Introduction
The pyrochlore-supergroup minerals belong to complex oxide minerals with the general formula A 2–mB 2X 6–wY 1–n (Atencio et al., Reference Atencio, Andrade, Christy, Gieré and Kartashov2010; Christy and Atencio, Reference Christy and Atencio2013). The A-site is occupied by large [8]-coordinated cations: Na+, Ca2+, Sr2+, Ba2+, Fe2+, Mn2+, Sn2+, Pb2+, Bi3+, Y3+, Ce3+ (+ other rare earth cations), Sc3+, U4+, Th4+, □ (vacancy), or H2O; the B-site is filled by [6]-coordinated cations, such as W6+, Nb5+, Ta5+, Sb5+, V5+, Ti4+, Sn4+, Zr4+, Hf4+, Si4+, Al3+, Fe3+ and Mg2+; the X-site is occupied by anions O2–, OH– or F–; and the Y-site is occupied by anions O2–, OH– or F–, molecular H2O, □, or very large monovalent cations: K+, Rb+ and Cs+ (Atencio et al., Reference Atencio, Andrade, Christy, Gieré and Kartashov2010). These minerals are divided according to dominant B-site cation occupancy to the classic pyrochlore, microlite and betafite groups (Nb, Ta and Ti dominant), complemented by roméite, elsmoreite, ralstonite and coulsellite groups (Hogarth, Reference Hogarth1977; Atencio et al., Reference Atencio, Andrade, Christy, Gieré and Kartashov2010, Reference Andrade, Yang, Atencio, Downs, Chukanov, Lemée-Cailleau, Persiano, Goeta and Ellena2017).
Members of the pyrochlore and betafite groups occur as magmatic-to-late hydrothermal and supergene or metamorphic accessory components in very diverse geological environments, principally in carbonatites, nepheline syenites and related rocks (e.g. Chakhmouradian and Mitchell, Reference Chakhmouradian and Mitchell2002; Melgajero et al., Reference Melgarejo, Costanzo, Bambi, Gonçalves and Neto2012; Walter et al., Reference Walter, Parsapoor, Braunger, Marks, Wenzel, Martin and Markl2018; Amores-Casals et al., Reference Amores-Casals, Gonçalves, Melgarejo and Martí2020; Dey et al., Reference Dey, Mitchell, Bhattacharjee, Chakrabarty, Pal, Pal and Sen2021; Zaitsev et al., Reference Zaitsev, Spratt, Shtukenberg, Zolotarev, Britvin, Petrov, Kuptsova and Antonov2021), rarely in alkaline and rare-element granites to pegmatites (Johan and Johan, Reference Johan and Johan1994; Lumpkin and Ewing, Reference Lumpkin and Ewing1996; De Vito et al., Reference De Vito, Pezzotta, Ferrini and Aurisicchio2006; Prol-Ledesma et al., Reference Prol-Ledesma, Melgarejo and Martin2012; Duran et al., Reference Duran, Seydoux-Guillaume, Bingen, Gouy, De Parseval, Ingrin and Guillaume2016; Atencio et al., Reference Atencio, Andrade, Bindi, Bonazzi, Zoppi, Stanley and Kristiansen2018; Siegel et al., Reference Siegel, Vasyukova and Williams-Jones2018), kimberlites (Sharygin et al., Reference Sharygin, Sobolev and Channer2009), hydrothermal veins in granite contact aureoles (Lumpkin et al., Reference Lumpkin, Gieré, Williams, McGlinn and Payne2017), or lunar rocks (Meyer and Young, Reference Meyer and Young1988; Mokhov et al., Reference Mokhov, Kartashov, Bogatikov, Ashikhmina, Magazina and Koporulina2008).
In contrast microlite-group minerals form almost exclusively in rare-element granitic pegmatites of the LCT (Li–Ta–Cs) family. They precipitate during various pegmatite stages from late-magmatic/early subsolidus crystallisation associated usually with albitisation, to moderate and low-temperature hydrothermal overprints (e.g. Lumpkin et al., Reference Lumpkin, Chakoumakos and Ewing1986; Černý et al., Reference Černý, Novák and Chapman1992; Spilde and Shearer, Reference Spilde and Shearer1992; Lumpkin, Reference Lumpkin1998; Tindle and Breaks, Reference Tindle and Breaks1998; Novák and Černý, Reference Novák and Černý1998; Novák et al., Reference Novák, Uher, Černý and Siman2000, Reference Novák, Černý and Uher2003; Guastoni et al., Reference Guastoni, Diella and Pezzotta2008; Chudík et al., Reference Chudík, Uher, Gadas, Škoda and Pršek2011; Demartis et al., Reference Demartis, Melgarejo, Colombo, Alfonso, Coniglio, Pinotti and D'Eramo2014; Galliski et al., Reference Galliski, Márquez-Zavalía, Černý and Lira2016; Szuszkiewicz et al., Reference Szuszkiewicz, Pieczka, Szełęg, Turniak, Ilnicki and Nejbert2016; Andrade et al., Reference Andrade, Yang, Atencio, Downs, Chukanov, Lemée-Cailleau, Persiano, Goeta and Ellena2017; Loun et al., Reference Loun, Novák, Cempírek, Škoda, Vašinová–Galiová, Prokeš, Dosbaba and Čopjaková2018; Kasatkin et al., Reference Kasatkin, Britvin, Peretyazhko, Chukanov, Škoda and Agakhanov2020). Lumpkin and Ewing (Reference Lumpkin and Ewing1992) recognised two principal stages of alteration of primary microlite in granitic pegmatites: the first takes place at relatively high temperatures (primary hydrothermal alteration) and is best described by the exchange vectors ANaYFACa–1YO–1 and A□Y□ACa–1YO–1; the second takes place at relatively low temperature (up to that of subsurface weathering) with ANaYF A□–1Y□–1, ACaYO A□–1Y□–1 and ACaXO A□–1X□–1 substitutions. Secondary alteration generates a significant loss of A-site elements (especially Na and Ca) and increases A-site vacancies and hydration of the mineral (Ewing, Reference Ewing1975; Loun et al., Reference Loun, Novák, Cempírek, Škoda, Vašinová–Galiová, Prokeš, Dosbaba and Čopjaková2018). The precipitation of microlite-group minerals as late-magmatic to post-magmatic (especially hydrothermal) alteration products after primary Nb–Ta phases has been examined in numerous rare-element granitic pegmatites, from less-evolved beryl–columbite–(phosphate) to complex Li–Cs–Ta rich mineralisation where the high activity of volatile elements, such as F, B and P, together with H2O is typical (e.g. Lumpkin et al., Reference Lumpkin, Chakoumakos and Ewing1986; Tindle and Breaks, Reference Tindle and Breaks1998; Uher et al., Reference Uher, Černý, Chapman, Határ and Miko1998; Chudík et al., Reference Chudík, Uher, Gadas, Škoda and Pršek2011; Gonçalves et al., Reference Gonçalves, Melgarejo, Alfonso, Amores, Paniagua, Neto, Morais and Camprubí2019).
We have investigated beryl–columbite pegmatites from the Maršíkov District, Bohemian Massif which are relatively poor in volatile elements (F, B or P). They contain accessory Nb–Ta oxide minerals (maily members of CGM), which have been the object of several investigations (Černý et al., Reference Černý, Novák and Chapman1992, Reference Černý, Novák and Chapman1995; Novák et al., Reference Novák, Černý and Uher2003; Novák and Dosbaba, Reference Novák and Dosbaba2006; Chládek et al., Reference Chládek, Uher and Novák2020), though these have had only minor focus on the microlite-group minerals replacing columbite–tantalite. The microlite-group minerals, which can form a variety of textural and compositional types, precipitated at least three different stages, which reflect complex subsolidus processes in the host pegmatite. Consequently, the objective of this study is to characterise textural, paragenetic and compositional variations of microlite-group minerals for a better understanding of the complex post-magmatic evolution of the pegmatites.
Geological setting
The Schinderhübel I, Scheibengraben and Bienergraben beryl–columbite granitic pegmatites are situated near Maršíkov village, Šumperk County in northern Moravia, Czech Republic. These pegmatites are spatially and geologically closely related and belong to the most fractionated bodies of the Maršíkov District. The pegmatites are situated in the southern part of the Neoproterozoic to Devonian Desná Dome (Cháb et al., Reference Cháb, Fediuková, Fišera, Novotný and Opletal1990; Novák and Rejl, Reference Novák and Rejl1993; Schulmann et al., Reference Schulmann, Oliot, Košuličová, Montigny and Štípská2014), a part of the Silesian Domain, North-East Bohemian Massif (Fig. 1). The Desná Dome consists of crystalline basement including ortho/paragneisses of the Neoproterozoic protolith (570–650 Ma, Kröner et al., Reference Krönner, O´Brien, Nemchin and Pidgeon2000) and overlaying Palaeozoic rocks, mainly metaquartzites, metaconglomerates, metapelites and marbles, locally intruded by Middle Devonian (Givetian) (back)-arc affinity metavolcanic rocks of the Sobotín Massif (Janoušek et al., Reference Janoušek, Aichler, Hanžl, Gerdes, Erban, Žáček, Pecina, Pudilová, Hrdličková, Mixa and Žáčková2014). The Silesian Domain underwent Variscan medium-pressure, Barrovian-type metamorphism at P ≈ 500–700 MPa and T ≈ 540–650°C (René, Reference René1983; Cháb et al., Reference Cháb, Fediuková, Fišera, Novotný and Opletal1990; Košuličová and Štípská, Reference Košuličová and Štípská2007).

