Introduction
Numerous weed species have evolved resistance to herbicides in many countries, which has made their management much more difficult and threatens crop production globally. Evolution of herbicide resistance is widely documented in southern Australian cropping systems in the genetically diverse, outcrossing species rigid ryegrass (Lolium rigidum Gaudin). Resistance in this species is conferred by mutations at the herbicide target site (Christopher et al. Reference Christopher, Powles and Holtum1992; Malone et al. Reference Malone, Boutsalis, Baker and Preston2014) or non–target site mechanisms, such as enhanced herbicide metabolism, which can confer cross- and multiple resistance (Preston et al. Reference Preston, Tardif, Powles and Brown1996; Yu and Powles Reference Yu and Powles2014). The role of different enzymatic processes involving cytochrome P450 enzymes have been identified as capable of herbicide metabolism in crop species, endowing crop selectivity to certain herbicides (Owen Reference Owen and Kirkwood1991; Van Eerd et al. Reference Van Eerd, Hoagland, Zablotowicz and Hall2003). Furthermore, the conjugation of some herbicides to the tripeptide glutathione occurs with glutathione S-transferases (GSTs), resulting in metabolic detoxification in crop species (Pan et al. Reference Pan, Si, Yu, Tu and Powles2012; Tanetani et al. Reference Tanetani, Ikeda, Kaku, Shimizu and Matsumoto2013). Resistance mediated by P450 or GST enzymes via enhanced herbicide metabolism has led to resistance to multiple site-of-action herbicides in L. rigidum in Australia (Busi et al. Reference Busi, Porri, Gaines and Powles2018; Preston Reference Preston2004), blackgrass (Alopecurus myosuroides Huds.) in England (Keshtkar et al. Reference Keshtkar, Mathiassen, Moss and Kudsk2015), and wild oat (Avena fatua L.) in Canada (Mangin et al. Reference Mangin, Hall and Beckie2016).
Organophosphate insecticides are highly reactive and chemically diverse molecules that possess a phosphorous atom with a covalent bond to either sulfur or oxygen (Durst et al. Reference Durst, Salaun, WerckReichhart, Zimmerlin, DePrado, Jorrin and GarciaTorres1997). Certain organophosphate insecticides have inhibitory effects on some P450 enzymes through which some herbicides are detoxified (Durst et al. Reference Durst, Salaun, WerckReichhart, Zimmerlin, DePrado, Jorrin and GarciaTorres1997; Kreuz et al. Reference Kreuz, Tommasini and Martinoia1996). Organophosphate insecticides have been shown to significantly reduce phytotoxicity from clomazone (13(F4): inhibitors of carotenoid biosynthesis) when applied to cotton (Gossypium hirsutum L.) by inhibiting P450-mediated oxidation into toxic clomazone metabolites (Ferhatoglu et al. Reference Ferhatoglu, Avdiushko and Barrett2005). Similarly, the thiocarbamate herbicides require conversion into a toxic metabolite in order to exert their herbicidal effects. Activation involves the oxidation of the parent compound via P450 enzymes into the bioactive sulfoxide form (Casida et al. Reference Casida, Gray and Tilles1974; Fuerst Reference Fuerst1987). The ability of organophosphate insecticides to act as a suicide substrate through binding and catalysis results in a phosphorous atom of the insecticide substrate molecule being released and covalently bound to the P450, thereby inhibiting activation or metabolism of these herbicides by P450 enzymes that can result in either increased resistance (thiocarbamates) or susceptibility (dinitroaniline and benzamides) in L. rigidum (Busi et al. Reference Busi, Gaines and Powles2017; Tanetani et al. Reference Tanetani, Ikeda, Kaku, Shimizu and Matsumoto2013; Tardif and Powles Reference Tardif and Powles1999).
