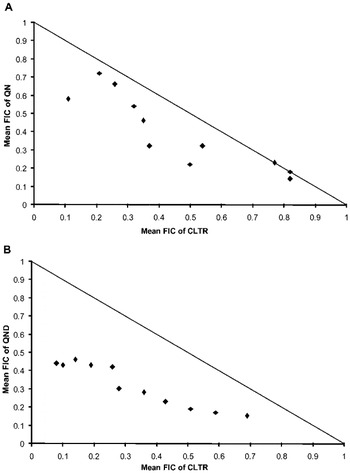
Fig. 1. The isobolograms representing in vitro antimalarial interactions. (A) The interaction between QN and CLTR. (B) The interaction between QND and CLTR.
In vivo interaction of QN and QND with CLTR
In the present study the antimalarial potential of oral QN and QND with CLTR has been evaluated against P. yoelii nigeriensis MDR–a multidrug-resistant rodent infection in random-bred Swiss mice following administration of individual drugs and their combinations.
QN at 200 mg/kg/day for 4 days and QND at 200 mg/kg/day for 4 days and 150 mg/kg/day for 4 days could produced only 0, 20, and 0% cure respectively, while CLTR at doses of 150 mg/kg/day for 7 days and 200 mg/kg/day for 7 days has shown a maximum 0% cure. In the combination (QN 200 + CLTR 200) MST was enhanced up to >28days while in their corresponding single-drug-treated groups, it was 8·6 and 13·2 days. Likewise in another combination (QND 200 + CLTR 150) MST was >28 days, while in their corresponding single-drug-treated groups, it was 15·4 and 12·8 days. Other combinations with lower doses showed MST enhancement (Table 2).
Table 2. Antimalarial response of QN, QND and CLTR against Plasmodium yoelii nigeriensis MDR in Swiss mice

The combination of QN or QND with CLTR enhances the antimalarial potential of these drugs. When QN and CLTR were administered at 200 mg/kg dose of each, 100% cure was observed with no recrudescence until day 28 and all the cured mice survived beyond the observation period. While no individual drug could produce >20% cure with <13·2 days of MST. However, lower doses of CLTR (150 mg/kg/day for 7 days) and the same dose of QN i.e. 200 mg/kg/day for 4 days produced only 60% cure with a mean survival time of 21·2 days. Further lower doses of both drugs in combination (150 mg/kg/day for 4 days QN + 150 mg/kg/day for 7 days CLTR and 150 mg/kg/day for 4 days QN + 75 mg/kg/day for 7 days CLTR) also showed better activity than their single-drug-treated groups. The combination of QND and CLTR at a dose of 200 mg/kg/day for 4 days QND and 150 mg/kg/day for 7 days CLTR showed a robust increase in MST (>28 days) with 100% survival and cure compared to the group which received individual drugs.
Furthermore, reducing the dose of both QND and CLTR (150 mg/kg/day for 4 days + 150 mg/kg/day for 7 days) resulted in slightly reduced antimalarial potential (80% cure). One mouse of this group showed parasitaemia on day 18; however, on day 28 it was cleared and all the mice of the group survived beyond day 28. Further lower doses i.e. 150 mg/kg/day for 4 days QND + 75 mg/kg/day for 7 days CLTR also showed a better response (40% cure) than either partner. Data suggest that QND+ CLTR combinations have better synergistic potential than QN + CLTR (Table 2).
The median (range) sums of the FICs for the antimalarial interaction between QN and QND with CLTR against MDR strain in Swiss mice were <1 and <0·88 respectively. The sums of the FICs of <1·0 in both experiments indicated synergistic interaction between the used drug combinations (Table 3).
Table 3. Sum of FICs for the interaction of QN/QND with CLTR against Plasmodium yoelii nigeriensis MDR

FICs-Synergy < 1; addtivity 1; antagonism > 1.
