Mosquitoes, which belong to the Culicidae family, are a group of haematophagous vectors that carry and transmit many human viruses in nature. Most mosquito-borne human viruses, belonging to the Flaviviridae, Togaviridae and Bunyaviridae families (Ref. Reference Cheng1), are aetiological agents of severe human diseases such as haemorrhagic fever, biphasic fever, encephalitis, and meningitis (Ref. Reference Gaunt2). Hundreds of millions of infections are caused by mosquito-borne viruses annually (Refs Reference Weaver and Reisen3–Reference Leta5). Dengue virus (DENV, Flavivirus, Flaviviridae family), the most prevalent mosquito-borne virus worldwide transmitted by the Aedes aegypti and A. albopictus mosquitoes, is estimated to cause 390 million infections every year, of which approximately 500 000 are severe cases that require hospitalisation, with more than 20 000 cases lead to death, mostly in tropical countries (Ref. Reference Bhatt6). Recent Zika virus (ZIKV, Flavivirus, Flaviviridae family) outbreaks in the Pacific islands and the Americas have resulted in more than 223 000 confirmed cases by the end of 2017 (Refs 7, Reference Gao8). Several neurological complications, such as Guillain–Barré syndrome in adults and microcephaly in neonates, are associated with ZIKV infection (Ref. Reference Parra9). In addition, both West Nile virus (WNV, Flavivirus, Flaviviridae family) and chikungunya virus (CHIKV, Alphavirus, Togaviridae family) caused millions of infections in Americas in the past two decades. Although arboviruses have propagated to a vast region of the globe and posed major public health concerns (Fig. 1), there are no vaccines or therapeutics available against most mosquito-borne viruses. The lifecycle of mosquito-borne viruses involves survival in and transmission between two distinct host environments. The strategies employed by viruses at each stage are thus different. Understanding the triangular interplay between host, virus and mosquito in the arboviral lifecycle may provide novel strategies for limiting viral transmission and their prevalence in nature.

Fig. 1. Global distribution of arboviruses. The map depicts countries or territories with previous or current transmission of Dengue virus (a), Zika virus (b), chikungunya virus (c) and West Nile virus (d). The map was generated using a free online tool Mapchart with public data from WHO, CDC, PAHO and a review of literature (Refs Reference Iranpour10–Reference Ruckert13).
Mosquito-borne viruses naturally cycle between mosquitoes and vertebrate animals. As a natural vector, a mosquito incidentally feeds on a virus-infected host to acquire viruses circulating in the host blood. The viruses subsequently establish an infection in the mosquito's epithelial cells by overcoming the physical and immune barrier of the epithelia and then spread into the mosquito haemocoel. The viruses subsequently infect other tissues, such as the salivary glands, ovaries, and neural system. Thus, the infected mosquito becomes competent to transmit the viruses to naive hosts through blood feeding (Ref. Reference Cheng1) (Fig. 2). During their lifecycle, the viruses exploit many strategies enabling mosquitoes to carry and transmit them efficiently. Here, we provide an overview of recent progress on the underlying mechanisms by which the arboviruses complete their life journey.