The L. rigidum population SLR31 has been comprehensively studied because of its known metabolism-based multiple resistance to the herbicides diclofop-methyl (1(A): inhibitors of acetyl CoA carboxylase [ACCase]) and chlorsulfuron (2(B): inhibitors of acetolactate synthase [ALS]) (Heap and Knight Reference Heap and Knight1986). Furthermore, it exhibits a moderate level of resistance to trifluralin (3(K1): inhibitors of microtubule polymerization) (McAlister et al. Reference McAlister, Holtum and Powles1995), S-metolachlor (15(K3): inhibitors of very-long-chain fatty-acid [VLCFA] synthesis) (Burnet et al. Reference Burnet, Barr and Powles1994a), and triallate (8(N): inhibitors of fatty acid elongation) (Tardif and Powles Reference Tardif and Powles1999). Further studies with SLR31 have confirmed altered target-site (ACCase) and non–target site enhanced herbicide metabolism (ALS) resistance (Christopher et al. Reference Christopher, Powles, Liljegren and Holtum1991, Reference Christopher, Powles and Holtum1992, Reference Christopher, Preston and Powles1994). Evolutionary studies in the glasshouse using low herbicide dose recurrent selection of SLR31 have selected resistance to the sulfonylisoxazoline and thiocarbamate herbicides, pyroxasulfone and prosulfocarb (Busi and Powles Reference Busi and Powles2013). Other than the studies by Busi et al. (Reference Busi, Gaines and Powles2017) on the recurrently selected population, there is limited information on non–target site resistance to thiocarbamates and cross-resistance to other chemical classes in L rigidum. Furthermore, non–target site resistance to these herbicides has not been investigated in any field-selected resistant populations of L. rigidum.
Previous studies have highlighted direct involvement of P450 enzymes as a mechanism of metabolic resistance to specific herbicides, including pyroxasulfone in the recurrently selected population (Busi and Powles Reference Busi and Powles2016). In this study, we investigated the response of seven field-selected populations from across southern Australia to six different herbicides from three different sites of action. The objective was to understand whether the P450 inhibitor phorate could increase susceptibility to these herbicides and whether the response of these field-selected L. rigidum populations would differ from those reported for SLR31 in previous studies (Busi et al. Reference Busi, Gaines and Powles2017).
Materials and Methods
Plant Material
The seed of L. rigidum populations 375-14 (R), 198-15 (R), 16.2 (R), RAC1 (R), A18 (R), and EP162 (R) used in this study was collected from cropping fields across southern Australia as described by Brunton et al. (Reference Brunton, Boutsalis, Gill and Preston2019). A well-characterized L. rigidum population, SLR4, was used as the susceptible (S) control (Boutsalis et al. Reference Boutsalis, Gill and Preston2012; Brunton et al. Reference Brunton, Boutsalis, Gill and Preston2018). In addition, the extensively studied multiple resistant L. rigidum population, SLR31, was used as a known metabolic-resistant biotype (Burnet et al. Reference Burnet, Barr and Powles1994a, Reference Burnet, Hart, Holtum and Powles1994b; McAlister et al. Reference McAlister, Holtum and Powles1995). Seeds from SLR4, 375-14, 198-15, 16.2, EP162, A18, RAC1, and SLR31 were weighed (0.2 g = 50 to 60 seeds) and spread onto the surface of 9.5 cm by 8.5 cm by 9.5 cm punnet pots (Masrac Plastics, Dry Creek, South Australia) containing cocoa peat potting mix as described by Boutsalis et al. (Reference Boutsalis, Gill and Preston2012).
Inhibitor and Herbicide Application
Before herbicide application (3 h), the insecticide phorate (Thimet®, Barmac Industries, Stapylton, QLD, Australia) was applied to the soil surface as described by Busi et al. (Reference Busi, Gaines and Powles2017) at a dose of 0.076 g pot−1 corresponding to 10 kg ha−1 phorate (Table 1). Pots treated with the inhibitor only were also included. Herbicides were applied directly to seed and soil using a laboratory spray cabinet equipped with flat-fan nozzles (Hardi® ISO F-110-01, Hardi, Adelaide, SA, Australia) delivering 118 L ha−1 water at a pressure of 2.54 kPa. The control pots were not treated with any herbicide or inhibitor. The experiment was conducted twice outdoors under natural growing conditions in winter during the normal growing season (May to July 2018). Pots were watered as needed to maintain the potting mix near field capacity. There were three replicates for each herbicide dose, and pots were arranged in a randomized complete block design.
Table 1. Active ingredients, formulations, and manufacturers of herbicides and insecticide used in dose–response experiments.

Dose–Response Experiment and Seedling Growth
The six PRE herbicides were applied (Table 1) following the methods described by Boutsalis et al. (Reference Boutsalis, Gill and Preston2012). The herbicide triallate was applied to the S biotype at 50, 100, 200, 400, 800, and 1,600 g ha−1 and to the R biotypes at 400, 800, 1,600, 3,200, 6,400, and 12,800 g ha−1. In Australia, the recommended label rate of triallate for L. rigidum control is 1,500 g ha−1. Prosulfocarb was applied to the S biotype at 75, 150, 300, 600, 1,200, and 2,400 g ha−1 and to the R biotypes at 600, 1,200, 2,400, 4,800, 9,600, and 19,200 g ha−1. The recommended label rate of prosulfocarb for L. rigidum control is 2,400 g ha−1.
