Introduction
Dicrocoeliosis caused by Dicrocoelium spp. (small liver fluke) is a global parasitic disease of wide economic implications and public health concern (Gonzalez-Lanza et al., Reference Gonzalez-Lanza, Manga-Gonzalez and Del-PozoCarnero1993; Campo et al., Reference Campo, Manga-Gonzalez and Gonzalez–Lanza2000). In India, limited information is available on the economic impact of this disease. The estimation becomes more complicated because dicrocoeliosis is often either not detected clinically or not diagnosed due to its subclinical nature (Ducommun & Pfister, Reference Ducommun and Pfister1991). The impact of parasitism on the livestock production also depends on the severity of infection, extent of the exposure, immunity of the host and the metabolic cost of a competent immune system. Dicrocoelium dendriticum shows cosmopolitan distribution throughout Asia, Europe, North Africa and North America (Soulsby, Reference Soulsby1968). Dicrocoelium dendriticum parasitizes a number of hosts, particularly domestic and wild ruminants, but rabbits, pigs, dogs, horses and humans can also be infected occasionally (Otranto & Traversa, Reference Otranto and Traversa2003). In addition to primary hosts, their distribution is contingent upon availability of intermediate hosts (snails and ants) and other environmental factors (temperature and humidity). The hepatic lesions caused by D. dendriticum often remain undetected as compared to lesions caused by other liver flukes. This is due to masking of its clinical signs by mixed infections of other more pathogenic helminths (Theodoridis et al., Reference Theodoridis, Duncan, Maclean and Himonas1991). In fact, it is difficult to investigate the pathogenesis of D. dendriticum under both natural and experimental conditions. The lack of evident lesions and clinical signs in the case of dicrocoeliosis may also be due to the behaviour of young flukes, which migrate directly up the biliary duct system of the liver without penetrating the gut wall or liver parenchyma (Theodoridis et al., Reference Theodoridis, Duncan, Maclean and Himonas1991). Studies related to pathogenesis under experimental conditions have shown a slight increase in bilirubin (7%) and albumin (3%), which is not, however, related to the worm burden (Otranto & Traversa, Reference Otranto and Traversa2002; Manga-Gonzalez et al., Reference Manga-Gonzalez, Ferreras, Campo, Gonzalez-Lanza, Perez and Garcia-Marin2004). Under natural infections, the burden of up to 4000 adult flukes does not cause any significant loss in the blood and plasma proteins (Theodoridis et al., Reference Theodoridis, Duncan, Maclean and Himonas1991). However, cholangitis and cholangiectasia have been described in both natural and experimental infections (Ranucci et al., Reference Ranucci, Mughetti, Ambrosi and Grelloni1981; Wolff et al., Reference Wolff, Hauser and Wild1984; Manga-Gonzalez et al., Reference Manga-Gonzalez, Ferreras, Campo, Gonzalez-Lanza, Perez and Garcia-Marin2004). In the case of severe infections, necrotic and haemorrhagic tracts have been reported. Moreover, portal hepatitis and portal, septal and, on some occasions, perisinusoidal fibrosis have also been observed (Manga-Gonzalez et al., Reference Manga-Gonzalez, Ferreras, Campo, Gonzalez-Lanza, Perez and Garcia-Marin2004). From the clinical aspect, the most significant sign of dicrocoeliosis is the reduced weight gain at the early stages of infection (Salimova, Reference Salimova1972; Manga-Gonzalez et al., Reference Manga-Gonzalez, Ferreras, Campo, Gonzalez-Lanza, Perez and Garcia-Marin2004). However, the clinical signs are not pathognomonic, even in severe infections. The animals under severe conditions can develop anaemia, icterus, oedema and a slight reduction in production.
Because of its subclinical nature, dicrocoeliosis often remains clinically undiagnosed (Ducommun & Pfister, Reference Ducommun and Pfister1991). The most commonly used diagnostic technique includes the coprological examination for the detection of fluke eggs or recovering adults from the liver at necropsy (Otranto & Traversa, Reference Otranto and Traversa2002). However, absence of eggs in the pre-patent phase (Nour Eldin et al., Reference Nour Eldin, EL- Ganaini, Abou EL-Enin, Hussein and Sultan2004) and intermittent release of eggs in the mature phase makes the results unreliable. The immunological methods for diagnosing dicrocoeliosis are based on the detection of antibodies in the serum against D. dendriticum. Various immunodiagnostic techniques like immunofluorescence (Calamel, Reference Calamel1977), passive haemagglutination test, complement fixation, enzyme-linked immunosorbent assay (ELISA) and Western blotting (Wedrychowicz et al., Reference Wedrychowicz, Ducommun, Gorski and Pfister1995, Reference Wedrychowicz, Ducommun, Bambera and Pfister1997; Gonzalez-Lanza et al., Reference Gonzalez-Lanza, Manga-Gonzalez, Compo, Del-Pozo, Sandoval, Oleaga and Ramajo2000) have been employed to detect anti-Dicrocoelium antibodies in naturally and/or experimentally infected animals.