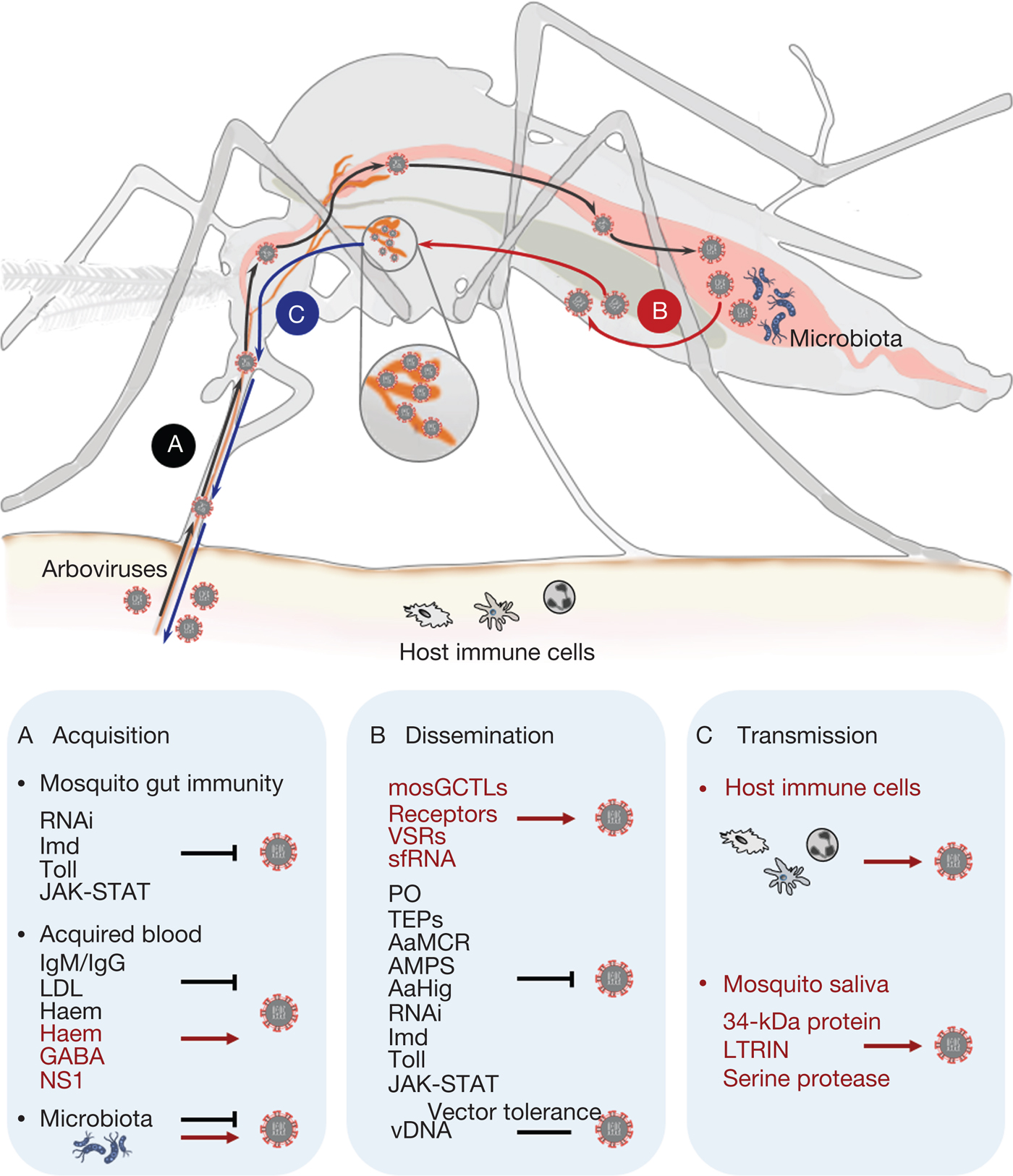
Fig. 2. Schematic overview of the arbovirus lifecycle in mosquito. (a) After acquisition of arbovirus from an infectious blood meal, the mosquito gut immunity, components of the host blood and the mosquito gut commensal microbiome regulate mosquito permissiveness to viral infection. Several evolutionarily conserved innate immune pathways, such as immune deficiency, Toll and Janus kinase-signal transduction and activators of transcription, as well as RNA interference, are key factors in inhibiting arbovirus replication in the mosquito gut epithelium. Host blood components either hinder the infection capacity (immunoglobulin M, immunoglobulin G or low-density lipopolyprotein), or enhance arbovirus infection by suppressing mosquito gut antiviral immunity (gamma-aminobutyric acid, flavivirus nonstructural protein-1). The commensal microbiome residing in the mosquito gut lumen also plays an important role in modulating vector susceptibility to arboviruses. (b) Arboviruses exploit vectorial or viral factors to facilitate systemic infection in mosquito tissues. Vectorial factors, such as mosquito galactose-specific C-type lectin-1, or other putative receptors and viral factors, such as viral suppressor against RNA silencing and subgenomic flaviviral RNA, can contribute to the spreading of arboviruses from the midgut epithelium into the haemolymph circulation. Mosquitoes utilise different strategies, such as phenoloxidase, thioester-containing proteins, A. aegypti macroglobulin complement-related factor, antimicrobial peptides, A. aegypti Hikaru genki and innate immune pathways, to interfere with arbovirus infection. Virus-derived DNA plays a role in tolerance of viral replication in mosquitoes, thus enabling effective transmission of arbovirus by mosquitoes in nature. (c) Arboviruses are transmitted by mosquito bites into human skin along with mosquito saliva. The intradermal immune cells, such as dendritic cell subsets, monocytes and macrophages, are considered primary permissive targets during the initial infection in hosts. Mosquito saliva has also been proven to facilitate arbovirus transmission by exploiting saliva factors, including the 34-kDa protein LTRIN and a serine protease, to enhance the infection of host cells.