Pyroxasulfone was applied to the S biotype at 3.2, 6.4, 12.8, 25.5, 51, and 102 g ha−1 and to the R biotypes at 25.5, 51, 102, 204, and 408 g ha−1 with the recommended label rate for L. rigidum control being 100 g ha−1. S-metolachlor was applied to the S biotype at 15, 30, 60, 120, 240, and 480 g ha−1 and to the R biotypes at 120, 240, 480, 960, 1,920, and 3,840 g ha−1 with the recommended label rate for L. rigidum control in Australia being 480 g ha−1.
Trifluralin was applied to the S biotype at 7.81, 15.62, 31.25, 62.5, 125, 250, and 500 g ha−1 and to the R biotypes at 125, 250, 500, 1,000, 2,000, and 4,000 g ha−1 with the recommended label rate for L. rigidum control being 500 g ha−1. Propyzamide was applied to the S biotype at 7.81, 15.62, 31.25, 62.5, 125, 250, and 500 g ha−1 and to the R biotypes at 125, 250, 500, 1,000, and 2,000 g ha−1 with the recommended label rate for L. rigidum control in Australia being 500 g ha−1. Pots were assessed at 28 d after herbicide treatment, and plants that had emerged and grown to the 2-leaf stage were counted. Percentage survival was assessed as the number of plants growing in the treated pots compared with the average number present in the untreated pots.
Statistical Analysis
Following an ANOVA, the data for both experimental runs were pooled, as there was no statistical difference between the runs. The data were analyzed using a log-logistic equation (GraphPad Prism v. 7.0, GraphPad Software, San Diego, CA, USA) fit to the percentage emergence data (Seefeldt et al. Reference Seefeldt, Jensen and Fuerst1995). The normalized three-parameter logistic regression model was fit where y represents plant survival (%), x is the log-dose of the herbicide used, LD50 is the herbicide dose required to cause 50% reduction in plant emergence, and b denotes the slope of the curve. LD50 parameter estimates from the log-logistic analysis were used to calculate the resistance index (RI), which is the resistant/susceptible ratio of the LD50.

To estimate no difference between estimated LD50 values for L. rigidum populations in the presence of the insecticide phorate, a t-test was conducted with the null hypothesis of no difference between resistant versus susceptible populations or their ratio was equal to 1 (Ritz et al. Reference Ritz, Kniss and Streibig2015).
Results and Discussion
Effect of Phorate on Thiocarbamates Herbicides
In the absence of herbicide, phorate at 10 kg ha−1 had no effect on the germination percentage of all L. rigidum populations when compared with nontreated controls (data not shown). The susceptible population SLR4 was completely controlled by both triallate and prosulfocarb applied at the recommended field rate. The LD50 value for SLR4 treated with triallate was 179 g ha−1 and ranged from 745 to 8,089 g ha−1 for the resistant populations (4.2- to 45.2-fold resistance compared with SLR4) (Table 2). The addition of phorate significantly (P < 0.01) increased the LD50 for triallate in SLR4, 375-14, 16.2, RAC1, A18, and SLR31 as compared with the herbicide-only treatment. In the presence of phorate, the LD50 for SLR4 was 1,595 g ha−1 and ranged from 2,738 to 8,579 g ha−1 for the resistant populations (15.3- to 47.9-fold resistance compared with SLR4) in the absence of phorate (Table 2). The L. rigidum populations SLR4, 16.2, RAC1, A18, and SLR31 treated with phorate and prosulfocarb displayed a significant (P < 0.01) antagonistic response similar to triallate. In the presence of phorate, the LD50 value for SLR4 was 2,006 g ha−1 (5.7-fold increase) and ranged from 3,379 to 18,329 g ha−1 (9.6- to 51.9-fold for the resistant biotypes compared with SLR4) in the absence of phorate (Table 2).
Table 2. Pooled dose–response data of thiocarbamate herbicides triallate and prosulfocarb with or without phorate required for 50% mortality (LD50) of resistant and susceptible Lolium rigidum populations with 95% confidence intervals in parentheses and resistance index (RI).