Control of dicrocoeliosis is difficult and unsatisfactory because of the epidemiology and complex life cycle of its etiological agent. For the effective control of dicrocoeliosis, an accurate characterization of D. dendriticum at different taxonomic levels is essential. Although the morphological characteristics are used as the basis to identify and differentiate the species of Dicrocoelium, their accurate identification is difficult because of their close resemblance with closely related species. Thus, it becomes necessary to use alternative techniques, like the modern molecular tools and markers for their identification and differentiation (Gasser et al., Reference Gasser, Rossi and Zhu1999, Reference Gasser, Bott, Chilton, Hunt and Beveridge2008; Martinez-Ibeas et al., Reference Martinez-Ibeas, Martınez-Valladares, Gonzalez-Lanza, Minambres and Manga- Gonzalez2011; Huang et al., Reference Huang, Zhao, Fu, Xu, Wang, Wu, Zou and Zhu2012; Wang et al., Reference Wang, Gao, Zhu and Zhao2012). For this purpose, a number of genes have been used that include the sequences of the first internal transcribed spacer (ITS-1), the 5.8S and the second internal transcribed spacer (ITS-2) of the nuclear ribosomal DNA (rDNA), 28S ribosomal ribonucleic acid (rRNA) (Adlard et al., Reference Adlard, Barker, Blair and Cribb1993; Itagaki & Tsutsumi, Reference Itagaki and Tsutsumi1998; Marcilla et al., Reference Marcilla, Bargues and Mas-Coma2002; Itagaki et al., Reference Itagaki, Kikawa, Sakaguchi, Shimo, Terasaki and Shibahara2005a; Le et al., Reference Le, De, Agatsuma, Nguyen, Nguyen and McManus2008; Ichikawa & Itagaki, Reference Ichikawa and Itagaki2010), 18S rRNA (Karimi, Reference Karimi2008), mitochondrial nicotinamide adenine dinucleotide (NAD) + hydrogen (H) dehydrogenase I (NDI) and cytochrome c oxidase I (COI) genes (Hashimoto et al., Reference Hashimoto, Watanabe, Liu, Init, Blair and Ohnishi1997; Itagaki et al., Reference Itagaki, Kikawa, Terasaki, Shibahara and Fukuda2005b). The 18S and ITS-2 of rDNA have been employed for clear differentiation of D. dendriticum and Dicrocoelium chinensis (Otranto et al., Reference Otranto, Rebien, Weigl, Cantacessi, Parisi, Paolo Lia, Meter and Olson2007). Moreover, the 28S and ITS-2 rDNA have also been utilized to differentiate D. dendriticum and Dicrocoelium hospes (Maurelli et al., Reference Maurelli, Rinaldi, Capuano, Perugini, Veneziano and Cringoli2007). The larval stages of D. dendriticum in their intermediate hosts (snail and ant) were also detected based on their mitochondrial cox 1 and ITS-2 rDNA sequences (Martınez-Ibeas et al., Reference Martinez-Ibeas, Martınez-Valladares, Gonzalez-Lanza, Minambres and Manga- Gonzalez2011).
Keeping all this in view, the current study was designed to properly identify the species D. dendriticum and differentiate it from other species of Dicrocoelium using the morphological and molecular data. The study was also carried out to recognize the somatic and excretory/secretory (E/S) antigenic profile of D. dendriticum by immunoblotting technique using sera from sheep naturally infected with D. dendriticum.
Materials and methods
Collection and identification of parasites
Adult flukes of D. dendriticum were collected from the bile ducts of naturally infected Sheep (Ovis aries) slaughtered for consumption at the local slaughterhouses. Parasites were identified to species according to morphological features (Taira et al., Reference Taira, Shirasaka, Taira, Ando and Adachi2006; Otranto et al., Reference Otranto, Rebien, Weigl, Cantacessi, Parisi, Paolo Lia, Meter and Olson2007).
Genomic DNA isolation, quality check and amplification
Genomic DNA was extracted using a NucleoSpin® Tissue Kit (Macherey-Nagel, Duren, Germany) following the manufacturer's instructions. The extracted DNA was treated with RNAase (100 mg/ml), split into aliquots and stored at −20°C until used. The quality of the isolated DNA was checked using agarose gel electrophoresis by using 0.8% of agarose gel in 0.5X Tris/Borate/EDTA (TBE) containing 0.5 μg/ml ethidium bromide.