Fig. 1. Geological map of the Silesian Domain and the Maršíkov District with individual pegmatite occurrences (modified after Cháb et al., Reference Cháb, Stráník and Eliáš2007 and on-line map application of the Czech Geological Survey 2019, Chládek et al., Reference Chládek, Uher and Novák2020).
The Maršíkov District includes several tens of granitic pegmatites of variable size, 1 to 10 m in thickness and up to 100 m in length, forming elongated dykes or slightly irregular lensoid bodies embedded in the host biotite-amphibole gneisses and/or amphibolites of the Sobotín Massif. Radiometric ages of the pegmatites from Maršíkov District and their potential parental granitic source(s) are not known; however, their Variscan Carboniferous age might be analogous to that of pegmatitic leucogranite at Čertovy Kameny hill near Jeseník (334 Ma U–Pb zircon radiometric ages; Hegner and Kröner, Reference Hegner, Kröner, Franke, Haak, Oncken and Tanner2000), which is also consistent with ages of older granitic plutons: Kłodzko-Złoty Stok, Jawornik, Strzelin, and Kudowa plutons (350–330 Ma, Mikulski et al., Reference Mikulski, Williams and Bagiński2013) in the adjacent Sudetic block on the North-East margin of the Bohemian Massif in Poland. Younger, late-Variscan, Early Permian magmatic activity documents the granodiorites to granites of the Žulová Pluton with the emplacement age of 292 ± 4 or 291 ± 5 Ma (LA ICP MS U–Pb zircon radiometric age determination; Laurent et al., Reference Laurent, Janoušek, Magna, Schulmann and Míková2014). Some granitic pegmatites were affected by a metamorphic overprint with local replacement of beryl by chrysoberyl and fibrolitic sillimanite (Dostál, Reference Dostál1966; Franz and Morteani, Reference Franz and Morteani1984; Černý et al., Reference Černý, Novák and Chapman1992). This overprint is possibly connected with Upper Carboniferous to Permian (~310–260 Ma) low pressure / high temperature tectono-metamorphic reactivation of the Desná Dome (Schulmann et al., Reference Schulmann, Oliot, Košuličová, Montigny and Štípská2014).
Internal structure, petrography and mineralogy of pegmatites
The granitic pegmatites investigated comprise the following textural-paragenetic units developed from the contact inwards (Fig. 2): (1) an aplitic unit (Kfs + Ab + Qtz + Bt) (≤2 cm thick), evolving to (2) a prevailing coarse-grained, muscovite rich unit (Kfs + Ab + Qtz + Ms). The locally present (3) graphic unit (Kfs + Qtz) gradually evolves into (4) a blocky K-feldspar unit. A quartz core (5) is only developed locally. Small nests of the cleavelandite unit (6) are common in the blocky K-feldspar and coarse-grained unit. Metasomatic replacement of pre-existing units by saccharoidal albite is widespread (Pokorný and Staněk, Reference Pokorný and Staněk1951; Dostál, Reference Dostál1966; Novák et al., Reference Novák, Černý and Uher2003).
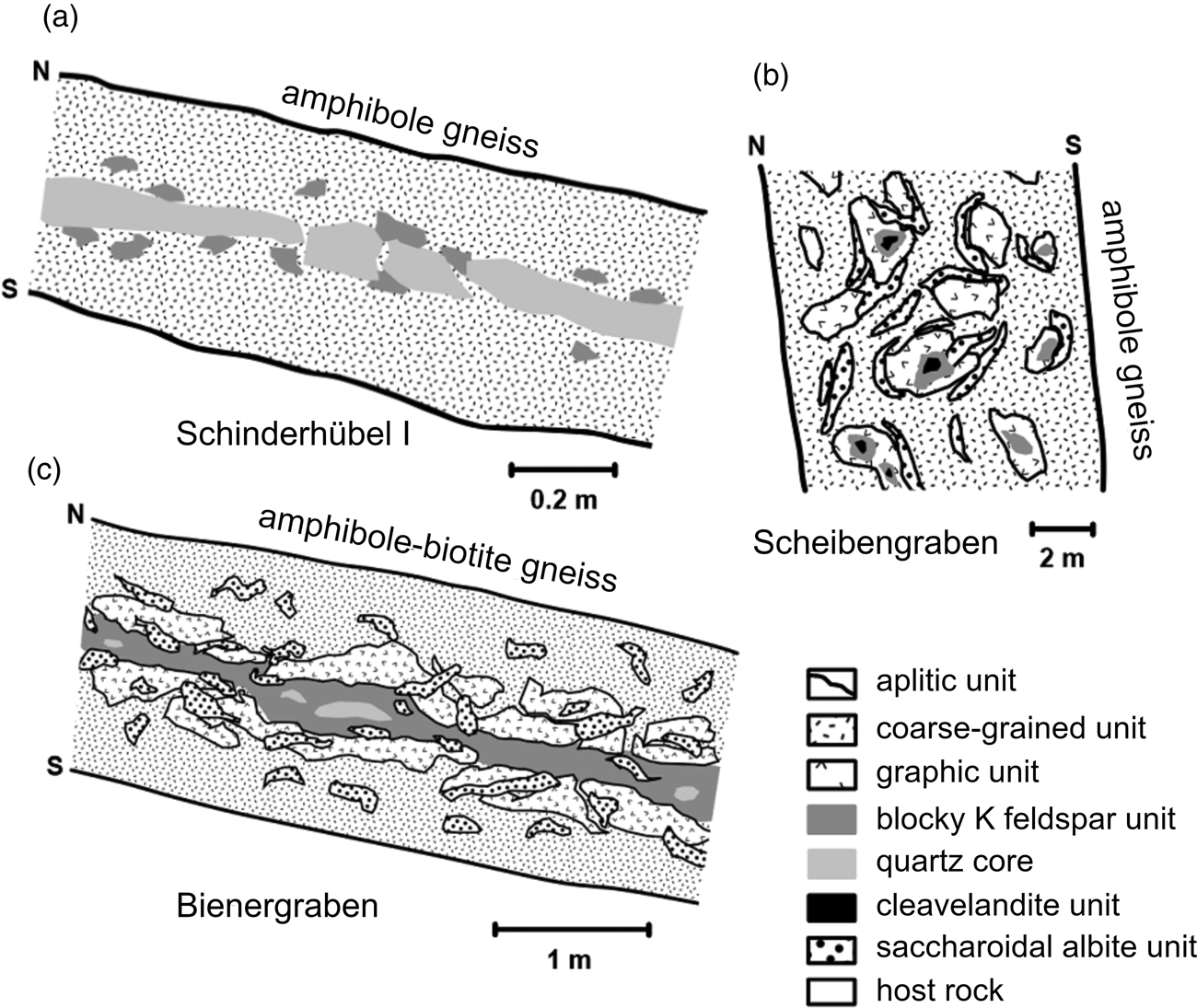
Fig. 2. Idealised cross-sections of the pegmatites: (a) Maršíkov – Schinderhübel I (Chládek et al., Reference Chládek, Uher and Novák2020, modified); (b) Maršíkov – Scheibengraben (Novák et al., Reference Novák, Černý and Uher2003, modified); (c) Sobotín – Bienergraben (Chládek et al., Reference Chládek, Uher and Novák2020, modified).
The Schinderhübel I pegmatite dyke, up to 0.5 m in thickness, consists of a coarse-grained muscovite-rich unit, prevailing over poorly-evolved graphic and blocky K-feldspar units (Fig. 2a). A small quartz core is developed in the central part of this dyke and the albite unit is distributed irregularly within the coarse-grained unit. The metamorphic overprint is relatively weak in comparison with the neighbouring strongly foliated chrysoberyl + sillimanite-bearing Schinderhübel III pegmatite (Dostál, Reference Dostál1966; Franz and Morteani, Reference Franz and Morteani1984; Černý et al., Reference Černý, Novák and Chapman1992); however, rare chrysoberyl and sillimanite are present. The largest pegmatite, Scheibengraben (e.g. Pokorný and Staněk, Reference Pokorný and Staněk1951; Novák, Reference Novák1988; Novák et al., Reference Novák, Černý and Uher2003) forms a ~10 m thick lensoid body where the individual pegmatite units are distributed irregularly (Fig. 2b). Saccharoidal albite has extensively replaced the other pegmatite units except for the minor coarse-grained albite (cleavelandite) unit, forming small nests neighbouring blocky K-feldspar. This pegmatite body has only been affected mildly by deformation and metamorphism, mostly along its contacts with the host amphibolite gneiss. The Bienergraben pegmatite (Kruťa, Reference Kruťa1966) is a ≤2 m thick dyke characterised by a symmetrically zoned internal structure with common nests of saccharoidal albite and a moderate degree of deformation (Fig. 2c).
All the pegmatites investigated contain very similar mineral assemblages and they correspond to the LCT family and beryl–columbite subtype of rare-element granitic pegmatites (sensu stricto Černý and Ercit, Reference Černý and Ercit2005). Accessory minerals include common garnet (almandine–spessartine), zircon, fluorapatite, gahnite and uraninite together with other, mostly rare, minerals (Pokorný and Staněk, Reference Pokorný and Staněk1951; Dostál, Reference Dostál1966; Kruťa, Reference Kruťa1966; Chládek et al., Reference Chládek, Uher and Novák2020; Dolníček et al., Reference Dolníček, Nepejchal, Sejkora, Ulmanová and Chládek2020a,Reference Dolníček, Nepejchal and Novákb). Beryl is the only primary magmatic Be mineral together with rare metamorphic chrysoberyl and common secondary bavenite and bohseite. Niobium–tantalum oxide minerals are represented by relatively common columbite–tantalite and microlite, whereas Nb–Ta-rich rutile, titanite, tapiolite-(Fe), rynersonite, and fersmite are less common (Čech, Reference Čech1973; Černý et al., Reference Černý, Novák and Chapman1992, Reference Černý, Novák and Chapman1995; Novák et al., Reference Novák, Černý and Uher2003; Novák and Dosbaba, Reference Novák and Dosbaba2006; Chládek et al., Reference Chládek, Uher and Novák2020). Most granitic pegmatites in the Maršíkov District are relatively poor in B, F and P (Novák, Reference Novák1988, Reference Novák2005; Novák et al., Reference Novák, Černý and Uher2003), only the most fractionated Scheibengraben body also contains accessory schorl, topaz and triplite (Pokorný and Staněk, Reference Pokorný and Staněk1951).
Methods
Compositions of Nb–Ta oxide minerals were determined using a Cameca SX100 electron probe micro-analyser (EPMA) in wavelength-dispersion mode at the Dionýz Štúr State Geological Institute, Bratislava; textural patterns were studied in back-scattered electron (BSE) mode using the same apparatus. Measurements were performed with the following analytical conditions: beam diameter of 1−2 μm; an acceleration potential of 15 kV; sample current of 40 nA measured on a Faraday cup; and a counting time of 20 s. Synthetic and natural standards used and detection limits for the individual elements are summarised in Table 1. Concentrations of other measured elements (e.g. Mg, Pb, Zn, Sr, Y, La, Nd, Bi and P) are very low, close to, or below the detection limits of EPMA. Measured data were reduced using the PAP routine (Pouchou and Pichoir, Reference Pouchou, Pichoir and Armstrong1985). The structural formulae of columbite-group minerals were normalised on the basis of 24 oxygen atoms and 12 cations using an Fe2+ and Fe3+ charge-balance calculation according to the procedure of Ercit (Reference Ercit1994). The formulae of microlite (Mic) and rare pyrochlore (Pcl) were normalised on the basis of B-site cations (W + Ta + Nb + Ti + Si + Zr + Al) = 2 atoms per formula unit (apfu) and by considering that Sntot is present as Sn2+, Fetot is present as Fe2+ and Utot as U4+ (Atencio et al., Reference Atencio, Andrade, Christy, Gieré and Kartashov2010). We also included Si4+ and Al3+ cations in the sixfold-coordinated B-site of the pyrochlore structure following the recent approved nomenclature of pyrochlore-supergroup minerals (Atencio et al., Reference Atencio, Andrade, Christy, Gieré and Kartashov2010). However, the presence of Si in the pyrochlore structure (isomorphic constituent or non-structural impurity) remains the subject of controversial discussion (Chakhmouradian and Mitchell, Reference Chakhmouradian and Mitchell2002; Bonazzi et al., Reference Bonazzi, Bindi, Zoppi, Capitani and Olmi2006; Andrade et al., Reference Andrade, Atencio, Chukanov and Ellena2013; Dumańska-Słowik et al., Reference Dumańska-Slowik, Pieczka, Tempesta, Olejniczak and Heflik2014). In contrast, Al3+ is an essential cation at the B-site in hydrokenoralstonite and fluornatrocoulsellite, two recently redefined members of the pyrochlore-supergroup minerals (Atencio et al., Reference Atencio, Andrade, Neto and Pereira2017). The structural formulae of fersmite were normalised on the basis of 3 cations and 6 oxygen atoms.
Table 1. EPMA conditions for the Nb–Ta minerals measured.