Assessment of liver and kidney toxicity
A slight increase in liver enzymes ALT and AST was observed in both the combinations (QN + CLTR and QND + CLTR). In QN + CLTR treated mice serum urea, total bilirubin, and blood urea nitrogen were found to be normal, only a minor increase in creatinine, ALT and AST levels was observed. While in the QND + CLTR combination urea and BUN levels were found to be normal with slightly elevated other parameters (Table 4).
Table 4. Biochemical parameters of mice liver and kidney from different groups on day 4 post-infection (P. yoelii nigeriensis)

DISCUSSION AND CONCLUSIONS
This is the first report indicating the antimalarial potentiation of QN and QND with CLTR against multidrug-resistant (MDR) malaria parasites.
CLTR is widely used for the treatment of respiratory tract infections, sexually transmitted diseases, Helicobacter pylori-associated peptic ulcers (King, Reference King1995; Zuckerman et al. Reference Zuckerman, Qamar and Bono2009; Calza et al. Reference Calza, Medana, Padovano, Giancotti and Baiocchi2012) and is a known inhibitor of bacterial 50S as well as 30S ribosomes (Champney et al. Reference Champney, Tober and Burdine1998). Furthermore, CLTR is also effective against other parasites like amoebae and helminths (Mathis et al. Reference Mathis, Wild, Deplazes and Boettger2004, Reference Mathis, Wild, Boettger, Kapel and Deplazes2005).
The in vitro antimalarial property of CLTR was first reported by Wisedpanichkij et al. (Reference Wisedpanichkij, Chaijaroenkula, Sangsuwanb, Tantisawat, Boonpraserta and Na-Bangchanga2009). These authors found that it has relatively low antimalarial potential against P. falciparum (IC50 >10μ m). Subsequently, in our previous publication (Tripathi et al. Reference Tripathi, Pandey and Rizvi2011), we reported the antimalarial effect of CLTR against MDR strain of P. yoelii nigeriensis in Swiss mice. In the present communication, our results are in agreement with Wisedpanichkij et al. (Reference Wisedpanichkij, Chaijaroenkula, Sangsuwanb, Tantisawat, Boonpraserta and Na-Bangchanga2009) and an IC50 of CLTR against P. falciparum 3D7 was found to be 19·05 ± 4·26 μ m.
QN and QND are well known traditional and affordable antimalarials. However, drug resistance against QN has confined its use against resistant malaria parasites (Wongsrichanalai et al. Reference Wongsrichanalai, Pickard, Wernsdorfer and Meshnick2002). Although the first observation of QN resistance was reported 100 years ago in Brazil, the clinically significant resistance to quinine was observed in 1960s from the Thai-Cambodian border. Currently it is sporadic in Southeast Asia and Western Oceania and is associated with mutation in the PfMDR gene of the malaria parasite (Wongsrichanalai et al. Reference Wongsrichanalai, Pickard, Wernsdorfer and Meshnick2002; Sidhu et al. Reference Sidhu, Valderramos and David2005).
The literature supports our finding that QND has better antimalarial effect than QN (White et al.Reference White, Looareesuwan, Warrell, Chongsuphajaisiddhi, Bunnag and Harinasuta1981; Kazim et al. Reference Kazim, Puri and Dutta1991) and it is evident in our in vitro as well as in vivo results. QND and QN have an IC50 26·8 ± 3·87 nM and 49·2 ± 7·24 nM respectively against P. falciparum. When QND was given at a dose of 200 mg/kg/day for 4 days in P. yoelii nigeriensis MDR-infected Swiss mice, it produced a 20% curative effect with a mean survival time of 15·4 days while at the same dose QN could not cure any treated animal and mice survived only for 13·2 days.
In uncomplicated malaria patients, following disease recovery with QN treatment, clearance of the drug increases and the volume of distribution expands and, as a result, the plasma concentration of the QN goes down, thereby affecting its antimalarial property. This may cause the problem of drug failure. Therefore, it is recommended to give QN in combination with a suitable partner drug in resistant areas (Na-Bangchang and Karbwang, Reference Na-Bangchang and Karbwang2009). Here, we have tried to explore CLTR as a partner for QN and QND, based on its PK-PD properties.