Polymerase chain reaction (PCR) was used to amplify the nuclear ITS-2 of rDNA by using the primers CAS5p8sFt (forward: 5′-TGAACATCGACATTTYGAACGCATAT-3′) and CAS28sB1d (reverse: 5′-TTCTTTTCCTCCSCTTAYTTGATATGCTTAA-3′) (Ji et al., Reference Ji, Zhang and He2003). PCR amplification was carried out in a 20 μl reaction volume, which contained 1X Phire PCR buffer (contains 1.5 mm magnesium chloride), 0.2 mm each deoxyribonucleotide triphosphate (dNTP) {(deoxyadenosine triphosphate (dATP), deoxythymidine triphosphate (dTTP), deoxycytidine triphosphate (dCTP), deoxyguanosine triphosphate (dGTP)}, 1 μl DNA, 0.2 μl PhireHotstart II DNA polymerase enzyme, 0.1 mg/ml Bovine Serum Albumin (BSA) and 3% Dimethyl sulfoxide (DMSO), 0.5 M betaine and 5 pM of forward and reverse primers. The PCR amplification was carried out in a PCR thermal cycler (GeneAmp PCR System 9700, Applied Biosystems, California, U.S.A) with initiation at 95°C for 5 min, followed by 35 cycles including denaturation at 95°C for 1 min, annealing at 50°C for 1 min and extension at 72°C for 50 s; a final extension step consisting of incubation at 72°C for 10 min was included. The PCR products were checked in 1.2% agarose gels prepared in 0.5X TBE buffer containing 0.5 μg/ml ethidium bromide. The gels were visualized in an ultraviolet (UV) transilluminator (Genei, Banglore, India) and the image was captured under UV light using a gel documentation system (Bio-Rad, California, U.S.A).
Sequencing of the ITS-2 amplicons and phylogenetic analysis
After complete amplification of the DNA samples, the amplified products were sent to Rajiv Gandhi Centre for Biotechnology, Thiruvananthapuram, Kerala, for sequencing. BioEdit Sequence Alignment Editor (Hall, Reference Hall1999) was used to trim and annotate the raw sequences. All the edited sequences were aligned in MEGA 7.0 (Kumar et al., Reference Kumar, Stecher and Tamura2016) using the clustalW algorithm (Thompson et al., Reference Thompson, Higgins and Gibson1994) in order to trace individual mutations. The sequences were identified using the BLAST function from the National Center for Biotechnology Information (NCBI), applying the default parameter. Additional sequences of D. dendriticum along with other species of Dicrocoelium from different geographical regions were retrieved from GenBank for sequence alignment and phylogenetic tree construction. Phylogenetic trees were constructed using the minimum-evolution method (Rzhetsky & Nei, Reference Rzhetsky and Nei1992) in MEGA 7.0 (Kumar et al., Reference Kumar, Stecher and Tamura2016) based on the Tamura and Nei model (Reference Tamura and Nei1993).
Preparation of crude somatic and E/S fraction of proteins
Freshly collected samples were washed with ice-cold 0.01% phosphate-buffered saline PBS (pH 7.4) three times so as to reduce muscle constriction of the acetabulum and oral sucker, to avoid release of E/S products and enzymes of the parasites, as described by Anuracpreeda et al. (Reference Anuracpreeda, Wanichanon, Chawengkirtikul, Chaithirayanon and Sobhon2009), with slight modifications. Protein extracts were obtained by homogenizing the flukes in ice-cold lysis buffer (0.01 M PBS, pH 7.2, containing 10 mm Tris hydrochloride, 150 mm sodium chloride, 0.5% Triton X-100, 1 mm ethylenediaminetetraacetic acid (EDTA), 1 mm phenylmethanesulfonyl fluoride (PMSF) and a 100 μl cocktail of protease inhibitors (Roche, Basel, Switzerland) using tissue homogenizer. Homogenization was carried out at 4°C for 10 min at 1300 rpm, taking pause of 2 min after every one minute of homogenization. After homogenization, the mixture was allowed to stand for half an hour on ice until the foam settled down. Thereafter, the suspension was sonicated for 10 min (pulse 10, lapse 30 and amplitude 70%) in an ice bath and centrifuged at 13,000 rpm for 20 min at 4°C. The supernatant protein fraction was concentrated using chilled acetone and aliquoted and stored at −20°C.
E/S proteins were prepared from living flukes according to Phiri et al. (Reference Phiri, Phiri and Harrison2006), with slight modifications. The adult worms of D. dendriticum were collected from the bile ducts and washed three times in 0.01 M PBS, pH 7.4, at room temperature. The cleaned worms were then incubated (40 worms per 100 ml) in RPMI-1640 medium containing 2 mm PMSF, 100 IU of penicillin and 100 μg of streptomycin per millilitre of medium for about 3 h at 37°C. After incubation, the worms were removed from the medium and the suspension containing the E/S proteins of the worms was centrifuged at 13,000 rpm for 30 min at 4°C. The supernatant was collected, aliquoted and stored at −20°C. The protein concentrations of both fractions were measured according to Bradford (Reference Bradford1976).