d.l. – detection limit = 3σ (in ppm). Mic – microlite; Pcl – pyrochlore.
The microRaman in situ analyses (μRS) were undertaken using a LabRAM-HR Evolution (Horiba Jobin-Yvon) spectrometer system with a Peltier cooled CCD detector and Olympus BX-41 microscope (Department of Geological Sciences, Masaryk University, Brno). A blue diode laser (473 nm) was used to obtain Raman spectra in the range of 50−4000 cm–1. The acquisition time was 10 s per frame. The Raman spectra were processed using PeakFit 4.1.12 (Systat Software, San Jose, CA); absorption bands were fitted by the Lorentz function with automatic background correction and Savitsky-Golay smoothing.
Results
Paragenesis and internal texture of Nb–Ta minerals
Columbite-group minerals (CGM) were investigated predominantly from the blocky K-feldspar unit in the Scheibengraben and Bienergraben pegmatites and from the muscovite-rich coarse-grained unit in the Schinderhübel I pegmatite. The CGM are common, especially in albitised portions of these two units and are associated closely with muscovite, almandine–spessartine, gahnite, zircon, beryl, fluorapatite and uraninite. Primary magmatic oscillatory zoning of CGM is commonly developed and is replaced locally by convolute oscillatory zoned-to-patchy domains of secondary (early hydrothermal) CGM (Figs 3, 4; Chládek et al., Reference Chládek, Uher and Novák2020).
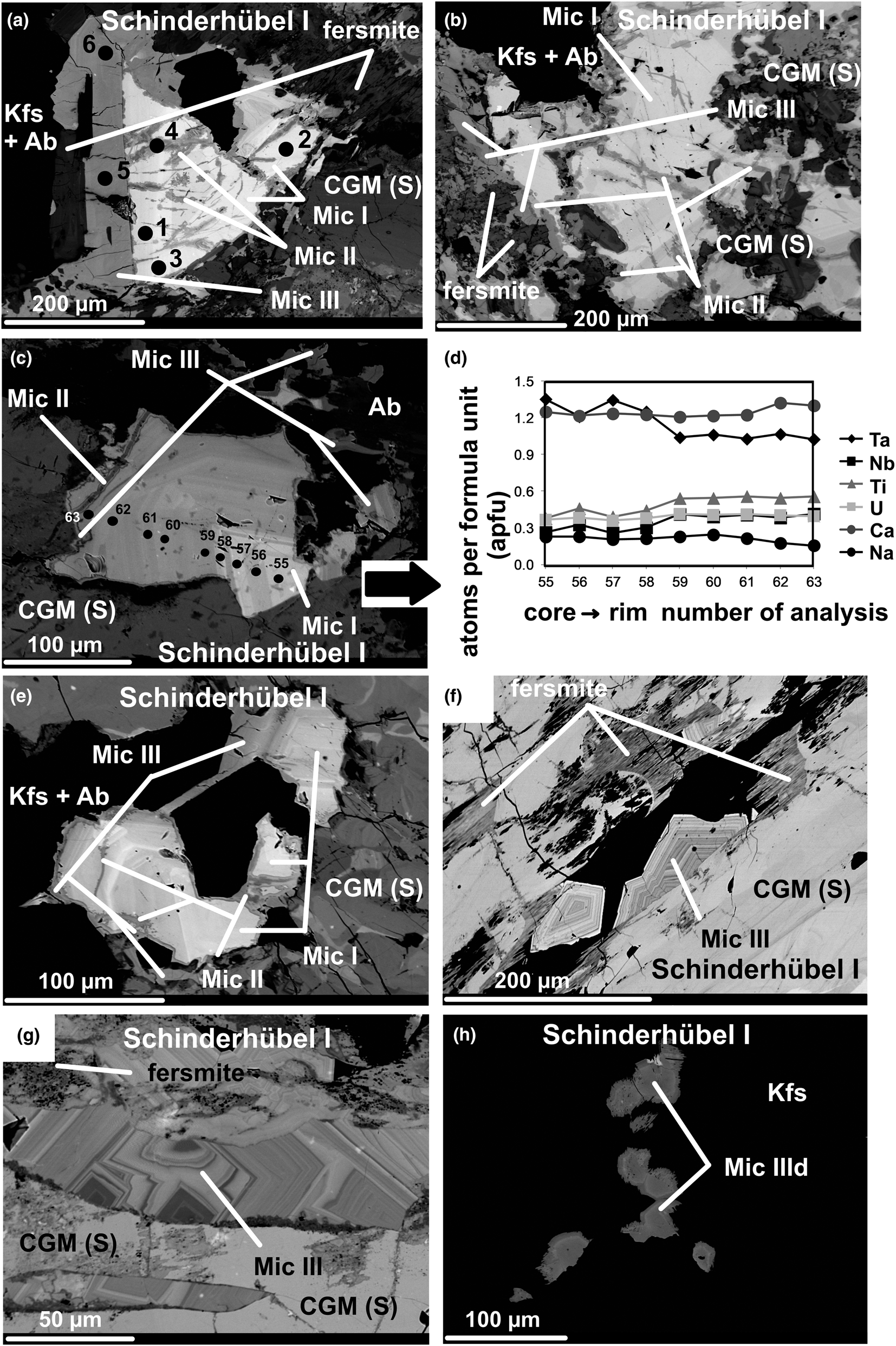
Fig. 3. Back-scattered electron (BSE) image of Nb–Ta minerals from the pegmatites: (a) three microlite generations replacing secondary CGM: core domains of Mic I, crosscut by irregularly zoned Mic II, slightly zoned rims of darker Mic III together with tabular fersmite; Raman analysed points: 1−3 Mic I; 4 Mic II; 5 and 6 Mic III (Schinderhübel I); (b) rather homogeneous domains of Mic I characterised by a high porosity and fracturing with irregularly zoned veinlets of Mic II crosscutting Mic I, rims of darker homogeneous Mic III associated with tabular fersmite; microlite types and fersmite replace secondary patchy zoned CGM (Schinderhübel I); (c) oscillatory zoned Mic I with EMPA profile, crosscut by irregularly zoned veinlets of Mic II, rims of indistinctly zoned Mic III; microlite types replace secondary patchy zoned CGM (Schinderhübel I); (d) plot of elemental concentrations in atoms per formula unit (apfu) from core to rim of oscillatory zoned Mic I illustrated in Fig. 3c (Schinderhübel I); (e) oscillatory zoned Mic I crosscut by irregularly zoned veinlets of Mic II, rims of indistinctly zonal Mic III; microlite types replace secondary patchy zoned CGM (Schinderhübel I); (f) oscillatory zoned euhedral Mic III accompanied by tabular dark fersmite on a crack of secondary patchy zoned CGM (Schinderhübel I); (g) oscillatory zoned euhedral Mic III on a crack of secondary patchy zoned CGM (Schinderhübel I); (h) euhedral zoned grains of Mic IIId in crack-fillings in K-feldspar (Schinderhübel I). Abbreviations: Ab – albite, Kfs – K feldspar; CGM (S) – columbite-group minerals (secondary).

