1. Introduction
The Central Asian Orogenic Belt (CAOB) is one of the largest and longest-lived accretionary orogenic collages in the world, with considerable Neoproterozoic and Phanerozoic crustal growth (Jahn et al. Reference Jahn, Wu and Chen2000; Xiao et al. Reference Xiao, Zhang, Qin, Sun and Li2004, Reference Xiao, Windley, Allen and Han2013, Reference Xiao, Chen, Hollings, Han, Wang, Yang and Cai2017; Yakubchuk, Reference Yakubchuk2017). It experienced a long and complex geological history, for example, the successive amalgamation and multiple collisions of accretionary wedges, island arcs, microcontinents and oceanic seamounts (Xiao et al. Reference Xiao, Windley, Allen and Han2013; Xie et al. Reference Xie, Luo, Xu, Chen, Hong, Ma and Ma2016a, b, c; Wali et al. Reference Wali, Wang, Cluzel and Zhong2018).
In the Bogda Mountains, located in the northern segment of the eastern Tianshan, SW CAOB, Carboniferous–Permian volcanic rocks and coeval intrusive rocks are well developed, including Daheyan intrusions. There is also an important ore province in northeastern Tianshan, and many late Carboniferous Cu polymetallic deposits, for example, the deposits of Tonggou, Miao’ergou, Xingshugou and Weicaogou, have been discovered and exploited. However, the late Palaeozoic tectonic setting of the Bogda Mountains is still the subject of strong debate concerning island-arc-, rifting- and mantle-plume-related large igneous provinces, post-collisional extension and intra-arc extension setting (Gu et al. Reference Gu, Hu, Yu, Li, Xiao and Yan2000, Reference Gu, Hu, Yu, Zhao, Wu and Li2001; Shu et al. Reference Shu, Zhu, Wang, Faure, Charvet and Cluzel2005, Reference Shu, Wang, Zhu, Guo, Charvet and Zhang2011; Xie et al. Reference Xie, Luo, Xu, Chen, Hong, Ma and Ma2016a, b, c; Wali et al. Reference Wali, Wang, Cluzel and Zhong2018). Numerous late Carboniferous intermediate-felsic intrusions have been found in the Daheyan area of the Bogda Mountains during regional geological surveying in recent years. These intrusions contain plenty of geological information about the tectonic evolutionary history of eastern Tianshan and can greatly contribute to our understanding of the suture zone. It is therefore a key area for understanding the late Palaeozoic geodynamic setting of mineralization and tectonic evolution of eastern Tianshan.
The Daheyan area is located in the southwestern Bogda Mountains, eastern Tianshan and Daheyan intrusions crop out to the north of the town of Daheyan. This study presents detailed zircon U–Pb, whole-rock geochemical and Hf isotope analyses for the intrusions. We discuss the tectonic setting and geodynamic evolution of eastern Tianshan during c. 311–303 Ma. We also address the tectonic setting of Cu polymetallic mineralization in the Bogda Mountains.
2. Regional tectonic setting
The E–W-striking Chinese Tianshan Belt is a major tectonic unit in the southernmost part of the CAOB (Fig. 1a; Allen et al. Reference Allen, Windley and Zhang1993). The eastern Tianshan is the east part of the Chinese Tianshan Belt, and is bounded by the Junggar Block to the north and the Precambrian Tarim Block to the south (Fig. 1b; Zhang et al. Reference Zhang, Niu, Terada, Yu, Sato and Ito2003; Han et al. Reference Han, Guo, Zhang, Zhang, Chen and Song2010). This belt witnessed the opening and closure of the Palaeo-Asian ocean during Palaeozoic–Triassic time (Allen et al. Reference Allen, Sengor and Natal’in1995; Carroll et al. Reference Carroll, Graham, Hendrix, Ying and Zhou1995; Wu et al. Reference Wu, Zhao, Sun, Wilde and Yang2007; Xu et al. Reference Xu, Charvet, Chen, Zhao and Shi2012), and experienced multiple accretion, collision and mineralization events (Xiao et al. Reference Xiao, Zhang, Qin, Sun and Li2004, Reference Xiao, Windley, Allen and Han2013). This area is subdivided into four tectonic units (from north to south): (1) the Bogda-Harlik Orogenic Belt, which is bounded by the Junggar basin in the north and the Turpan-Hami basin in the south; (2) the Dananhu-Tousuquan Zone, which is situated north of the Kangguer Fault; (3) the Kanggur-Yamansu-Heiyingshan Zone, which is between the Kangguer and Aqikuduke faults; and (4) the Central Tianshan Massif, which lies between the Aqikuduke-Shaquanzi Fault in the north and the Hongliuhe-Xingxingxia and Kawabulak faults in the south (Fig. 1b; Qin et al. Reference Qin, Su, Sakyi, Tang, Li, Sun, Xiao and Liu2011; Su et al. Reference Su, Qin, Sun, Tang, Sakyi, Chu and Xiao2012; Wu et al. Reference Wu, Chen and Zhou2016).