Hepatic biotransformation of drugs by the CYP enzymes is mainly responsible for drug elimination and, among these CYPs, CYP3A4 accounts for 30–40% of the total amount of identified drug-metabolizing cytochromes (Dorne et al. Reference Dorne, Walton and Renwick2003). CLTR is metabolized to 14-hydroxy CLTR in the liver by CYP3A4 (Rodrigues et al. Reference Rodrigues, Roberts, Mulford, Yao and Ouellet1997; Suzuki et al. Reference Suzuki, Iida, Hirota, Akimoto, Huguchi, Suwa, Tani, Ishizaki and Chiba2003). Interestingly, it is also a potent inhibitor of its metabolizing enzyme CYP3A4 (Pinto et al. Reference Pinto, Wanq, Chalasani, Skaar, Kolwankar, Gorski, Lianqpunsaqul, Hamman, Arefayene and Hall2005). Favourable bioavailability, stability in the stomach and ability to achieve a high therapeutic level of oral CLTR makes it the preferred antibacterial treatment for children (Pai et al. Reference Pai, Graci and Amesden2000).
Moreover, QN and QND are metabolized to their 3-hydroxy metabolites through CYP3A4 (Nielsen et al. Reference Nielsen, Rasmussen, Flinois, Beaune and Brosen1999; Damkier and Brosen, Reference Damkier and Brosen2000; Mirghani et al. Reference Mirghani, Yasar, Zheng, Cook, Gustafsson, Tybring and Ericsson2002). It was reported that the antimalarial potential of some drugs can be enhanced when used in combination with selective CYP inhibitors (Awasthi et al. Reference Awasthi, Dutta, Bhakuni and Tripathi2004; Tripathi et al. Reference Tripathi, Awasthi and Dutta2005; Soyinka et al. Reference Soyinka, Onyeji, Omoruyi, Owolabi, Sarma and Cook2009; Ward et al. Reference Ward, Sorich, Evans and McKinnon2009; Wisedpanichkij et al. Reference Wisedpanichkij, Chaijaroenkula, Sangsuwanb, Tantisawat, Boonpraserta and Na-Bangchanga2009; Tripathi et al. Reference Tripathi, Pandey and Rizvi2011).
In P. yoelii nigeriensis MDR-infected mice, CLTR could enhance the antimalarial activity of QN/QND significantly. With a dose of 200 mg/kg/day for 4 days QN or QND in combination with 200 mg/kg and 150 mg/kg/day for 7 days CLTR respectively, 100% cure was produced, while single-drug groups showed a maximum 20% cure of treated animals. At lower doses both the combinations have also produced higher cure rates with prolonged survival time as compared to either drug.
Moreover, sums of the FICs for the antimalarial interaction between QN + CLTR and QND + CLTR was found to be <1 and <0·88 respectively. These data clearly indicate that both combinations have synergistic antimalarial potential against multidrug-resistant malaria parasites.
Thus, authors speculate that the synergistic antimalarial effect of CLTR with QN/QND in mice may be due to inhibition of the drug metabolizing enzyme CYP 3A4 which in turn increases the QN/QND producing enhanced antimalarial activity. Furthermore, CLTR is known to have a disrupting effect on the plasmodium apicoplast which is a vital organ of the malaria parasite (Kalanon and McFadden, Reference Kalanon and McFadden2010; Ekland et al. Reference Ekland, Schneider and Fidock2011). In in vitro studies we have seen clear synergism between CLTR and QN/QND that may be due to the same property of CLTR, as well as inhibition of heme polymerization of the malaria parasite through QN/QND (Sullivan et al. Reference Sullivan, Gluzman, Russell and Goldberg1996). A different site of action i.e. heme polymerization inhibition for QN, QND and apicoplast disruption as well as CYP inhibiting properties of CLTR might enable the combination of QN/QND and CLTR to synergize their antimalarial potential. In vitro combination results clearly show that both QN and QND have a synergistic interaction with CLTR, the mean ΣFIC values being 0·85 and 0·64 for QN + CLTR and QND + CLTR respectively. Additionally, isobolograms show that there is a marked synergism between QN/QND and CLTR.