Fig. 4. BSE images of Nb–Ta minerals from the pegmatites: (a) veinlets of Mic III crosscutting oscillatory zoned primary CGM replaced by patchy domains of secondary CGM (Scheibengraben); (b) part of the Fig. 4a: veinlets of Mic III crosscutting both oscillatory zoned primary CGM and patchy domains of secondary CGM (Scheibengraben); (c) veinlets of Mic III penetrating oscillatory zoned CGM; Mic IIId chains composed of anhedral grains at the cracks of K-feldspar (Scheibengraben); (d) Mic IIId chains composed of anhedral grains on cracks of K-feldspar and quartz (Scheibengraben); (e) euhedral oscillatory zoned Mic III in vugs and cracks of the primary and secondary CGM (Bienergraben); (f) oscillatory zoned Mic III on cracks of the primary CGM (Bienergraben). Abbreviations: Ab – albite, Kfs – K feldspar; CGM (S) – columbite-group minerals (secondary); CGM (P) – columbite-group minerals (primary).
Two principal textural types of microlite (pyrochlore) were distinguished in the sense of Novák et al. (Reference Novák, Čopjaková, Dosbaba, Galiová, Všianský and Staněk2015): a proximal microlite directly replacing the CGM, commonly along cracks; and a near distal type that forms aggregates of small grains within cracks in host K-feldspar and quartz, close to grains of replaced CGM (Figs 3, 4). The detailed textural relationships and compositional variations at the individual pegmatites show four distinct types of microlite- and rarely, pyrochlore-group minerals. Proximal Mic I to Mic III replace both primary and secondary CGM (Figs 3, 4), whereas early Mic I and II are replaced by late Mic III; and by distal Mic IIId in cracks of host K-feldspar and quartz. Only the Schinderhübel I pegmatite contained all the types of microlite described (Fig. 3a–h).
Microlite I forms subhedral grains replacing primary and secondary CGM along fractures (Fig. 3a−c, e); it constitutes homogeneous (Fig. 3a,b) to oscillatory zoned (Fig. 3c,e) corroded domains, characterised by indistinct compositional zonation (Fig. 3d). Microlite I is cut and replaced locally by irregularly zoned veinlets of Mic II (Fig. 3a−c, e). Microlite III forms late rims and homogeneous to slightly oscillatory zoned euhedral-to-subhedral grains surrounding and replacing Mic I−II (Fig. 3a–c, e) and CGM in cracks (Fig. 3f,g). Microlite III is associated locally with fibrous aggregates of fersmite (Fig. 3a,b,f,g). Thin chains composed of euhedral-to-anhedral grains of distal microlite IIId (Mic IIId) were identified occasionally on cracks in K-feldspar grains close to CGM (≤300 μm, Fig. 3h). Relatively homogeneous Mic III veinlets (≤1 mm in length) commonly crosscut CGM crystals (Fig. 4a–c) at Scheibengraben. Distal Mic IIId forms anhedral grains grouped into chains in cracks in quartz and K-feldspar close to CGM (Fig. 4c,d). At Bienergraben, similar to Mic III from Schinderhübel I, oscillatory-to-homogeneous euhedral-to-subhedral Mic III fills cavities and occurs in cracks in CGM (Fig. 4e,f),
Composition
Columbite–tantalite
Both primary magmatic and secondary CGM are characterised by variable evolution in one or both Mn/(Mn+Fe) and Ta/(Ta+Nb) ratios from columbite-(Fe) to tantalite-(Mn) (Fig. 5; Table 2; Chládek et al., Reference Chládek, Uher and Novák2020). In the Schinderhübel I pegmatite, secondary CGM are compositionally more variable than primary CGM, the opposite to their behaviour at Scheibengraben (compare with Fig. 5a,b). Primary and secondary compositions are poor in minor elements except for Ti (≤2.0 wt.% TiO2) and Mg (≤0.6 wt.% MgO) in secondary CGM from Schinderhübel I. Calculated Fe3+ values are up to 3.0 wt.% Fe2O3 in both primary and secondary CGM (Table 2).