Fig. 1. (a) Schematic geological map of the Central Asian Orogenic Belt (CAOB); (b) simplified tectonic sketch map of most of Xinjiang Province, NW China (modified after Pirajno et al. Reference Pirajno, Mao, Zhang, Zhang and Chai2008; Wang et al. Reference Wang, Shu, Faure, Jahn, Cluzel, Charvet, Chung and Meffre2011; Xiao et al. Reference Xiao, Windley, Allen and Han2013); and (c) geological map of the Bogda Orogenic Belt at the north margin of the Chinese North Tianshan (modified after Chen et al. Reference Chen, Zhao and Deng2010; Zhao et al. Reference Zhao, Xu, Zhu, Liu and Chen2014).
The Bogda Mountains extends over 360 km from east to west along the northern margin of eastern Tianshan. The oldest formation in the Bogda Mountains is Devonian strata, which are dominated by marine and terrigenous sediments, tuffaceous sandstone and volcanic rocks. Carboniferous strata are widely exposed in the Bogda Mountains and are in fault contact with the Devonian rocks (Fig. 1c). The Carboniferous strata are divided into three formations, namely, the Lower Carboniferous Qijiaojing Formation and the Upper Carboniferous Liushugou and Qijiagou formations (BGMRXUAR, 1993; Gu et al. Reference Gu, Hu, Yu, Zhao, Wu and Li2001; Xia et al. Reference Xia, Xia, Xu, Li, Ma and Wang2004; Liang et al. Reference Liang, Guo, Gao, Fan, Qin, Zhou and Huan2011). The Lower and Upper Carboniferous formations are separated by regional faults (Fig. 1c). The Lower Carboniferous Formation mainly consists of marine volcanic ignimbrites, tuffaceous sandstone and bimodal volcanic lavas, while the Upper Carboniferous Formation is dominated by marine (pillow) basaltic lava and felsic ignimbrites, with minor sandstone and siltstone. Permian strata are unconformably overlying the Carboniferous rocks. In this region, the Permian strata are mainly composed of terrestrial conglomerate, sandstone and siliceous mudstone intercalated with bimodal volcanic lavas. Jurassic clastic sediments occur in the SE of the study area and unconformably overlie the Permian strata (Fig. 1c; Carroll et al. Reference Carroll, Liang, Graham, Xiao, Hendrix, Chu and Mcknight1990; BGMRXUAR, 1993). Many approximately E–W-trending folds and faults are well developed in the Bogda Mountains. These folds mainly include the Miao’ergou anticline and Tonggou syncline, and a series of secondary E–W-trending faults are developed in the flanks of the folds (BGMRXUAR, 1993).
Late Palaeozoic intrusions crop out extensively in the Bogda Mountains (Fig. 1c), such as the Daheyan intrusion which is situated in the SW part of the Bogda Mountains. Zircon U–Pb ages indicate that intrusions in the Bogda Mountains are mostly of late Carboniferous (Sun et al. Reference Sun, Li, Gao and Yang2005; Han et al. Reference Han, Guo, Zhang, Zhang, Chen and Song2010; Li et al. Reference Li, Liu, Zhu, Chen, Jin, Xu and Chen2013; Si et al. Reference Si, Su, Yang, Zhang and Yang2014; Wang et al. Reference Wang, Chen, Ma and Yan2015; Lei et al. Reference Lei, Guo, Ma, Xiao, Li, Liu and Li2016a) and early Permian (Gu et al. Reference Gu, Hu, Yu, Li, Xiao and Yan2000; Yuan et al. Reference Yuan, Sun, Wilde, Xiao, Xu, Long and Zhao2010) in age. Late Carboniferous intrusive rocks consist of gabbro, diorite, granodiorite and granite. Early Permian intrusive rocks include diorite, monzogranite and granite. The Bogda Mountains hosts several Cu polymetallic deposits, for example, the Tonggou, Xingshugou, Miao’ergou and Weicaogou deposits. Re–Os isotopic analyses yield a chalcopyrite mineralization age of 303 ± 12 Ma (unpublished data), which indicates that the Tonggou deposit formed during late Carboniferous time. The Tonggou deposit shows a transition from a high-sulphidation epithermal to porphyry system; the Cu polymetallic mineralization may be associated with late Carboniferous magmatism.
3. Sample description and analytical methods
3.a. Sample description
Daheyan intrusions can be divided into Upper Daheyan and Lower Daheyan intrusions (Fig. 2). The Upper Daheyan intrusion lies 30 km east of Dabancheng, and mainly consists of syenogranite and diorite (Fig. 3). The Lower Daheyan intrusion lies 22 km east of Dabancheng, and mainly consists of granodiorite and monzonite (Fig. 3). In this study, we collected intrusive rocks from Daheyan intrusions, described in detail in the following.

Fig. 2. Geological map of the Daheyan area, southern Bogda Range (compiled from the 1:200,000 map by XBGMR 1988).