From host to mosquito: arboviral acquisition by mosquitoes feeding on viraemic hosts
Arboviral acquisition, which is the process of viral transmission from an infected host to a mosquito vector, is an essential step in the viral lifecycle. The circulating viral particles in the infected hosts' blood are taken into the midgut by blood meal, thereby initiating an infection in the mosquitoes. The viruses must conquer the gut epithelial barrier to establish their infection in the epithelial cells, prior to systemic dissemination. The outcomes of arboviral acquisition are determined by multiple factors, such as the gut immunity, the components in the host blood and the gut resident commensal microbes.
As a haematophagous insect, mosquitoes take a large amount of blood from the infected host by blood meal. Therefore, the host blood components may modulate the immune and physiological status of the mosquito gut, thus regulating the mosquito's permissiveness to viral infection. Based on current knowledge, several host blood factors may modulate viral replication in the mosquito gut epithelium. For example, variations in the host immunological responses may correspond with the capacity for DENV acquisition from viraemic patients to A. aegypti mosquitoes. Increasing titres of immunoglobulin M and immunoglobulin G against DENV envelope proteins may contribute to a reduction in DENV infection in mosquitoes (Ref. Reference Nguyen14). Besides, low-density lipopolyprotein (LDL) is a highly abundant blood component whose expression is decreased during severe DENV infection. Indeed, LDL acquired from host blood can be endocytosed by the mosquito midgut epithelial cells and accumulate at the luminal epithelium during blood digestion, therefore hindering flavivirus acquisition in A. aegypti by blood meal (Ref. Reference Wagar15). In addition, a haemoglobin metabolite called haem can induce the expression of genes related to redox metabolism, thereby triggering large, variable expression of energy metabolism and immune genes in the mosquito gut to modulate the vectorial susceptibility to arboviruses (Ref. Reference Bottino-Rojas16). We recently found that the digestion of blood proteins generally facilitates replication of arboviruses through activation of GABAergic signalling in mosquitoes. Activation of the GABAergic system enhances the infection of arboviruses of the Flavivirus, Alphavirus and Orthobunyavirus genera by modulating the gut antiviral immunity. Gamma-aminobutyric acid (GABA) can be generated via decarboxylation of the amino acid glutamic acid. The ingestion of blood in the mosquito guts results in robust GABA production from glutamic acid derived from blood protein digestion, thereby facilitating viral replication of the mosquito vectors (Ref. Reference Zhu17). Altogether, the haematophagous nature of mosquitoes regulates arboviral acquisition by blood meal. In addition to the host blood components, the virus also utilises its own non-structural proteins secreted in host blood to efficiently overcome the hostile gut environment, thereby enabling viral acquisition. Flavivirus nonstructural protein-1 (NS1) is a virus-encoded nonstructural protein that is secreted into the blood of infected hosts. Circulating NS1 can be acquired by mosquitoes together with virions when they feed on a viraemic host. Indeed, flavivirus NS1 helps the viruses conquer the gut immune barrier by inhibiting gut immunity, such as reactive oxygen species production and the Janus kinase (JAK)-signal transduction and activators of transcription (STAT) pathway, thereby enabling efficient viral infection in mosquitoes (Refs Reference Liu18, Reference Liu19).