Fig. 5. Compositions of primary and secondary CGM in a classification diagram for CGM: (a) Schinderhübel I; (b) Scheibengraben; (c) Bienergraben. Abbreviations: CGM (P) / (S) – primary (magmatic) / secondary (hydrothermal) columbite-group minerals.
Table 2. Representative compositions of primary (P) and secondary (S) CGM from Maršíkov District.*

* ‘–’ content of the element is below the detection limit. Abbreviations: Schl — Schinderhübel I, Sch — Scheibengraben, Bn — Bienergraben.
Microlite- and pyrochlore-group minerals
In the Schinderhübel I pegmatite (Fig. 6; Table 3), Ca (≤1.76 apfu) is typically the dominant cation in the A-site of most of the microlite compositions (Fig. 6a,b). The contents of Na and U decrease from Mic I (≤0.40 apfu Na, ≤0.48 apfu U) through Mic II (≤0.28 apfu Na, ≤0.47 apfu U) to Mic III (≤0.09 apfu Na, ≤0.10 apfu U) and Mic IIId (≤0.06 apfu Na, ≤0.01 apfu U). The Ta/(Ta+Nb) ratio is similar in Mic I (0.61−0.84) and Mic II (0.57−0.81); increases in Mic III (0.74−0.93), and is slightly higher in Mic IIId (0.79−0.81). However, concentrations of Ti decrease in the same manner from Mic I and Mic II (≤0.68 apfu and ≤0.78 apfu) to Mic III (≤0.35 apfu) and Mic IIId (≤0.24 apfu, Fig. 6c). Trace concentrations of Si (≤0.01 apfu), Zr (≤0.02 apfu) are present in Mic III and Mic IIId (Table 3), whereas W and Nb are more-or-less constant in all microlite types (Table 3). Microlite III is distinctly enriched in F (≤0.47 apfu) compared to Mic I (≤0.14 apfu), Mic II (≤0.18 apfu) and also Mic IIId (≤0.14 apfu, Fig. 6d; Table 3). The associated fersmite has a relatively low Ta/(Ta+Nb) ratio (0.11−0.43) in comparison with Mic I−III, ~1.0 apfu Ca and minor contents of REE and U (Table 4).

Fig. 6. Ternary classification diagrams and F–Na plot for microlites in Schinderhübel I: (a) A-site (Na–Ca–Other cations); (b) A-site (Na–Ca–vacancy); (c) B-site (Nb–Ta–Ti); (d) F vs. Na plot; note, ‘Other cations’ represent Th, Sb, Sc, Ce, Fe, Mn and Sn in the A-site.
Table 3. Representative compositions of microlite (Mic) from Schinderhübel I.*

* ‘–’ content of the element is below detection limit. Abbreviations: Schl – Schinderhübel I; A, B – structural positions of pyrochlore structure; Mic I, II, III, IIId – microlite types. End-members of pyrochlore-supergroup minerals (molecule %): pyrochlore (Nb#), microlite (Ta#) and betafite (Ti#).
Table 4. Representative compositions of fersmite from Schinderhübel I.*

* ‘–’ content of the element is below the detection limit. Abbreviations: Schl — Schinderhübel I.
At Scheibengraben, Mic III and IIId (Fig. 4a–d) are similar to Mic III from Schinderhübel I (Figs 6, 7; Table 5), only concentrations of Na are slightly higher (≤0.32 apfu) and locally also Si (≤0.33 apfu, Table 5). Euhedral-to-subhedral oscillatory zoned Mic III from Bienergraben is similar to Mic III and IIId in Schinderhübel I and Scheibengraben (Figs 6–8; Table 5); however, it differs in having a greater variation in Ta/(Ta+Nb) = 0.44−0.94 (Fig. 8a–c; Table 5).

Fig. 7. Ternary classification diagrams and F–Na plot for microlites in Scheibengraben: (a) A-site (Na–Ca–Other cations); (b) A-site (Na–Ca–vacancy); (c) B-site (Nb–Ta–Ti); (d) F vs. Na plot; note ‘Other cations’ represent Th, Sb, Sc, Ce, Fe, Mn and Sn in the A-site.