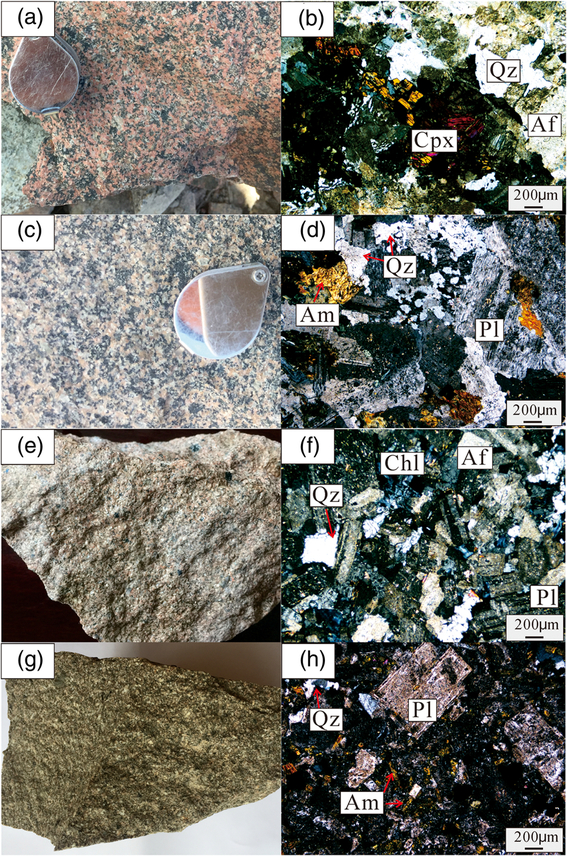
Fig. 3. Representative photographs of the magma rocks in hand specimen and thin-section in the Daheyan area. (a, b) syenogranite (D01); (c, d) diorite (D02); (e, f) granodiorite (D03); (g, h) monzonite (D04). Qtz – quartz; Or – orthoclase; Pl – plagioclase; Pth – perthite; Bt – biotite; Hbl – hornblende.
Syenogranite was collected from an intrusion in the north of Daheyan (Fig. 2). The syenogranite is flesh red in colour and displays a subhedral and allotriomorphic texture in the mass, shows a graphic texture on the local scale and possesses a massive structure (Fig. 3a). It consists of quartz (20%), plagioclase (25%), alkali-feldspar (50%, including orthoclase and perthite), clinopyroxene (3%) (Fig. 3b) and minor accessory minerals (2%) that include apatite and zoisite.
Diorite was collected from an intrusion in the north of Daheyan (Fig. 2). The diorite is light red in colour, displays a subhedral and allotriomorphic texture, possesses a massive structure (Fig. 3c), and consists of plagioclase (76%), hornblende (22%), quartz (1%) and biotite (5%) (Fig. 3d).
Granodiorite was collected from an intrusion in the west of Daheyan (Fig. 2). The granodiorite is grey white, and commonly has a subhedral and allotriomorphic texture, and shows a massive structure (Fig. 3e). It consists of quartz (10%), plagioclase (77%), alkali-feldspar (6%, including orthoclase and perthite), chlorite (4%) and biotite (2%) (Fig. 3f).
Monzonite was collected from an intrusion in the west of Daheyan (Fig. 2). The monzonite is grey black, and commonly has a subhedral and allotriomorphic texture, and shows a massive structure (Fig. 3g). It consists of alkali-feldspar (53%, including orthoclase and perthite), quartz (10%), plagioclase (32%), hornblende (8%) and quartz (4%) (Fig. 3h).
3.b. Analytical methods
3.b.1. Zircon U–Pb dating
Zircon U–Pb ages were determined for samples from the Daheyan area (Fig. 2). Four samples were crushed, and zircon grains and cathodoluminescence (CL) images were generated at the Faith Geological Service Company, Langfang, Hebei province, China. U–Pb–Hf isotopic analyses of the zircons were performed at the Yanduzhongshi Geological Analysis Laboratories Ltd, Beijing. Zircon grains were mounted on epoxy blocks and polished to expose grain centres. CL images are shown in Figure 4. U–Pb dating and Hf isotopic analyses of zircons were obtained using an Agilent 7500a inductively coupled plasma (ICP) mass spectrometer (MS) and GeoLas 2005 laser ablation system. 206Pb/238U ratios were calculated by ICPMSDataCal (Liu et al. Reference Liu, Hu, Gao, Günther, Xu, Gao and Chen2008, Reference Liu, Hu, Zong, Gao and Shan2010), while the concordia age diagram and probability density plots of detrital zircon were obtained with Isoplot 3.0 (Ludwig, Reference Ludwig2003).

Fig. 4. Zircon concordia diagrams and weighting diagrams for the four igneous rock samples in the Daheyan region.
3.b.2. Major and trace elements
Twelve samples of major, trace and rare earth elements were measured and analysed at ALS Minerals-ALS Chemex, Guangzhou. The samples were crushed in a steel jaw crusher and then powdered to 200 mesh in an agate mill. Major-element compositions were analysed using ME-XRF06 X. Trace and rare earth element compositions were analysed using ME-MS81 and ME-MS82.
4. Results
4.a. Zircon U–Pb dating
Syenogranite zircons exhibit oscillatory or planar zoning under CL, as is typical of magmatic zircons (Fig. 4a). The results of U–Pb dating for 30 zircons, collected from the syenogranite (sample D01) in the Daheyan area, are listed in Table 1. Th/U ratios range from 0.34 to 0.60, indicating that the origin of the zircons is magmatic (Hoskin & Black, Reference Hoskin and Black2000). Analysis reveals that 26 of the samples plot near the concordia curve (Fig. 4a, b). 206Pb/238U ages range from 304 to 313 Ma, with a mean of 307.7 ± 1.2 Ma (MSWD = 0.74), which represents the crystallization age of the syenogranite. In addition, one of the samples has a 206Pb/238U age of 339 Ma, which represents the crystallization age of zircons captured or entrained from the wall rock during magma ascent. The other three measured points deviate from the concordia curve.