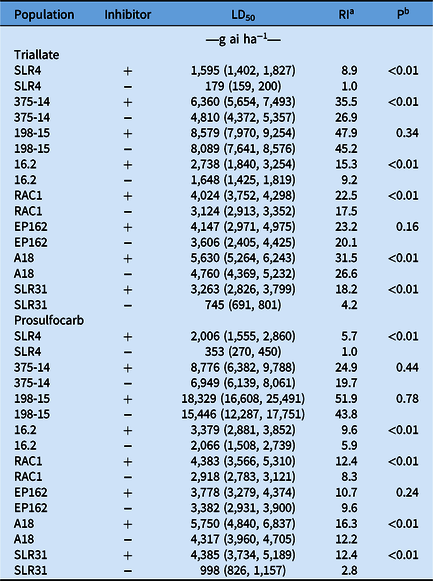
a RI values calculated as the ratio between the LD50 of the resistant populations compared with the mean LD50 value of the susceptible population (SLR4) without phorate.
b Probability value (P) indicates the significance of differences in LD50 values between L. rigidum populations treated or not treated with phorate.
In this study, we report major differences between field-selected L. rigidum populations in response to herbicides when the P450 inhibitor phorate was present. Phorate was shown to antagonize the thiocarbamate herbicides triallate in SLR4, 375-14, 16.2, RAC1, A18, and SLR31 and prosulfocarb in SLR4, 16.2, RAC1, A18, and SLR31. As previously reported, P450 enzymes are involved in the activation of thiocarbamate herbicides (Fuerst Reference Fuerst1987), and therefore, inhibition of P450s by phorate would reduce the biological activity of these herbicides. Reduced activation has been documented to elicit triallate resistance in A. fatua (Kern et al. Reference Kern, Peterson, Miller, Colliver and Dyer1996). This was also shown in the recurrently selected L. rigidum by Busi et al. (Reference Busi, Gaines and Powles2017), who reported a herbicide-susceptible population treated with phorate exhibited an LD50 value similar to that seen in the resistant population by inhibiting the activation of either triallate or prosulfocarb.
The antagonism between triallate or prosulfocarb and phorate was also observed in the susceptible population SLR4, which indicates involvement of P450s in herbicide activation in this population as well. However, three of the resistant populations displayed no antagonism to phorate. If the P450 responsible for thiocarbamate activation is not active in these populations, then no antagonism with phorate would occur, suggesting a lack of activation as a possible resistance mechanism in these populations (Kern et al. Reference Kern, Peterson, Miller, Colliver and Dyer1996). Different P450s inhibited by phorate might bioactivate thiocarbamates in these populations or phorate might not inhibit these P450s, leading to further detoxification of the sulfoxide activated by GSTs. The resistant populations 198-15 and EP162 treated with either triallate or prosulfocarb displayed an antagonistic response with phorate, suggesting that specific P450s involved in thiocarbamate conversion are still active and resistance is likely due to something other than the loss of a specific P450. The possible involvement of a target-site resistance mechanism is known to confer resistance to trifluralin, but not to other herbicides, including the thiocarbamates. This suggests a complex set of resistance mechanisms involving lack of herbicide activation, but also possible involvement at a later stage of glutathione conjugation of the herbicide following activation (Fuerst Reference Fuerst1987). The involvement of GSTs mediating herbicide detoxification via glutathione conjugation is widely documented for chloroacetamide and thiocarbamate herbicides (Cummins et al. Reference Cummins, Moss, Cole and Edwards1997; Siminszky Reference Siminszky2006). Studies by Busi et al. (Reference Busi, Gaines and Powles2017) suggested the presence of a metabolism-based mechanism in L. rigidum similar to that found in wheat (Triticum aestivum L.).
Effect of Phorate on VLCFA Inhibitors
All L. rigidum populations treated with S-metolachlor displayed no statistical difference (P > 0.05) in LD50 in the presence of phorate compared with herbicide-only treatment (Table 3). The S-metolachlor rate required to control 50% of the SLR4 population was 148 g ha−1. In contrast, the LD50 for the resistant populations ranged from 250 to 4,179 g ha−1 (1.7- to 28.2-fold). In the presence of phorate, the LD50 was 125 g ha−1 for SLR4 and ranged from 240 to 3,620 g ha−1 (1.6- to 24.5-fold) in the resistant populations. As with S-metolachlor, phorate treatment had no significant effect on the LD50 response of the susceptible and resistant populations treated with pyroxasulfone compared with the absence of phorate (Table 3).