Fig. 8. Ternary classification diagrams and F-Na plot for microlites and pyrochlore in Bienergraben: (a) A-site (Na–Ca–other cations); (b) A-site (Na–Ca–vacancy); (c) B-site (Nb–Ta–Ti); (d) F versus Na plot. Note, 'Other cations' represent Th, Sb, Sc, Ce, Fe, Mn and Sn in the A-site.
Table 5. Representative compositions of microlite (Mic) and pyrochlore (Pcl) from Scheibengraben and Bienergraben.*

* ‘–’ content of the element is below detection limit. Abbreviations: Sch – Scheibengraben, Bn – Bienergraben. A-, B – structural positions of pyrochlore structure. Mic III, IIId, Pcl III – microlite (Pcl – pyrochlore) types and at individual pegmatites. End-members of pyrochlore supergroup minerals (molecule %): pyrochlore (Nb#), microlite (Ta#) and betafite (Ti#).
The compositions investigated belong to the microlite group, with fewer examples belonging to the pyrochlore group at the Bienergraben pegmatite, with Ca cations dominant in the A-site (Figs 6−8; Tables 3 and 5). Analytical totals (91.0 to 98.6 wt.%), as well as total O (6.03–6.90 apfu) and F (0.00–0.43 apfu) indicate the presence of oxycalciomicrolite, fluorcalciomicrolite and hydroxycalciomicrolite. However, some compositons might represent zero-valence dominant members at the A- and Y- sites (Atencio et al., Reference Atencio, Andrade, Christy, Gieré and Kartashov2010).
Compositional trends in the individual types of microlite from the Schinderhübel I pegmatite were examined in detail and correlations between the individual elements and A-site vacancy are illustrated in Fig. 9. An inverse correlation between Ca and Na at Mic I (Fig. 9a), and only trace concentrations of F in most compositions (Fig. 9b) suggest the dominant substitution is (1): ANaY(OH)ACa–1YO–1. The correlation vectors between U and Ti at ~0.5 (Fig. 9c), Ti/(Nb+Ta) ≈ –1 (Fig. 6c) and the A-site vacancy versus U close to –1 (Fig. 9d) can be expressed via substitution (2): AU0.5BTiYOA□–0.5B(Ta,Nb)–1Y(OH)–1. Uranium was considered as U4+ although U6+ as the uranyl group (UO2)2+ has also been found in microlite, for example from the Harding pegmatite (Lumpkin et al., Reference Lumpkin, Chakoumakos and Ewing1986). However, substitution 2 has only minor importance and these data are not as conclusive as for substitution (1). The compositions of Mic II show an inverse correlation between the A-site vacancy and Ca (Fig. 9e) and trace concentrations of Na (Fig. 9a). These data suggest substitution (3): ACaYOA□–1Y□–1; however, these data are less conclusive, mainly due to the more heterogeneous composition of Mic II (Fig. 6a,b). The very unusual composition of Mic III (+ IIId) can be expressed by substitution (4): ACa0.5YF0.5Y(OH)0.5 A□–0.5Y□–1 as there is a positive correlation for Ca versus F (Fig. 9b), an almost total absence of Na, and low contents of Ti and U (Fig. 9a,c,f).

Fig. 9. Correlations of elemental concentrations in atoms per formula unit (apfu) in Mic I–III and IIId from Schinderhübel I: (a) Ca vs. Na; (b) Ca vs. F; (c) U vs. Ti; (d) U vs. vacancy in the A-site; (e) Ca vs. vacancy in the A-site; (f) Ca vs. U.
Raman spectrometry
Raman spectra of Mic I−III from the Schinderhübel I pegmatite display very broad bands with large full width at half maximum (FWHM) and extensive overlaps (Fig. 10a). These bands can be attributed to specific modes according to Geisler et al. (Reference Geisler, Berndt, Meyer, Pollok and Putnis2004) (Fig. 10; Table 6): (a) T2g modes in the region of 196−259 cm–1 assigned to A–Y stretching and X–A–Y bending and the 637−802 cm–1 region for B–X octahedra stretching, and two types of band with ambiguous assignment but probably forbidden or combination mode and vibrational mode in an amorphous structure; (b) E g modes between 286 and 328 cm–1 assigned to X–B–X bending; (c) A 1g modes in the region of 532−540 cm–1 assigned to B–X octahedra stretching. Features such as the large FWHM, overlapping bands and the highest intensity of the 773–802 cm–1 bands probably associated with a vibrational mode in an amorphous structure, indicate the low-ordered crystalline to amorphous state of the microlites investigated. The presence of low-intensity (OH)– bands was observed only in two spectra of Mic I (Fig. 10b; Table 6, spectrum 2) and III (Fig. 10b; Table 6, spectrum 5). All spectra display variable but relatively intensive luminescence resulting in the increased background.

Fig. 10. Representative Raman spectra of Mic I−III from Schinderhübel I: (a) 100−1200 cm–1 region; (b) 3000−4000 cm–1 region; abbreviation: 1 (I) is number of analysis (microlite type).
Table 6. Raman bands and their assignments in Mic I, II and III from Schinderhübel I pegmatite (in cm–1) compared to unaltered microlite from Geisler et al. (Reference Geisler, Berndt, Meyer, Pollok and Putnis2004).*