Table 1. LA-ICP-MS U–Pb data on zircons from the Daheyan intrusion

Diorite zircons exhibit oscillatory or planar zoning under CL, as is typical of magmatic zircons (Fig. 4c). The results of U–Pb dating for 24 zircons, collected from the diorite (sample D02) in the Daheyan area, are listed in Table 1. Th/U ratios range from 0.44 to 1.11, indicating that the origin of the zircons is magmatic (Hoskin & Black, Reference Hoskin and Black2000). Analysis reveals that nine of the samples plot near the concordia curve (Fig. 4c, d). 206Pb/238U ages range from 308 to 312 Ma, with a mean of 310.6 ± 1.5 Ma (MSWD = 0.22), which represents the crystallization age of the diorite. In addition, 11 of the samples have 206Pb/238U ages varying from 324 to 327 Ma, which represents the crystallization age of zircons captured or entrained from the wall rock during magma ascent. The other four measured points deviate from the concordia curve.
Granodiorite zircons exhibit oscillatory or planar zoning under CL, as is typical of magmatic zircons (Fig. 4e). The results of U–Pb dating for 30 zircons collected from the granodiorite (sample D03) in the Daheyan area are listed in Table 1. Th/U ratios range from 0.23 to 0.75, indicating a magmatic origin for the zircons (Hoskin & Black, Reference Hoskin and Black2000). Analysis reveals that 20 of the samples plot near the concordia curve (Fig. 4e, f). 206Pb/238U ages range from 301 to 305 Ma, with a mean of 303.3 ± 1.3 Ma (MSWD = 0.28), which represents the crystallization age of the granodiorite. In addition, six of the samples have 206Pb/238U ages that vary from 354 to 360 Ma, which represents the crystallization age of zircons captured or entrained from the wall rock during magma ascent. The other four measured points deviate from the concordia curve.
Monzonite zircons exhibit oscillatory or planar zoning under CL, as is typical of magmatic zircons (Fig. 4g). The results of U–Pb dating for 30 zircons collected from the monzonite (sample D03) in the Daheyan area are listed in Table 1. Th/U ratios range from 0.41 to 0.78, indicating a magmatic origin for the zircons (Hoskin & Black, Reference Hoskin and Black2000). Analysis reveals that 21 of the samples plot near the concordia curve (Fig. 4g, h). 206Pb/238U ages range from 302 to 307 Ma, with a mean of 303.8 ± 1.6 Ma (MSWD = 0.12), which represents the crystallization age of the monzonite. In addition, 206Pb/238U ages of some samples are 326, 327, 351 and 361 Ma, which represents the crystallization age of zircons captured or entrained from the wall rock during magma ascent. The other five measured points deviate from the concordia curve.
4.b. Major- and trace-element geochemistry
4.b.1. Major elements
Syenogranite shows SiO2 enrichment (70.18–70.87 wt%), high K2O + Na2O values (9.37–9.50 wt%), and Na2O/K2O = 0.88–1.11, with Al2O3 values of 13.34–13.43 wt%, total Fe2O3 values of 3.48–3.70 wt%, Mg number (100Mg2+/(Mg2++ TFe2+)) of 5–19, and CaO values of 0.92–1.14 wt% (Table 2). All samples plot in the granite field on a SiO2 versus K2O+Na2O diagram (Fig. 5a). The Rittmann index (σ) varies from 3.46 to 3.56 and, because of their high K2O + Na2O values, all samples plot in the high-K calc-alkaline and shoshonite series range on a SiO2 versus K2O diagram (Fig. 5b). The A/CNK values range from 0.89 to 0.92 and plot in the metaluminous field on an A/CNK versus A/NK diagram (Fig. 5c).
Table 2. Major (%) and trace element (μg g–1) data for the intrusion in the Daheyan area. LREE – light rare earth element; HREE – heavy rare earth element


Fig. 5. (a) SiO2 versus total alkali (Na2O + K2O) diagram; (b) SiO2 versus K2O diagram; and (c) A/CNK versus A/NK diagram for the Daheyan region. SiO2 versus total alkali (Na2O + K2O), SiO2 versus K2O and A/CNK versus A/NK diagrams are from Middlemost (Reference Middlemost1994), Peccerillo & Taylor (Reference Peccerillo and Taylor1976) and Maniar & Piccoli (Reference Maniar and Piccoli1989), respectively.
Diorite has SiO2 = 61.22–61.70 wt%, Al2O3 = 14.67–14.90 wt%, total Fe2O3 = 7.08–7.14 wt%, Mg no. = 33.7–33.8, CaO = 4.22 wt%, K2O = 0.14–0.42 wt% and Na2O = 7.55–7.76 wt% (Table 2). All samples plot in the monzonite field on a SiO2 versus K2O+Na2O diagram (Fig. 5a). The Rittmann index (σ) varies from 3.92 to 4.00 and, because of their high Na2O values, all samples plot in the tholeiite series range on a SiO2 versus K2O diagram (Fig. 5b). The A/CNK values approach 0.71 and plot in the metaluminous field on an A/CNK versus A/NK diagram (Fig. 5c).