Collection of serum samples
Blood samples were collected from local abattoirs at the time of slaughtering of sheep with the monospecific infections of D. dendriticum. Blood samples were also obtained from uninfected sheep serving as the negative control. The infections were ascertained by post-mortem examinations. Sera were obtained from each clotted blood samples by centrifugation at 4000 rpm for 10 min and stored at −20°C.
Polypeptide analysis by sodium dodecyl sulphate–polyacrylamide gel electrophoresis (SDS–PAGE)
Somatic and E/S protein fractions of these liver flukes were separated by SDS–PAGE as described by Laemmli (Reference Laemmli1970). The protein samples were heated in a water bath at 100°C under denaturing and reducing conditions for 10 min. Samples were then loaded to each well of a 6% stacking gel and 12% separating gel and electrophoresis was carried out in Mini Protean II electrophoresis apparatus (Bio-Rad) at 90 V for about 4–5 h. Gels were stained with 0.05% Coomassie Brilliant Blue. The molecular weights of proteins were then determined by comparing their migration distance against that of a known molecular weight marker.
Antigenic analysis by Western blotting
Antigenically active components among the resolved bands in SDS–PAGE were detected by Western blotting. After SDS–PAGE, the unstained gels were transferred electrophoretically onto a nitrocellulose sheet using a transfer blot apparatus. Nitrocellulose sheets containing transferred sample strips were incubated in a blocking solution (1% skimmed milk and 0.1% Tween-20 in 100 mm PBS, pH 7.4) overnight at 4°C. The strips were then incubated with sera containing test antibodies. All sera were diluted 1:1000 in TBS and incubated at 4°C overnight, with gentle shaking. Following three PBS washes to remove unbound antibodies, the nitrocellulose sheets were then incubated for 1 h in horseradish peroxidase conjugate anti-Immunoglobulin-G antibodies. Unbound conjugate was removed by washing three times in PBS, before the addition of substrate solution containing 3,3′-diaminobenzidine. Gels were then visualized under the Bio-Rad gel documentation system.
Results
Molecular characterization
The amplified product of ITS-2 of D. dendriticum using the primers CAS5p8sFt (forward: 5′-TGAACATCGACATTTYGAACGCATAT-3′) and CAS28sB1d (reverse: 5′-TTCTTTTCCTCCSCTTAYTTGATATGCTTAA-3′) yielded the fragment of 500 bp (fig. 1). The sequence analysis showed that ITS-2 rDNA had a length of 238 base pairs, and, along with ITS-2, were partial sequences of 5.8S and 28SrDNA sequences. The annotated sequence is shown in fig. 2, and the same sequences were submitted to NCBI via Banklt with accession numbers MH048705, MH753596, MH753597, MH753598 and MH753599.

Fig. 1. Representative gel (1.2% agarose) image of amplified ITS-2 region of rDNA (L1–L5: Dicrocoelium dendriticum).

Fig. 2. Annotated sequences of Dicrocoelium dendriticum isolate (5.8S rRNA gene, partial sequence (yellow); ITS-2, complete sequence (blue); and 28S rRNA gene, partial sequence (purple)).
The BLAST analysis of rDNA ITS-2 sequences JD1–JD5 isolates showed 98–100% similarity with D. dendriticum.
The phylogeny of Dicrocoelium species based on ITS-2 sequences was analysed using the minimum-evolution method. The present isolates formed a monophyletic clade with the already described species of D. dendriticum identified from different parts of the world. The bootstrap values are shown next to clades, and D. hospes (EF102026) was used as the outgroup taxa (fig. 3). The sequence compositions of the ITS-2 regions of JD1–JD5 are 48.32% (GC content), 51.68% (AT content), A = 24.37% (58), C = 23.95% (57), G = 24.37% (58), T = 27.31% (65) (table 1). By comparing the ITS-2 sequences of our D. dendriticum isolates with the other species of Dicrocoelium, like Dicrocoelium orientalis and D. chinensis, the species were found to differentiate at 12 nucleotide sites, viz.: 1, 6, 57, 116, 131, 133, 135, 190, 201, 202, 209 and 232 (fig. 4).

Fig. 3. Minimum-evolution phylogenetic tree, the bootstrap test (10,000 replicates), based on ITS-2 gene of Dicrocoelium dendriticum isolates, with Dicrocoelium hospes (EF102026) designated as outgroup. The analysis involved 18 nucleotide sequences. The evolutionary distances were computed using the Tamura–Nei method. All ambiguous positions were removed from each sequence pair. There were a total of 242 positions in the final dataset. Evolutionary analyses were conducted in MEGA 7.0. Scale bar shows the number of nucleotide substitutions per site.