* Abbreviation: 1 (Mic I) – analysis (microlite type).
Discussion
Compositional variability of microlite
Proximal microlites from the Schinderhübel I pegmatite demonstrate the evolution from Mic I, with high Na, moderate Ca and low A-site vacancy, to Mic II characterised by low Na, variable A-site vacancy and slightly elevated Ca (Fig. 6; Table 3); both have high but variable U and very low contents of F. The Ta/(Ta+Nb) ratio is relatively constant whereas Ti is variable in both types. Compared to Mic I and II, Mic III differs significantly in having higher Ca, low A-site vacancy, higher Ta/(Ta+Nb), lower Ti and generally with high, but variable F. The distal Mic IIId is generally similar to Mic III, it differs only in fewer compositional variations, perhaps due to fewer analysed spots.
Oxygen is a dominant anion in the compositions with high totals (Tables 3, 5). Molecular water was not determined using μRS, only the local occurrence of a hydroxyl anion in the Y-site of Mic I and III was indicated (Fig. 10; Table 6). Hence, mutual relationships between Na and Ca vs. F (Figs 6−9) in Mic I−III (+ IIId) illustrate different compositional trends in these sites. In the Schinderhübel I pegmatite, two distinct trends are evident (Fig. 6d), although both differ from data commonly published (e.g. Lumpkin et al., Reference Lumpkin, Chakoumakos and Ewing1986; Loun et al., Reference Loun, Novák, Cempírek, Škoda, Vašinová–Galiová, Prokeš, Dosbaba and Čopjaková2018). Microlite I with elevated Na content and Na-poor Mic II have low to zero concentrations of F (Fig. 6d; Table 3). However, Mic III (+ IIId) is F-enriched and Na-poor. Such a trend is remarkable because secondary microlites typically contain only minor to zero F concentrations and the A-site has a large vacancy content (e.g. Lumpkin et al., Reference Lumpkin, Chakoumakos and Ewing1986; Lumpkin and Ewing, Reference Lumpkin and Ewing1992; Loun et al., Reference Loun, Novák, Cempírek, Škoda, Vašinová–Galiová, Prokeš, Dosbaba and Čopjaková2018). Although an unusual trend it has been documented in post-magmatic microlite from beryl–columbite pegmatites of the Western Carpathians, which contain almost 3 wt.% F (≤0.9 apfu F) and ≤3.5 wt.% Na2O (≤0.6 apfu Na, Chudík and Uher, Reference Chudík and Uher2009; Chudík et al., Reference Chudík, Uher, Gadas, Škoda and Pršek2011) and similar microlite has also been described from the Schinderhübel I pegmatite (Černý et al., Reference Černý, Novák and Chapman1992). A reduction in analytical totals could be explained by an incorporation of hydroxyl groups (OH)– into the microlite structure or the presence of water molecules in domains of higher porosity (Geisler et al., Reference Geisler, Berndt, Meyer, Pollok and Putnis2004). This phenomenon is also known from altered zircon and monazite characterised by extensive porosity (Lee and Tromp, Reference Lee and Tromp1995; Nasdala et al., Reference Nasdala, Kronz, Wirth, Váczi, Pérez-Soba, Willner and Kennedy2009). Unaltered domains of Mic I and the majority of Mic III have analytical totals ≈99 wt.%, which decreases to ≈91 wt.% for more altered domains, mainly in Mic II (Figs 6–8; Tables 3, 5).
The substitution mechanisms in the individual types of microlite from the Schinderhübel I pegmatite differ significantly. The dominant substitution (1) ANaY(OH)ACa–1YO–1 in the heterogeneous Mic I is well constrained, whereas the minor substitution (2) AU0.5BTiYOA□–0.5B(Ta,Nb)–1Y(OH)–1, illustrating variations in Ti and (Nb, Ta)5+, is less pronounced. Substitution (1) is similar to the substitution ANaYFACa–1YO–1 interpreted in numerous pegmatites as primary hydrothermal alteration (Lumpkin et al., Reference Lumpkin, Chakoumakos and Ewing1986; Lumpkin and Ewing, Reference Lumpkin and Ewing1992; Novák and Černý, Reference Novák and Černý1998). However, microlite I is F-poor, hence this substitution has been modified to the F-free substitution (1) ANaY(OH)ACa–1YO–1. In the more heterogeneous Mic II, substitution (3) ACaYOA□–1Y□–1 is identical with secondary hydrothermal alteration of microlite (Lumpkin et al., Reference Lumpkin, Chakoumakos and Ewing1986, Novák and Černý, Reference Novák and Černý1998; Geisler et al., Reference Geisler, Berndt, Meyer, Pollok and Putnis2004; De Vito et al., Reference De Vito, Pezzotta, Ferrini and Aurisicchio2006; Loun et al., Reference Loun, Novák, Cempírek, Škoda, Vašinová–Galiová, Prokeš, Dosbaba and Čopjaková2018). Heterogeneous Ca-, F-rich Mic III has an unusual composition and the relevant substitution (4) ACa0.5YF0.5Y(OH)0.5A□–0.5Y□–1 is unique. It evidently demonstrates high activity of Ca and F in fluids which facilitated the crystallisation of Mic III, fersmite and of a distal Mic IIId on cracks of K-feldspar and quartz (Fig. 9). Substitution (4) clearly requires input of Ca and F from the external fluids, possibly from an amphibolite–biotite gneiss host (see below).
Raman spectroscopy of microlite and textural features of metamictisation
Factor group analysis yields six Raman-active modes for microlite using the site symmetries D 3d for A (16d) and B (16c) ions, C 2v for X (48f) ions and T d for Y (8b) ions, respectively (Oliveira et al., Reference Oliveira, Guedes, Ayala, Gesland, Ellena, Moreira and Grimsditch2004). Only one Raman-active vibration is associated with the Y-site (8b) ions (F 2g) and five for the X-site (48f) ions (A g + E g + 3F 2g) (Glerup et al., Reference Glerup, Nielsen and Poulsen2001). A tentative assignment of observed Raman modes was based on previous studies on pyrochlore-supergroup minerals (Geisler et al., Reference Geisler, Berndt, Meyer, Pollok and Putnis2004; Oliveira et al., Reference Oliveira, Guedes, Ayala, Gesland, Ellena, Moreira and Grimsditch2004; Arenas et al., Reference Arenas, Gasparov, Qiu, Nino, Patterson and Tanner2010; Andrade et al., Reference Andrade, Atencio, Chukanov and Ellena2013). However, the Raman shift of Mic I and II from Schinderhübel I is relatively different to the published data. Some of the bands are absent and most of the assigned bands were only roughly fitted. These Raman spectra display very broad, almost unrecognisable bands, suggesting very low structural order of Mic I and II (Fig. 10). Only Mic III provided spectra with relatively narrower and better-defined bands (mostly 532 and 637 cm–1, which are absent in Mic I, II), similar to published microlite Raman spectra (Geisler et al., Reference Geisler, Berndt, Meyer, Pollok and Putnis2004; Andrade et al., Reference Andrade, Atencio, Chukanov and Ellena2013). Only two spectra demonstrated the presence of low-intensity (OH)– bands: spectrum 2 of Mic I with 3502 cm–1 and spectrum 5 of Mic III with 3592 cm–1 (Fig. 10b; Table 6). No O–H band was determined in predominantly altered domains of Mic II veinlets; H2O bands at 1630 cm–1 are absent. These differences could be attributed to aqueous alteration (Geisler et al., Reference Geisler, Berndt, Meyer, Pollok and Putnis2004), though more probably result from the radiation damage (Zietlow, Reference Zietlow2016; Zietlow et al., Reference Zietlow, Beirau, Mihailova, Groat, Chudy, Shelyug, Navrotsky, Ewing, Schlütter, Škoda and Bismayer2017) due to the high U content (≤23 wt.%) in Mic I and Mic II, whereas it is below 4.5 wt.% in Mic III and IIId.
In addition to compositional variations, extensive textural transformations (existence of haloes surrounding metamict grains, high microporosity, etc.) are the most prominent markers of alteration and recrystallisation of the microlite crystals. Such textural variations are best seen in Mic I–III from Schinderhübel I (Fig. 3): homogeneous to slightly oscillatory zoned Mic I is characterised by a high porosity and extensive fracturing of its grain relics. Cracks in Mic I are filled by irregularly zoned Mic II veinlets (Fig. 3a,b). Finally, homogeneous-to-oscillatory zoned U-poor Mic III exhibits a low porosity and radiation damage. Radioactive decay of α particles of suitable radionuclides (especially U and Th) and recoil nuclei processes lead to amorphisation of the microlite structure (Ewing, Reference Ewing1994; Ewing et al., Reference Ewing, Meldrum, Wang, Wang, Ewing, Meldrum, Wang and Wang2000; Zietlow, Reference Zietlow2016; Zietlow et al., Reference Zietlow, Beirau, Mihailova, Groat, Chudy, Shelyug, Navrotsky, Ewing, Schlütter, Škoda and Bismayer2017), which is manifested by an increase in grain volume and radial cracks from the core to the grain periphery (Lee and Tromp, Reference Lee and Tromp1995; Loun et al., Reference Loun, Novák, Cempírek, Škoda, Vašinová–Galiová, Prokeš, Dosbaba and Čopjaková2018).