Granodiorite shows high SiO2 (68.14–68.67 wt%), K2O (2.60–3.11 wt%) and Al2O3 (15.54–15.92 wt%). Na2O values are 5.53–6.14 wt%, total Fe2O3 = 3.01–3.48 wt%, Mg no. = 29–33 and CaO = 1.12–1.60 wt% (Table 2). All samples plot in the quartz monzonite field on a SiO2 versus K2O + Na2O diagram (Fig. 5a). The Rittmann index (σ) varies from 2.99 to 3.29 and, because of their high Na2O values, all samples plot in the high-K calc-alkaline and calc-alkaline series range on the SiO2 versus K2O diagram (Fig. 5b). A/CNK values range from 1.05 to 1.08 and plot in the peraluminous field on an A/CNK versus A/NK diagram (Fig. 5c).
Monzonite has and SiO2 value of 61.50 wt%, K2O of 2.86 wt%, Al2O3 of 14.68 wt%, Na2O of 5.42 wt%, total Fe2O3 of 7.61 wt%, Mg no. 32 and CaO of 3.74 wt% (Table 2). Samples plot in the monzonite field on a SiO2 versus K2O+Na2O diagram (Fig. 5a). The Rittmann index (σ) is 3.97 and, due to their high Na2O, monzonite sample plots in the high-K calc-alkaline series range on a SiO2 versus K2O diagram (Fig. 5b). The A/CNK value is 0.78 and plots in the metaluminous field on an A/CNK versus A/NK diagram (Fig. 5c).
4.b.2. Trace elements
Syenogranite samples are slightly enriched in light rare earth elements (LREEs), depleted in heavy rare earth elements (HREEs) (LREE/HREE = 4.21–4.61; (La/Yb)N = 3.14–3.57) and show clear negative Eu anomalies (δEu = 0.34–0.36) on a chondrite-normalized rare earth element (REE) diagram (Fig. 6a; Table 2). A primitive-mantle-normalized trace-element diagram indicates that the rock is enriched in incompatible elements (e.g. Th, U, Zr and Hf) and some large-ion lithophile elements (LILE; e.g. K and Rb), and is depleted in high-field-strength elements (HFSE; e.g. Ta, Nb, Ti and Sr) (Fig. 6b).

Fig. 6. Chondrite-normalized REE pattern and primitive-mantle-normalized spider diagrams of igneous rocks in Daheyan. Chondrite and primitive mantle values used for normalization are from Boynton (Reference Boynton and Henderson1984) and Sun & McDonough (Reference Sun, McDonough, Saunders and Norry1989).
Diorite samples are slightly enriched in LREEs, depleted in HREEs (LREE/HREE = 3.89–4.15; (La/Yb)N = 3.05–3.46), and show weak negative Eu anomalies (δEu = 0.63–0.67) on a chondrite-normalized REE diagram (Fig. 6c; Table 2). The primitive-mantle-normalized trace-element diagram indicates that the rock is enriched in incompatible elements (e.g. Th, U, Zr and Hf) and some LILEs (e.g. K, Rb, Sr and Ba), and depleted in HFSEs (e.g. Ta, Nb and Ti) (Fig. 6d).
Granodiorite samples are obviously enriched in LREEs and depleted in HREEs (LREE/HREE = 9.54–10.41; (La/Yb)N = 9.05–10.53). Granodiorite shows no negative Eu anomalies (δEu = 0.94–0.99) on the chondrite-normalized REE diagram (Fig. 6e; Table 2). The primitive-mantle-normalized trace-element diagram indicates that the rocks are enriched in incompatible elements (e.g. Th, U, Zr and Hf) and some LILEs (e.g. Rb), and depleted in HFSEs (e.g. Ta, Nb and Ti) (Fig. 6f).
Monzonite samples are slightly enriched in LREEs and depleted in HREEs (LREE/HREE = 9.54–10.41; (La/Yb)N = 9.05–10.53). Monzonite shows weak negative Eu anomalies (δEu = 0.65) on the chondrite-normalized REE diagram (Fig. 6g; Table 2). The primitive-mantle-normalized trace-element diagram indicates that the rock is enriched in incompatible elements (e.g. Th, U, Zr and Hf) and some LILEs (e.g. K and Rb), and depleted in HFSEs (e.g. Ta, Nb, Ti and Sr) (Fig. 6d).
4.c. Hf isotopes
In situ Hf isotopic compositions of zircon from the syenogranite, diorite, granodiorite and monzonite are listed in Table 3.
Table 3. Zircon Lu–Hf isotopic data for the Daheyan intrusion from the Bogda orogenic belt

The 24 analyses of sample D01 from syenogranite display variable initial Hf isotopic compositions, with 176Hf/177Hf = 0.0009–0.0023, and ϵ Hf (t) values of +11.2 to +14.4. The corresponding single-stage model ages (T DM1) range from c. 367 Ma to c. 499 Ma and two-stage model ages (T DM2) range from c. 400 Ma to c. 606 Ma.
Twenty-five analyses of sample D02 from diorite display 176Hf/177Hf ratios of 0.0012–0.0119 and ϵ Hf (t) values of +4.3 to +14.7. The corresponding T DM1 is c. 362–941 Ma and the corresponding T DM2 is c. 389–1048 Ma.
The 19 analyses of sample D03 from granodiorite display 176Hf/177Hf ratios of 0.0007–0.0032 and ϵ Hf (t) values of +7.6 to +15.6. The corresponding T DM1 is c. 316–702 Ma, whereas the T DM2 is c. 322–872 Ma.