During early malaria infection the liver and kidney are the most affected organs, being prone to biochemical changes (Nand et al. Reference Nand, Aggarwal, Sharma and Singh2001; Uzuegbu and Emeka, Reference Uzuegbu and Emeka2011). However, the present study reveals that combinations of QN/QND and CLTR administered orally were safe as evidenced by toxicity biomarkers, namely urea, alanine transaminase (ALT), aspartate aminotransferase (AST), bilirubin, creatinine and blood urea nitrogen (BUN). Serum levels of these biomarkers were comparable to the healthy control in the combination groups.
The World Health Organization recommends the use of artemisinin-based combination therapies (ACTs) to produce high cure rates of falciparum malaria and also to overcome the problem of drug resistance. However, the implementation of the ACT policy in the African public health sector is limited by unavailability, inaccessibility and the high cost of these drugs. As alternative combinations to ACT with high efficacy and good margins of safety, the World Health Organization (2006) had recommended QN in combination with either tetracycline or doxycycline or clindamycin (Whitty et al. Reference Whitty, Ansah, Leslie and Staedke2008). The tetracycline or doxycycline combination particularly with QN is reported to be a failure (World Malaria Report, 2011). In addition, these combinations were contra-indicated in children and pregnant women (Lell and Kremsner, Reference Lell and Kremsner2002), while CLTR was found to be quite safe in pregnancy. Einarson et al. (Reference Einarson, Phillips, Mawji, D'Alimonte, Schick, Addis, Mastroiacova, Mazzone, Matsui and Koren1998) had reported that this agent (CLTR) does not increase the rate of major malformations above the baseline risk of 1–3%. QN in combination with clindamycin has a faster parasite clearance rate than when used alone; however, compared to monotherapy, it is not necessarily related with reduced mortality (Lell and Kremsner, Reference Lell and Kremsner2002). Our experimental data clearly show that all the combination groups (QN/QND with CLTR) can significantly reduce the mortality rate in treated animals. Moreover, the major metabolite of QN i.e. 3-hydroxyquinine is proved to cause renal failure in patients (Newton et al. Reference Newton, Keeratithakul, Teja-Isavadharm, Pukrittayakamee, Kyle and White1999). In the present communication, the authors suggests that inhibition of CYP3A4 by CLTR may reduce the formation of 3-hydroxyquinine, thereby reducing the risk. Therefore, introduction of CLTR as a partner drug with quinine may be beneficial over other partners already in use.
In conclusion, we suggest that the antimalarial potential of QN/QND, the two most affordable antimalarials, may be enhanced significantly when used in combination with CLTR. The different site of action i.e. apicoplast for CLTR and heme polymerization inhibition by QN/QND might be helpful to prolong the antimalarial life as well as to overcome the problem of QN/QND resistance. However, further studies are needed to explain the role of CLTR to enhance the antimalarial activity of QN and QND. Since the parasite used in the present study is QN/QND resistant, enhanced antimalarial activity of combinations encourages the administration of these combinations in QN/QND resistant areas. Although the current study holds good for the murine model the clinical implication of the study needs to be evaluated further for human application.
ACKNOWLEDGEMENTS
The authors are grateful to the Director, C.D.R.I. and Head, Division of Parasitology, C.D.R.I. for their continuous support throughout the study. S.K.P. is thankful to Professor Shakir Ali, Department of Biochemistry, Jamia Hamdard for his valuable suggestions. CSIR-CDRI communication number 8337.