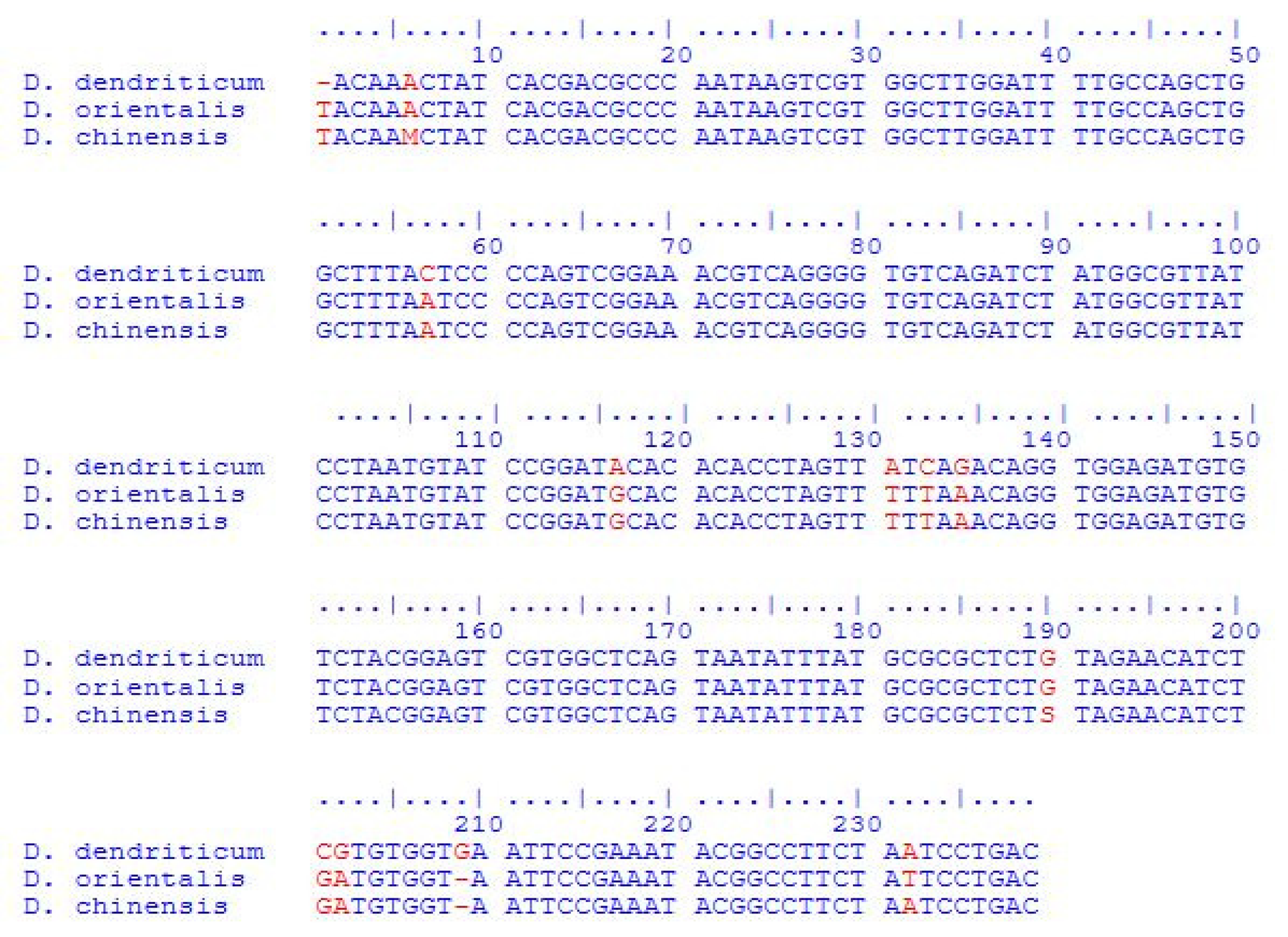
Fig. 4. Interspecies alignment of sequences of Dicrocoelium dendriticum, Dicrocoelium orientalis and Dicrocoelium chinensis, depicting variations at 12 positions in ITS-2 using the ClustalW alignment tool.
Table 1. Comparison of sequence lengths and composition of ITS-2 between Dicrocoelium species from different parts of the world and present isolate JD1.

The distance matrix analysis carried out by p-distance and the maximum composite likelihood method of MEGA 7.0 showed 100% similarity and zero total character difference with sequences of already reported D. dendriticum isolates from various parts of the world. However, with other closely related species of the same genus, 7–26 bp differences were observed (table 2).