Table 3. Pooled dose–response data of the very-long-chain fatty-acid–inhibiting herbicides S-metolachlor and pyroxasulfone with or without phorate required for 50% mortality (LD50) of resistant and susceptible Lolium rigidum populations with 95% confidence intervals in parentheses and resistance index (RI).

a RI values calculated as the ratio between the LD50 of the resistant populations compared with the mean LD50 value of the susceptible population (SLR4) without phorate.
b Probability value (P) indicates the significance of differences in LD50 values between L. rigidum populations treated or not treated with phorate.
Our results suggest limited involvement of P450s in the metabolism of the VLCFA-inhibiting herbicides S-metolachlor and pyroxasulfone. More recently, studies have shown that rapid GST-mediated pyroxasulfone metabolism in wheat compared with slower metabolism in L. rigidum plants was responsible for herbicide selectivity (Tanetani et al. Reference Tanetani, Ikeda, Kaku, Shimizu and Matsumoto2013). In addition, Busi et al. (Reference Busi, Porri, Gaines and Powles2018) suggested that increased transcription of GST was the primary mechanism of resistance to the VLCFA-inhibiting herbicides and that the expression of five P450s did not substantially differ among individuals in the recurrently selected L. rigidum populations. They also showed a synergistic interaction between phorate and pyroxasulfone that substantially reduced (approximately 45%) the level of pyroxasulfone resistance. However, in this study, we found no significant synergistic effect between phorate and either VLCFA-inhibiting herbicide. Limited P450 response was observed with no change in LD50 either in the presence or absence of phorate in all populations. A possible explanation is that phorate does not inhibit specific P450s involved in the metabolism of S-metolachlor and pyroxasulfone. However, evidence of enhanced P450 activity conferring resistance to these herbicides in L. rigidum remains not fully understood, and it has been reported that selectivity of these herbicides in crops can be attributed to enhanced levels of GST activity (Lamoureux et al. Reference Lamoureux, Shimabukuro, Frear, Caseiey, Cussans and Atkin1991). The response of both resistant and susceptible L. rigidum populations to chloroacetamide and sulfonylisoxazoline herbicides suggests a possible pathway wherein herbicides are metabolized by GST conjugation similar to that reported in wheat and L. rigidum (Busi et al. Reference Busi, Porri, Gaines and Powles2018; Dücker et al. Reference Dücker, Zöllner, Lümmen, Ries, Collavo and Beffa2019; Tanetani et al. Reference Tanetani, Ikeda, Kaku, Shimizu and Matsumoto2013).
Effect of Phorate on Microtubule Polymerization Inhibitors
The LD50 for L. rigidum population SLR4 treated with trifluralin in the absence of phorate was 52 g ha−1 compared with 73 g ha−1 when treated with phorate, indicating a significant (P < 0.01) antagonistic response (Table 4). However, there was a significant (P < 0.01) reduction in the rate of trifluralin required to control some L. rigidum populations when phorate was applied. The addition of phorate reduced the LD50 for trifluralin by about 50% in the resistant populations 16.2, RAC1, A18, and SLR31. The LD50 in the absence of phorate ranged from 146 to 1,471 g ha−1 (2.8- to 28.3-fold compared with SLR4), which reduced to 117 to 665 g ha−1 (2.3- to 12.8-fold resistance compared with SLR4) in the presence of phorate. However, three of the trifluralin-resistant populations (375-14, 198-15, and EP162) showed no change in LD50 to trifluralin with the addition of phorate. All L. rigidum populations were controlled by propyzamide at the recommended dose. Addition of phorate to propyzamide reduced LD50 (0.6- to 1.5-fold) in all L. rigidum populations; however, this was only significant (P < 0.01) for SLR4 (S), 16.2, RAC1, and SLR31 (Table 4).
Table 4. Pooled dose–response data of the microtubule polymerization–inhibiting herbicides trifluralin and propyzamide with or without phorate required for 50% mortality (LD50) of resistant and susceptible Lolium rigidum populations with 95% confidence intervals in parentheses and resistance index (RI).

a RI values calculated as the ratio between the LD50 of the resistant populations compared with the mean LD50 value of the susceptible population (SLR4) without phorate.
b Probability value (P) indicates the significance of differences in LD50 values between L. rigidum populations treated or not treated with phorate.