Twenty-five in situ Hf isotopic analyses of sample D04 from monzonite exhibit variable initial Hf isotopic compositions, resulting in 176Hf/177Hf ratios of 0.0006–0.0016 and ϵ Hf (t) of +11.0 to +15.8. T DM1 values are c. 311–502 Ma and T DM2 values are c. 313–617 Ma.
5. Discussion
5.a. Timing of magmatism in the Daheyan area, Bogda Mountains
The LA-ICP-MS zircon U–Pb ages for the syenogranite, diorite, granodiorite and monzonite in the Daheyan area are 307.7 ± 1.2, 310.6 ± 1.5, 303.3 ± 1.3 and 303.8 ± 1.6 Ma, respectively, which are consistent with some volcanic rocks in the Bogda Mountains. Wali et al. (Reference Wali, Wang, Cluzel and Zhong2018) reported LA-ICP-MS zircon U–Pb ages of 298 ± 8 Ma for rhyolite of the Daheyan area, and Xie et al. (Reference Xie, Xu, Chen, Luo, Hong, Ma and Liu2016b) reported a LA-ICP-MS zircon U–Pb age of 311 ± 2 Ma for dacite ignimbrite from Baiyanggou, Bogda Mountains. The similarity between these ages shows they were derived from the same late Carboniferous magmatic events.
5.b. Petrogenesis of Upper Daheyan intrusions
5.b.1 Syenogranite
Syenogranite is enriched in SiO2, slightly alkaline and low in Al2O3. It shows Ti, Ba, P and Sr negative anomalies, and displays clear negative Eu anomalies. This syenogranite has the chemical characteristics of A-type granite, which is characteristically enriched in SiO2 and K2O, shows Ba and Sr anomalies, and exhibits clearly negative Eu anomalies (Zhang et al. Reference Zhang, Parrish, Zhang, Xu, Yuan, Gao and Crowley2007). In addition, the value of Zr + Ce + Nb + Y ranges from 589 to 624 × 10–6, and approaches the related value of A-type granites (>350 × 10–6; Whalen et al. Reference Whalen, Currie and Chappell1987). Moreover, we calculated the Zr saturation temperature of the syenogranite, revealing high crystallization temperatures (929–938°C, calculated from the equation of Watson & Harrison, Reference Watson and Harrison1983), which also suggests that syenogranite is an A-type granite. The zircon ϵ Hf (t) values of the syenogranite vary from 11.2 to 14.4 (average of 12.8). On the ϵ Hf (t) versus age diagram, all samples plot below the depleted mantle reference line and above the CHUR reference line (Fig. 7), suggesting that syenogranite was mainly derived from the melting of young crust with some contribution from mantle-derived materials. In addition, the two-stage Hf model ages (T DM2) of syenogranite are 400–609 Ma, also indicating that syenogranite was mainly derived from the melting of juvenile crust.

Fig. 7. Zircon U–Pb ages versus ϵ Hf(t) plots for the upper Carboniferous magmatic rocks of the Daheyan region.
5.b.2 Diorite
Chemical compositions of Daheyan diorite suggest they are tholeiite metaluminous diorites (Fig. 5b, c) that can be produced by partial melting of mafic lower crust (Jung et al. Reference Jung, Hoernes and Mezger2002). Moreover, experimental studies have shown that dehydration melting of crustal (basaltic and pelitic) rocks would produce low Mg (generally < 40) melts, regardless of the melting degrees (Patiño Douce & Johnston, Reference Patiño and Johnston1991; Rapp & Watson, Reference Rapp and Watson1995). The Daheyan diorite has low Mg values of 33.7–33.8, also indicating it was mainly derived from crust rather than mantle material. Zircon ϵ Hf (t) values of diorite are 4.3–14.7 with a wide range of values, indicating that the primary magma of the diorite was derived from multiple sources. On the ϵ Hf (t) versus age diagram, all samples plot below the depleted mantle reference line and above the CHUR reference line (Fig. 7), suggesting that diorite was derived from the melting of young crust with some contributions from mantle-derived materials. In addition, the two-stage Hf model ages (T DM2) of diorite vary from 389 to 1048 Ma. The T DM2 of diorite is older than that of syenogranite, which indicates that primary magma of diorite was derived from juvenile crust and some mixed mantle materials.
In brief, the studied syenogranite is an A-type granite, and the studied diorite is a low-Mg diorite with tholeiite and metaluminous features. The syenogranite and diorite both resulted from late Carboniferous magmatism with ages of 307.7 ± 1.2 Ma and 310.6 ± 1.5 Ma, respectively. On the other hand, samples of syenogranite and diorite have different trace-element patterns (Fig. 6) and diverse negative Eu anomalies; moreover, the diorite has a wider range of ϵ Hf (t) values than the syenogranite. These features imply that syenogranite and diorite have disparate magma source characteristics; the primary magma of syenogranite was sourced from juvenile crust, while the primary magma of diorite was derived from juvenile crust and mixed mantle materials.