Table 2. Distance matrix analysis of the ITS-2 region between Dicrocoelium species.

SDS–PAGE and immunoblotting
SDS–PAGE analysis of somatic protein extract of D. dendriticum revealed 16 protein bands between the molecular weights ranges of 15–175 kDa under reducing conditions (SDS–PAGE, 6% stacking and 12% resolving gel) (fig. 5). Western blotting analysis of somatic proteins with the sera of sheep naturally infected with D. dendriticum showed six bands with molecular weights of 27, 42, 66, 84, 97 and 130 kDa (fig. 6). Among these, the protein band of 130 kDa was most prevalent and was found to react with five out of six positive sera samples, while the 84 kDa band was found to react with four positive sera samples. No band was observed in the negative control sera.

Fig. 5. Coomassie Brilliant Blue-stained protein profile of somatic antigens of Dicrocoelium dendriticum from different sheep livers (lanes L1–L6). M, molecular marker.

Fig. 6. Western blot analysis of somatic antigens of Dicrocoelium dendriticum against serum samples. M, molecular weight marker. 1–6 = positive sera of D. dendriticum; 7–12 = negative sera of D. dendriticum.
The electrophoretic protein profile of E/S antigens of D. dendriticum under reducing conditions revealed nine protein bands with molecular weights ranging between 15 and 140 kDa (fig. 7). Western blotting analysis of E/S proteins with the sera of sheep naturally infected with D. dendriticum showed five bands with molecular weights of 27, 42, 50, 62 and 130 kDa (fig. 8). Among these, the protein band of 130 kDa was found to react with five out of six positive sera samples. No band was observed in the negative control sera.

Fig. 7. Coomassie Brilliant Blue-stained protein profile of E/S antigens of Dicrocoelium dendriticum from different sheep livers (lanes L1–L4). M, molecular marker.

Fig. 8. Western blot analysis of E/S antigens of Dicrocoelium dendriticum against serum samples. M, molecular weight marker. 1–6 = positive sera of D. dendriticum; 7–12 = negative sera of D. dendriticum.
Thus, protein bands with molecular weights of 130 and 84 kDa in somatic fraction and 130 kDa in E/S fraction of D. dendriticum were found to be highly specific.
Discussion
The accurate identification of Dicrocoelium species has an important implication for the epidemiology, control and management of dicrocoeliosis. The phylogenetic analysis of Dicrocoelium species based on their ITS-2 sequences using the minimum-evolution method showed that the isolates JD1–JD5 formed the clade with D. dendriticum isolates from different countries. ITS-2 sequences of D. dendriticum isolates in our study formed a single cluster with the D. dendriticum isolates from Slovakia, Italy, China and Iran, with significant bootstrap values. In the current study, ITS-2 was used as a genetic marker to identify the species of Dicrocoelium from the Kashmir Valley of India. ITS-2 sequences of Dicrocoelium isolates in our study were also compared with sequences of closely related species that exist in the GenBank database. According to the results obtained from the study, we concluded that D. dendriticum is the only species of Dicrocoelium that exists in the valley of Kashmir. No significant intraspecific variation was revealed among different isolates of D. dendriticum. However, considerable variation in the nucleotide sequence of ITS-2 was revealed when compared with the closely related species of Dicrocoelium from other parts of the world. For example, by comparing the ITS-2 sequences of our D. dendriticum isolates with the other species of Dicrocoelium, like D. orientalis and D. chinensis, the species were found to differ at 12 nucleotide sites, viz.: 1, 6, 57, 116, 131, 133, 135, 190, 201, 202, 209 and 232.
Consistent with our studies, Arbabi et al. (Reference Arbabi, Dalimi-Asl, Ghaffarifar and Foorozandeh-Moghadam2012) failed to demonstrate any significant intraspecific variation in the rDNA among different isolates of D. dendriticum. Similarly, Maurelli et al. (Reference Maurelli, Rinaldi, Capuano, Perugini, Veneziano and Cringoli2007) reported the ITS-2 intraspecific variation of less than 1% in different isolates of D. dendriticum from different hosts, while the authors reported a higher interspecific variation of 8.2–8.5% between D. dendriticum and D. hospes. Along the similar lines, in the case of different isolates of D. dendriticum, Otranto et al. (Reference Otranto, Rebien, Weigl, Cantacessi, Parisi, Paolo Lia, Meter and Olson2007) reported a low intraspecific variation of about 1.3% in the ITS-2 region of rDNA, irrespective of host species (sheep and cattle) or localities (Italy and Germany). Our results were also in accordance with the studies of Bian et al. (Reference Bian, Zhao, Jia, Fang, Cheng, Du, Ma and Lin2015), who also used ITS-2 along with ITS-1 and 5.8S for the accurate identification and estimation of genetic variation in Dicrocoelium isolates. The authors reported the lengths of ITS-1, 5.8S and ITS-2 as 749, 161 and 234 bp, respectively, and the species was identified as D. dendriticum from NCBI-BLAST search, as well as from phylogenetic analysis. Whilst the intraspecific sequence variation among the D. dendriticum species was found to be 0–0.5% for ITS-1 and 0–1.3% for ITS-2 rDNA, the interspecific variations between different species in genus Dicrocoelium in ITS-2 rDNA were found to be 3.4–12.3%.