There was significant synergism between trifluralin and phorate in some L. rigidum populations. Therefore, a possible P450-mediated herbicide detoxification mechanism appears to be involved in microtubule polymerization inhibitor resistance in L. rigidum. In the susceptible population, phorate antagonized trifluralin. One possible explanation is that P450 inhibitors might induce P450 activity, resulting in herbicide metabolism (Hidayat and Preston Reference Hidayat and Preston2001). Previous studies in L. rigidum and A. myosuroides have shown that the presence of resistance to the microtubule polymerization–inhibiting herbicide pendimethalin could be reversed with the P450 inhibitor malathion (James et al. Reference James, Kemp and Moss1995; Tardif and Powles Reference Tardif and Powles1999). Some of the populations of L. rigidum showed no synergism between phorate and trifluralin, which suggests the presence of a possible target-site resistance mechanism. The amino acid change from threonine to isoleucine at position 239 or valine to phenylalanine at position 2020 of the α-tubulin gene contributes greater resistance to trifluralin (>10-fold) compared with metabolic resistance (Fleet et al. Reference Fleet, Malone, Preston and Gill2018). All L. rigidum populations treated with propyzamide were susceptible and completely killed at the recommended dose. However, there was significant synergism between propyzamide and phorate for four out of the eight populations. Therefore, a metabolic P450 detoxification mechanism for propyzamide is likely in L. rigidum.
This study highlights an antagonistic response to the P450 inhibitor phorate in L. rigidum when treated with thiocarbamate herbicides, no response when treated with VLCFA-inhibiting herbicides, and a synergistic response with microtubule polymerization–inhibiting herbicides. This suggests the possible involvement of a complex of P450-mediated and other mechanisms in resistant L. rigidum populations, similar to those conferring tolerance to thiocarbamate, chloroacetamide, and sulfonylisoxazoline herbicides in wheat (Busi et al. Reference Busi, Gaines and Powles2017; Tanetani et al. Reference Tanetani, Ikeda, Kaku, Shimizu and Matsumoto2013). In addition, some populations showed no interaction between phorate and trifluralin, suggesting a possible target-site resistance mechanism. There were also differences among the resistant populations, with populations 198-15 and EP162 not responding to phorate in combination with any of the six herbicides used. The possibility that phorate may be detoxified before it is able to inhibit P450s in these populations is likely and would explain the limited response in these populations. The application of a GST inhibitor may clarify the possible involvement of GSTs in the studied field-selected L. rigidum populations. However, there remains a limited understanding about the possibility of a specific target-site mutation that confers resistance (>20-fold) to inhibitors of fatty-acid elongation and VLCFA inhibition in these populations.
Phorate enables a greater understanding of potential biochemical mechanisms involved in herbicide resistance, but it is unlikely that applications of this P450 inhibitor in broad-acre scale would be environmentally or economically sustainable. Furthermore, phorate was unable to completely overcome resistance and antagonized thiocarbamate-resistant and thiocarbamate-susceptible populations. The challenges of understanding metabolic resistance in L. rigidum reported in this study highlight the significant differences in response among populations, herbicides, and inhibitors. In the presence of phorate, populations 198-15 and EP162 showed no response when treated with either thiocarbamate or microtubule polymerization–inhibiting herbicides, suggesting the likelihood of a complex resistance mechanism. In addition to these populations showing no response to either herbicide, the response of the other populations varied significantly, highlighting a diversity among the resistant biotypes. Lolium rigidum populations 16.2, RAC1, and SLR31 responded to all site-of-action herbicides. In contrast, population 375-14 responded to triallate only, while A18 showed a response to both thiocarbamates and the microtubule polymerization inhibitor (trifluralin). Metabolic detoxification mechanisms in L. rigidum have the ability to confer resistance to herbicides that have never been applied and result in herbicides with different sites of action not controlling these populations in the field (Brunton et al. Reference Brunton, Boutsalis, Gill and Preston2019; Mangin et al. Reference Mangin, Hall and Beckie2016; Tardif and Powles Reference Tardif and Powles1999). Therefore, in addition to the use of herbicides, a diverse array of cultural and non-herbicidal weed control tactics should be implemented to manage L. rigidum populations to reduce the amount of viable seed returning to the weed seedbank (Beckie Reference Beckie2006).
Acknowledgments
The authors acknowledge the Grains Research and Development Corporation for funding this research. Additional acknowledgment to Des Andriske from Barmac Industries for supplying Thimet® (phorate). No conflicts of interest have been declared.