5.c. Petrogenesis of Lower Daheyan intrusions
5.c.1. Granodiorite
The granodiorite is characteristically enriched in SiO2, has a high value of Na2O and CaO, has relatively low K2O values and is weakly peraluminous. Moreover, the value of Zr + Ce + Nb + Y ranges from 219 to 242 × 10–6, below the lower limit of A-type granites (> 350 × 10–6; Whalen et al. Reference Whalen, Currie and Chappell1987). Moreover, we calculated the Zr saturation temperature of the granodiorite, revealing high crystallization temperatures (827–841°C; Watson & Harrison, Reference Watson and Harrison1983), significantly lower than that of typical A-type granites (> 900°C; Eby, Reference Eby1990, Reference Eby1992). As observed through petrographic microscopy, granodiorite contains hornblende minerals; the granodiorite is therefore an I-type granite (Chappell & White, Reference Chappell and White1992; Searle et al. Reference Searle, Parrish, Hodges, Hurford, Ayres and Whitehouse1997; Wu et al. Reference Wu, Zhao, Sun, Wilde and Yang2007). Zircon ϵ Hf (t) values from granodiorite range from 7.6 to 14.5 (Table 3). On the ϵ Hf (t) versus age diagram, all samples plot below the depleted mantle reference line and above the CHUR reference line (Fig. 7), suggesting that granodiorite was mainly derived from melting of the crust with some contribution from mantle-derived materials. Devonian zircon xenocrysts in the Carboniferous granodiorite generally show positive ϵ Hf (t) values; a fact that suggests the possible existence of Devonian juvenile crustal rocks in the basement of the Bogda Mountains. Moreover, two-stage Hf model ages (T DM2) of granodiorite correspond to 427–872 Ma. These values are younger than those of basement rocks in the Cathaysia Block (1.8–2.2 Ga) (Chen et al. Reference Chen, Guo, Tang and Zhou1999), also indicating that the granodiorite was mainly derived from a juvenile crustal source.
5.c.2. Monzonite
Monzonite shows high Na2O and CaO values. However, it has relatively low K2O values, and is metaluminous. Moreover, we calculated the Zr saturation temperature of monzonite, which shows a high crystallization temperature (852°C; Watson & Harrison, Reference Watson and Harrison1983), significantly lower than that of typical A-type granites (> 900°C; Eby, Reference Eby1990, Reference Eby1992). In petrography, monzonite contains hornblende minerals; we therefore conclude that the monzonite is an I-type granite (Chappell & White, Reference Chappell and White1992; Searle et al. Reference Searle, Parrish, Hodges, Hurford, Ayres and Whitehouse1997; Wu et al. Reference Wu, Zhao, Sun, Wilde and Yang2007).
Zircon ϵ Hf (t) values of monzonite are 11.0–15.0 (Table 3). On the ϵ Hf (t) versus age diagram, all samples plot below the depleted mantle reference line and above the CHUR reference line (Fig. 7), suggesting that monzonite was mainly derived from the melting of young crust with some contribution from mantle–derived materials. Moreover, two-stage Hf model ages (T DM2) of monzonite vary from 357 to 617 Ma. These values are younger than those for basement rocks in the Cathaysia Block (1.8–2.2 Ga) (Chen et al. Reference Chen, Guo, Tang and Zhou1999), which also indicates that the monzonite was mainly derived from a Neoproterozoic crustal source.
Generally, samples of granodiorite and monzonite have similar LA-ICP-MS zircon U–Pb ages and Hf isotope data. However, they show differences in major- (e.g. Na2O, CaO) and trace-element patterns, and in δEu values (Figs 5, 6). This suggests that granodiorite and monzonite have similar magma source characteristics but have experienced different degrees of fractional crystallization. In addition, the mineralization age of the Tonggou deposit is 303 ± 12 Ma based on Re–Os isotopic analyses (unpublished data), which is consistent with those of Lower Daheyan intrusions. The Tonggou deposit and the Lower Daheyan intrusions (303 Ma) are therefore both related to late Carboniferous magmatism.
5.d. Tectonic setting of the Bogda Mountains
In recent years, many studies have been conducted on the Bogda Mountains (Xie et al. Reference Xie, Luo, Xu, Chen, Hong, Ma and Ma2016a, b, c; Wali et al. Reference Wali, Wang, Cluzel and Zhong2018) since it is a key area for understanding the Palaeozoic geodynamic evolution of the CAOB. There are different interpretations for the tectonic setting of the Bogda Mountains during Carboniferous time. Gu et al. (Reference Gu, Hu, Yu, Li, Xiao and Yan2000, Reference Gu, Hu, Yu, Zhao, Wu and Li2001), suggested an intracontinental rift environment based on bimodal volcanism, while Shu et al. (Reference Shu, Zhu, Wang, Faure, Charvet and Cluzel2005, Reference Shu, Wang, Zhu, Guo, Charvet and Zhang2011), believed that the Carboniferous volcanic arc was superimposed by the early Permian rift with bimodal volcanism and an olistostrome. In addition, Xie et al. (Reference Xie, Luo, Xu, Chen, Hong, Ma and Ma2016a, b, c) suggest an island-arc setting based on the presence of high-Al basaltic lava, while Wali et al. (Reference Wali, Wang, Cluzel and Zhong2018) support the theory of intra-arc extension based on their research on Carboniferous and lower Permian volcanic and sedimentary sequences of the Daheyan section. Previous studies mostly focus on the volcanic rocks in the Bogda Mountains, but relevant investigations on intrusions from the Bogda Mountains are limited. In this study, we present new geochronological and geochemical data for the intrusions from the southwestern part of the Bogda Mountains, and synthesize the available data for the Carboniferous magmatic rocks of the entire Bogda Mountains. The Daheyan syenogranite and coeval Sujishan granite in this belt both correspond to A-type granite (Lei et al. Reference Lei, Guo, Ma, Xiao, Li, Liu and Li2016a). A-type granites can be generated in diverse tectonic settings; however, the majority of A-type granites are affected by extension settings and are often associated with the generation of other rock types (e.g. I-type granites Wu et al. Reference Wu, Sun, Li, Jahn and Wilde2002, Reference Wu, Zhao, Sun, Wilde and Yang2007; Ma et al. Reference Ma, Liu, Wang, Tan, Qian, Qin, Feng, Sun and Zang2019). This is the case for the Permian stratigraphy of NE China, where A- and I-type rocks along the middle segment of the Hegenshan-Heihe Suture within the middle Great Xing’an Range were formed in a post-collisional extension setting (Ma et al. Reference Ma, Liu, Wang, Tan, Qian, Qin, Feng, Sun and Zang2019), indicating that Daheyan A- and I-type rocks may form in a post-collisional extension setting.