In addition to nuclear rDNA, mitochondrial genes like COI and NDI have also been used for the proper identification and characterization of Dicrocoelium species. For example, Liu et al. (Reference Liu, Yan, Otranto, Wang, Zhao, Jia and Zhu2014) differentiated the two species of lancet liver flukes as D. dendriticum and D. chinensis based on mitochondrial and nuclear rDNA sequences. Results from the sequence comparison of a conserved mitochondrial gene and nuclear rDNA sequences among the number of lancet flukes revealed considerable nucleotide variation between the species but limited nucleotide variation within each of them.
The lower level of intraspecific nucleotide differences among the isolates of D. dendriticum, irrespective of host and locality, suggested a limited genetic affinity among the isolates of D. dendriticum and their host species. These findings accounted for a possible genetic stability within D. dendriticum population, as revealed for other generalist parasites, which infect multiple species of hosts all around the world (Humbert & Cabaret, Reference Humbert and Cabaret1995; Nolan & Cribb, Reference Nolan and Cribb2005). However, it cannot be ruled out that a larger number of isolates sampled from different localities and host species may show a pattern of population subdivision not found in a more limited sample.
In our studies with D. dendriticum, SDS–PAGE analysis of somatic protein extract revealed 16 protein bands between the molecular weight range of 15–175 kDa under reducing conditions (SDS–PAGE, 10% stacking and 12% resolving gel), while the electrophoretic protein profile of E/S antigens of D. dendriticum under similar conditions revealed nine protein bands with molecular weights ranging between 15 and 140 kDa. Western blotting analysis of somatic proteins with the sera of sheep naturally infected with D. dendriticum showed six bands with molecular weights of 27, 42, 66, 84, 97 and 130 kDa. Among these, the protein bands of 84 and 130 kDa were found to react with four and five sera, respectively, out of six positive sera tested. No band was observed in the negative control sera. Western blotting analysis of E/S proteins with the sera of sheep naturally infected with D. dendriticum showed five bands with molecular weights of 27, 42, 50, 62 and 130 kDa. Among these, the protein bands of 130 kDa were found to react with five out of six positive sera samples tested.
Since there is limited information about the proteomic study of D. dendriticum, we have been able to make a lesser number of comparisons of our results with those of other researchers. By SDS–PAGE analysis, Revilla-Nuin et al. (Reference Revilla-Nuin, Manga-Gonzalez, Minanbres and Gonzalez-Lanza2005) showed a greater number of polypeptides in both somatic (36) and E/S (18) antigenic fractions than our study in the case of D. dendriticum; however, most of their bands were similar to our study. Moreover, the authors also revealed eight immunoreactive antigenic polypeptides with molecular weights ranging from 24 to 205 kDa for somatic antigen and seven immunoreactive polypeptides between the molecular weight ranges of 26 and 205 kDa for E/S product of D. dendriticum using the immunoblotting technique. Our study supports and augments their study, as both the studies revealed the most specific immunoreactive band at the molecular weight of 130 kDa. This band also showed strong immunoreactivity by Western blot against ovine sera experimentally infected with D. dendriticum (Revilla-Nuin et al., Reference Revilla-Nuin, Manga-Gonzalez, Minanbres and Gonzalez-Lanza2005).
Revilla-Nuin et al. (Reference Revilla-Nuin, Manga-Gonzalez, Minanbres and Gonzalez-Lanza2005) also carried out the partial sequencing of 130 kDa protein from both somatic and E/S products, revealing that both are identical at their amino-termini. In agreement with biochemical data, the comparison of this N-terminal sequence on database also revealed a perfect match (10/10 amino acids) with a peptide from D. dendriticum described as a globin (Kunz, Reference Kunz1975). This peptide, consisting of about 58 amino acids length, illustrates an enormous similarity with other peptides described as globins (Rashid et al., Reference Rashid, Van-Hauwaert and Haque1997; Zhang et al., Reference Zhang, Rashid, Haque, Siddiqi, Vinogradov, Moens and Mar1997; Hu et al., Reference Hu, Yan and Shen2003; Sim et al., Reference Sim, Park and Yong2003; unpublished results, see https://blast.ncbi.nlm.nih.gov/Blast.cgi, accession number AAX11352). Taking into consideration the aforementioned reports, Revilla-Nuin et al. (Reference Revilla-Nuin, Manga-Gonzalez, Minanbres and Gonzalez-Lanza2005) postulated a possible relation of this 130 kDa protein with the family of globins. However, the protein band of 130 kDa from D. dendriticum is much larger than the described family of globins from other digenetic trematodes and also no homology was found for internal peptides. On the other hand, N-terminal sequences match properly with the amino-termini of the described Digenea globins. Thus, to summarize, the immunodiagnostic band of 130 kDa could be related to the family of globins, at least at its N-terminal end; however, more investigations are needed to establish its proper sequence and function.