Fluid-mediated alteration of microlites
Several alteration and recrystallisation stages of microlite were recognised, mainly in the Schinderhübel I pegmatite: (1) formation of Mic I on CGM fractures; (2) recrystallisation of Mic I on Mic II; and (3) recrystallisation/replacement of both Mic I and II on Mic III. Microlite I has a slightly low A-site vacancy, moderate contents of Na (≤0.40 apfu) and very low F. The microlite I composition was probably more or less modified during the subsequent stage (2), characterised by a significant increase in the A-site vacancy (Fig. 6b). Loss of Na and Ca are typical whereas B-site cations as well as concentrations of U are stable (Figs 6c, 9). This indicates resistance of U during early replacement processes and does not fully correlate with the common U loss described in altered metamict microlite (e.g. Ewing et al., Reference Ewing, Meldrum, Wang, Wang, Ewing, Meldrum, Wang and Wang2000; Zietlow et al., Reference Zietlow, Beirau, Mihailova, Groat, Chudy, Shelyug, Navrotsky, Ewing, Schlütter, Škoda and Bismayer2017). Substantial A-site cation loss is marked by a decrease in analytical totals in Mic II to ~90 wt.%. Both (1) and (2) stages might represent individual episodes of generally assumed secondary alteration in granitic pegmatites in the sense of Lumpkin and Ewing (Reference Lumpkin and Ewing1992). In contrast to stages (1) and (2), stage (3) includes more complex compositional variations. Mineral-replacement processes lead to Na and predominantly U and Ti loss, as well as evident Ca- and F-enrichments (Figs 6a,b,d, 9).
The origin of early Mic I was probably facilitated by residual fluids exsolved from pegmatite melt in the latest stage of magmatic crystallisation. However, some participation of externally derived fluids is indicated by the elevated Mg contents of the associated recrystallised CGM (Chládek et al., Reference Chládek, Uher and Novák2020). The chemical signature of this later process is transitional between primary magmatic microlite and the microlite originated during early alteration (Lumpkin and Ewing, Reference Lumpkin and Ewing1992). The fluids that generated Mic II could have a similar origin as those forming Mic I, although the alteration proceeded at slightly lower temperatures along thin cracks in the CGM (Fig. 3a,b). This compositional trend resulted in an increased A-site vacancy in Mic II; and is similar to the secondary alteration defined by Lumpkin and Ewing (Reference Lumpkin and Ewing1992), and described from numerous pegmatites (e.g. Chudík and Uher, Reference Chudík and Uher2009; Chudík et al., Reference Chudík, Uher, Gadas, Škoda and Pršek2011; Melcher et al., Reference Melcher, Graupner, Gäbler, Sitnikova, Henjes-Kunst, Oberthür, Gerdes and Dewaele2015; Loun et al., Reference Loun, Novák, Cempírek, Škoda, Vašinová–Galiová, Prokeš, Dosbaba and Čopjaková2018). However, the microlite compositions with very high A-site vacancy and very low contents of F, typical for low-temperature hydrothermal and weathering processes facilitated by meteoritic fluids (e.g. Lumpkin and Ewing, Reference Lumpkin and Ewing1992; Loun et al., Reference Loun, Novák, Cempírek, Škoda, Vašinová–Galiová, Prokeš, Dosbaba and Čopjaková2018), are absent in the pegmatites investigated.
The latest stage of microlite, Mic III (+ IIId), characterised by high Ca, low A-site vacancy and elevated F contents does not fit with final low-temperature secondary alteration of pyrochlore-supergroup minerals described elsewhere (e.g. Lumpkin and Ewing, Reference Lumpkin and Ewing1992; Llorens and Moro, Reference Llorens and Moro2010; Loun et al., Reference Loun, Novák, Cempírek, Škoda, Vašinová–Galiová, Prokeš, Dosbaba and Čopjaková2018). A significant addition of Ca is apparent in the fluids from the Schinderhübel I pegmatite, demonstrated by the common association of epidote ± pumpellyite (Dostál, Reference Dostál1966; unpubl. data of MN) with late pyrochlore (Novák and Dosbaba, Reference Novák and Dosbaba2006), and by secondary bavenite–bohseite after beryl, known from most Maršíkov pegmatites (Dolníček et al., Reference Dolníček, Nepejchal, Sejkora, Ulmanová and Chládek2020a,Reference Dolníček, Nepejchal and Novákb). Whether there is an external or internal source of Ca in subsolidus processes in granitic pegmatites is a matter of discussion, and an external source has been suggested (e.g. Martin and De Vito, Reference Martin and De Vito2014; Novák et al., Reference Novák, Prokop, Losos and Macek2017). Further, the temperature of these external fluids might be slightly higher relative to stage (2). Fissure-filling Mic III (+ IIId) texturally resembles Alpine-type hydrothermal veins (e.g. Weisenberger and Bucher, Reference Weisenberger and Bucher2011) and these authors also consider host rocks the sourcee, not only of Ca, but also other elements. Consequently, late hydrothermal-metamorphic fluids could facilitate the origin of Mic III, Mic IIId and associated fersmite (Fig. 3). Fluorine might have been released from the rock-forming minerals of the host amphibole gneiss or parental pegmatite during such a hydrothermal-metamorphic overprint. Biotite and amphibole from host rocks are potential sources of fluorine; elevated concentrations of fluorine were found in pumpellyite (≤0.45 wt.% F) and epidote (≤0.2 wt.% F) associated with bavenite–bohseite at thin Alpine-type veinlets cutting the Schinderhübel I pegmatite (unpubl. data of MN). However, we cannot exclude pegmatitic muscovite (≤0.2 wt.% F; Dolníček et al., Reference Dolníček, Nepejchal and Novák2020b) as another source of F.
Conclusions
Microlite- (and rarely pyrochlore-) group minerals are essential tracers of post-magmatic evolution of the beryl–columbite pegmatites in the Maršíkov District. Complex subsolidus evolution of Nb–Ta mineralisation involves individual stages (1), (2) and (3) producing microlite types [Mic I, Mic II, Mic III and Mic IIId], respectively (Fig. 11). The complete sequence of microlite types has only been found in the Schinderhübel I pegmatite. The alteration processes commenced with recrystallisation of primary magmatic to secondary post-magmatic CGM, facilitated by residual fluids locally mixed with external fluids from the host rocks (Chládek et al., Reference Chládek, Uher and Novák2020) and crystallisation of early Na-rich Mic I. Subsequent formation of Na-poor Mic II indicates a change of the fluid composition connected with partial alteration of Mic I and removal of Na (Fig. 11). Microlite II is compositionally very similar to secondary alteration microlite (sensu Lumpkin and Ewing, Reference Lumpkin and Ewing1992). The latest stage (3), producing Ca- and F-enriched Mic III + fersmite and rare distal Mic IIId displays a different textural style; hence, the extensive introduction of external (metamorphic) fluids enriched in Ca, and perhaps F is feasible. The wide diversity of compositional and textural features of microlite minerals and secondary CGM reflects the complex evolution of the pegmatites during the subsolidus, late hydrothermal stage, probably including a weak-to-moderate metamorphic overprint at the latest event.

Fig. 11. Evolutionary sequence of microlite-group minerals.
Acknowledgements
We thank Alessandro Guastoni, Magdalena Dumańska-Słowik, an anonymous reviewer and editors of the Mineralogical Magazine for their critical and constructive reviews, as well as Viera Kollárová for their assistance during electron microprobe work. This research was supported by the Slovak Research and Development Agency (APVV-15-0050 and APVV-18-0065 projects), the Ministry of Education, Slovak Republic (VEGA-1/0499/16, VEGA-1/007915 and VEGA-1/0137/20) to PU and PB, the research project GAČR 19-05198S to MN, the Ministry of Education, Youth and Sports of the Czech Republic, Grant number SGS SP 2020/105 to ŠCH and by the Palacký University in Olomouc (IGA_PrF_2020_030) to TO.