In addition, the tectonic setting of late Carboniferous intrusions in the Bogda Mountains can be made clearer by the following facts. First, late Carboniferous volcanic rocks of the Bogda-Harlik Orogenic Belt clearly show a linear distribution from basalt and andesite to dacite and rhyolite on the Zr/TiO2 versus Nb/Y diagram (Winchester & Floyd, Reference Winchester and Floyd1976; Fig. 8a), which indicates that these rocks correspond to the calc-alkaline series. Moreover, high-Al basaltic lava and I-type intrusions are well developed in the Bogda Mountains, indicating that this magmatism formed in an arc setting (Xie et al. Reference Xie, Xu, Chen, Luo, Hong, Ma and Liu2016b; this study). Second, most upper Carboniferous volcanic rocks consistently plot in the field of volcanic arcs (Fig. 8b), indicating that the upper Carboniferous volcanic rocks of the Bogda-Harlik Orogenic Belt show arc-type features and formed in a subduction zone (Chen et al. Reference Chen, Shu, Santosh and Zhao2013; Xie et al. Reference Xie, Luo, Xu, Chen, Hong, Ma and Ma2016a, b, c). Third, Proterozoic zircon xenocrysts in the Carboniferous and lower Permian magmatic rocks generally show positive ϵ Hf (t) values (Wali et al. Reference Wali, Wang, Cluzel and Zhong2018; this study), suggesting the possible existence of Proterozoic juvenile crustal rocks in the basement of the Bogda Mountains and significant continental crustal growth during Carboniferous–Permian time (Kröner et al. Reference Kröner, Kovach, Belousova, Hegner, Armstrong, Dolgopolova, Seltmann, Alexeiev, Hoffmann, Wong, Sun, Cai, Wang, Tong, Wilde, Degtyarev and Rytsk2014; Wali et al. Reference Wali, Wang, Cluzel and Zhong2018). As mentioned above, despite widespread changes in depositional environment from Carboniferous marine or shallow-marine environments to middle Permian terrigenous sedimentation (BGMRXUAR, 1993; Wali et al. Reference Wali, Wang, Cluzel and Zhong2018), a localized narrow deep-water basin appeared in a regionally shallow-marine environment during early Permian time (Shu et al. Reference Shu, Wang, Zhu, Guo, Charvet and Zhang2011). Based on these results, we consider that the Bogda-Harlik Orogenic Belt was still in an intra-arc extension related to a continent-based arc setting during late Carboniferous time. The Late Carboniferous Daheyan intrusions and Cu multi-metal mineralization in the Bogda Mountains therefore potentially formed in a continent-based arc setting.

Fig. 8. Geochemical classification diagrams for the upper Carboniferous volcanic rocks of the Bogda-Harlik Belt (Zhao et al. Reference Zhao, Xu, Zhu, Liu and Chen2014; Xie et al. Reference Xie, Xu, Luo, Liu, Hong and Ma2016c; Wang et al. Reference Wang, Ma, Chen and Yan2017; Wali et al. Reference Wali, Wang, Cluzel and Zhong2018): (a) Zr/TiO2 versus Nb/Y diagram (Winchester & Floyd, Reference Winchester and Floyd1976); and (b) Th–Zr/117–Nb/16 diagrams (Wood, Reference Wood1980).
6. Conclusions
(1) Late Carboniferous magmatism is intensive in the Daheyan region; rock types mainly include syenogranite, diorite, granodiorite and monzonite and ages are in the range 303–311 Ma.
(2) Syenogranite from Daheyan is an A-type granite, mainly derived from the melting of juvenile crust. Diorite from Daheyan is derived from young crust and some mixed mantle materials. Granodiorite and monzonite are both I-type granites, and sourced from the melting of juvenile crust.
(3) A comprehensive analysis of geochronological, geochemical and isotopic data of late Carboniferous magmatic rocks from the entire Bogda-Harlik Orogenic Belt suggest an intra-arc extension related to a continent-based arc setting.
Acknowledgements
We thank Editor Chad Deering and two anonymous reviewers for their constructive comments that led to the significant improvement of the manuscript. We are most grateful to the staff of the Yanduzhongshi geological analysis laboratories Ltd for LA-ICP-MS zircon U–Pb dating and Hf isotope analysis. We also thank the staff of the ALS Laboratory Group for geochemical analyses. This research was supported by the Natural Science Foundation of Xinjiang Province (grant no. 2018D01C042).