Our studies were partially in accordance with Wedrychowicz et al. (Reference Wedrychowicz, Ducommun, Gorski and Pfister1995) who, by using the SDS–PAGE technique, detected eight to nine polypeptides ranging from 29 to 205 kDa in the surface proteins and 17 bands in SDS soluble or somatic proteins of D. dendriticum extracted with TBS. Moreover, they detected 11–12 major polypeptides ranging between 15 and 205 kDa in culture fluids (E/S) collected before 18 h incubation, and only six polypeptides ranging from 15 to 78 kDa in those collected after 18–24 h. Our results regarding the immunodiagnostic bands of somatic and E/S antigens of D. dendriticum also partially coincide with those of Wedrychowicz et al. (Reference Wedrychowicz, Ducommun, Klockiewicz and Pfister1996), who revealed seven immunodiagnostic polypeptide bands between 28 and 124 kDa in the surface extracts and eight immunodiagnostic polypeptide bands between 21 and 158 kDa in the E/S product using Western blotting with rabbit antisera experimentally infected with D. dendriticum. Simsek et al. (Reference Simsek, Koroglu and Utuk2006) used SDS–PAGE analysis to reveal nine protein bands between the molecular weight ranges of 6 and 66 kDa with Coomassie stain and 14 bands with molecular weight ranges between 6 and 205 kDa with silver stain. Further, they revealed two immunodominant bands with molecular weights of 205 and 98 kDa in the E/S product of D. dendriticum using sera from positive animals, which was in disagreement with our results. Contrary to our studies, Meshgi & Khodaveisi (Reference Meshgi and Khodaveisi2014) showed a major immunoreactive band of 25–28 kDa in both somatic and E/S antigens of D. dendriticum using antisera from rabbits immunized with somatic and E/S antigens of D. dendriticum.
The present study concludes that the protein bands of 84 and 130 kDa in somatic fraction and 130 kDa in E/S fraction were found to give consistent reactions with the sera of infected sheep and can be used for the immunodiagnostic purpose for this economically important parasite. The present study also demonstrates the validity of the immunoblotting technique for the determination of D. dendriticum infections in sheep. However, the purification of these immunoreactive antigens may qualitatively improve the diagnostic value of such a test and may also encourage further studies regarding their vaccine potential.
E/S antigens have proved to be more useful for immunodiagnostic purpose as well as for protection against future infections than the somatic proteins (Parkhouse et al., Reference Parkhouse, Almond, Cabrera and Harnett1987; Dalton & Heffernan, Reference Dalton and Heffernan1989). This is because E/S antigens more commonly get in touch with the host's immune system than somatic antigens. Most of the E/S antigens are the enzymatic proteins in the intestinal caeca of the parasite like cathepsins and others with cytolytic activities, and these enzymes are involved in degrading tissues and facilitating the invasion and migration of the parasite.
Different studies have shown a different number of protein bands using SDS–PAGE analysis, and a variable number and pattern of immunoreactive bands using Western blotting, which could be attributed to the existence of genetic variability in different isolates from different host species, geographical variations, the use of different extraction buffers in protein extraction processes and handling errors. Moreover, antigenic variations, as revealed by a number of helminths as a response to evade host immune response, may contribute to the variation in number and pattern of immunoreactive bands. The other reason could be the presence of both endogenous and host-derived proteins primarily during the isolation of flukes from thawed livers, as suggested by De Vera et al. (Reference De Vera, Sato, Oyong and Claveria2009). Further, the differences can also be attributed to the existence of mixed trematode infections in the sheep, which may contribute to the observation of different immunogenic bands in different studies. Besides, the role of ecological and geographical factors cannot be neglected. Regardless of the difference existing in the number of protein bands or molecular weights of somatic and E/S polypeptides of Dicrocoelium species, the findings of various researchers suggest the existence of antigens with promising diagnostic and protective significance for humans and animals.
Financial support.
Financial support was provided by University Grants Commission, India (serial No. 2061330969; Ref. No. 23/06/2013(i)EU-V).
Conflicts of interest
None.
Ethical standards
The authors assert that all procedures contributing to this work comply with the ethical standards of the relevant national and institutional guides on the care and use of animals.