The mosquito gut is a pivotal natural entry site for arboviruses. The intricate ecological gut environment, including the gut immunity and resident gut commensal microbes, plays a role in modulating arbovirus infection in the gut epithelial cells. The gut antiviral mechanisms in mosquitoes have been well summarised in previous reviews (Refs Reference Cheng1, Reference Merkling and van Rij20, Reference Blair and Olson21). Briefly, RNA interference (RNAi) is deemed as a predominant antiviral mechanism in invertebrates. Recent studies have demonstrated that the RNAi system responds to many arboviral infections in mosquitoes. Suppression of the RNAi components resulted in higher viral replication in the mosquito guts (Refs Reference Blair and Olson21–Reference Sanchez-Vargas23). In addition, several evolutionarily conserved innate immune pathways, such as the Toll pathway, the immune deficiency (Imd) pathway and the JAK-STAT pathways, also play resistant roles against arbovirus replication in the gut epithelium. O'nyong'nyong virus (Alphavirus, Togaviridae family) infection in Anopheles gambiae was enhanced by knockdown of the Imd components in the mosquito midguts (Ref. Reference Carissimo24). Silencing of key components in the Toll (Myd88) and JAK-STAT (Dome and Hop) pathways significantly increased DENV replication (Ref. Reference Xi25), while silencing PIAS (a negative regulator of the JAK-STAT pathway) showed an opposite role (Ref. Reference Souza-Neto26) in DENV infection by blood meal. These pieces of evidence indicate the crucial roles of these mosquito antiviral pathways in controlling arboviral acquisition. In addition, the gut inhabitant commensal microbiome can modulate viral replication in the mosquito gut epithelia. During arboviral acquisition by blood meal, the viruses are taken into the mosquito gut, where an abundant commensal microbiome resides. Indeed, colonisation by a Chromobacterium species, a commensal gut bacterium isolated from field-caught A. aegypti, compromised mosquito vector competence to DENV infection (Ref. Reference Ramirez27). A gut commensal bacterium, Chromobacterium sp. Panama, presents its anti-DENV activity by secreting an aminopeptidase to degrade the DENV envelope protein, thus preventing DENV attachment and infection of the mosquito cells (Ref. Reference Saraiva28). In addition, oral introduction of a Proteus sp. strain, which was derived from the mosquito gut, led to an increase of resistance against DENV infection through up-regulation of the expression of antimicrobial peptide (AMP) genes in the gut epithelial cells (Ref. Reference Ramirez29). An entomopathogenic fungus, Beauveria bassiana, can activate the Toll and JAK-STAT pathway-controlled effector genes and shows a resistance role in DENV infection of A. aegypti (Ref. Reference Dong30). Conversely, oral introduction of Serratia odorifera, a gut bacterium isolated from field A. aegypti mosquitoes, rendered mosquitoes highly susceptible to DENV infection (Ref. Reference Apte-Deshpande31). A Talaromyces fungus identified in the A. aegypti gut promotes more permissive of mosquitoes to DENV infection, a phenotype that is attributed to the modulation of digestive enzymes and trypsin activity in the mosquito gut (Ref. Reference Anglero-Rodriguez32). Altogether, the complicated interplay among these aforementioned factors, including the gut immunity, the blood components and the gut commensal microbes, determines the outcomes of infection in mosquitoes after arboviral acquisition by blood meal.
Viral propagation in mosquito tissues
After their initial replication in the epithelia of the mosquito midgut, arboviruses spread into the haemolymph in the mosquito haemocoel. Haemolymph circulation facilitates systemic viral invasion into tissues such as the fat body, ovaries, salivary glands and neural system (Ref. Reference Franz33). Indeed, arboviruses utilise multiple strategies to complete their systemic infection in mosquito tissues, thereby enabling mosquitoes to carry and transmit the viruses efficiently (Ref. Reference Barrows34). Recent studies reported that multiple mosquito C-type lectins play susceptibility roles in dengue and WNV infection (Refs Reference Cheng35, Reference Liu36). An A. aegypti C-type lectin, mosquito galactose-specific C-type lectin-1 (mosGCTL-1), interacts with WNV in a calcium-dependent manner. This ‘mosGCTL-virus’ complex consequently interacts with the mosquito protein tyrosine phosphatase-1 (mosPTP-1), a mosquito homologue of human CD45 in A. aegypti, thus enabling viral attachment to the plasma membrane for viral entry (Ref. Reference Cheng35). In addition, another nine mosGCTL paralogues facilitate DENV infection in A. aegypti. These mosGCTLs interact with DENV envelope proteins to serve as susceptibility factors (Ref. Reference Liu36). Furthermore, several mosquito proteins, such as prohibitin (Ref. Reference Kuadkitkan37), heat-shock related protein (Ref. Reference Salas-Benito38), and laminin-binding protein (Ref. Reference Sakoonwatanyoo39), may act as putative receptors for DENV infection in mosquitoes. A putative cysteine-rich venom protein (CRVP379) can bind to mosquito prohibitin, thereby facilitating DENV replication in mosquitoes (Ref. Reference Londono-Renteria40). In addition, an evolutionarily conserved protein in both insects and mammalian hosts, natural resistance-associated macrophage protein (NRAMP), serves as a host cell surface molecule required for Sindbis virus (SINV, Alphavirus, Togaviridae family) binding and entry into Drosophila cells (Ref. Reference Rose41), suggesting a potential role for NRAMP in alphavirus infection of mosquitoes. Recently, mounting evidence suggested that arthropod-borne viruses encode antagonists of antiviral immune pathways in mosquitoes. A nonstructural protein encoded by the S-segment (NSs) of Bunyamwera virus has been proposed to act as a viral suppressor against RNA silencing (Ref. Reference Samuel42). NS4B protein of DENV interferes with processing of siRNAs by modulating host RNAi factors to favour virus replication (Ref. Reference Kakumani43). Analysis of CHIKV encoding proteins reveals that the non-structural proteins, nsP2 and nsP3, exhibit the suppressor activity against RNAi (Ref. Reference Mathur44). Besides the protein factors, non-coding subgenomic viral RNA also contributes to viral replication in mosquitoes. Flaviviruses can produce an abundant subgenomic flaviviral RNAs (sfRNAs) during infection in the host cells. sfRNA determines the infection and transmission rates of WNV in the Culex mosquitoes by overcoming the mosquito midgut barrier (Ref. Reference Göertz45). Besides, the flavivirus sfRNA directly interacts with the RNAi component to suppress RNAi in cultured cells and mosquitoes (Ref. Reference Moon46). In addition, DENV subgenomic RNA suppresses Toll-mediated immunity in the mosquito salivary glands to increase DENV replication, thereby promoting virus transmission by mosquitoes (Ref. Reference Pompon47).
Although the tissues in the mosquito haemocoel generally show permissiveness for arbovirus infection (Refs Reference Parikh48, Reference Goic49), mosquitoes must equip many cellular and humoural immune strategies to prevent the dramatic pathological sequelae caused by robust viral replication (Ref. Reference Cheng1). One of the humoural antiviral mechanisms in the haemolymph is the phenoloxidase (PO) cascade (Ref. Reference Christensen50). In this humoural immune cascades, the Prophenoloxidase (PPO) gene, which encodes a prototype of PO, is secreted into the mosquito haemolymph (Ref. Reference Cerenius and Soderhall51). The formation of melanin mediated by the PO cascades can be activated by Semliki Forest virus (SFV, Alphavirus) infection in A. albopictus U4.4 cells. Inhibition of PO activity facilitated both SFV replication in U4.4 cells and viral burden and mortality in A. aegypti (Ref. Reference Rodriguez-Andres52). In addition to the PO cascade, a recent study reported extracellular pattern recognition mechanisms mediated by complement-like proteins suppress flavivirus infection of A. aegypti (Refs Reference Xiao53, Reference Xiao54). A group of thioester-containing proteins (TEPs) in mosquitoes shares evolutionary conservation with the mammalian C3 protein (Refs Reference Blandin55, Reference Shokal and Eleftherianos56). In the mosquito haemolymph, a member of the TEP family, named as macroglobulin complement-related factor (AaMCR), shows antiviral immune activity against DENV infection (Refs Reference Cheng35, Reference Xiao54). However, AaMCR is incapable of directly interacting with the viral surface; instead, AaMCR employs a scavenger receptor with complement control protein domains to bind DENV virions, subsequently suppressing viral infection by inducing multiple AMPs. In the mosquito nervous system, a neuron-specific complement-related factor, designated Hikaru genki (Hig), controls both DENV and Japanese encephalitis virus replication by interfering with viral entry (Ref. Reference Xiao53). In addition to the humoural antiviral systems, immune signalling cascades in tissues (i.e. the RNAi, Toll, Imd and JAK-STAT pathways) may also limit viral replication by inducing AMPs or other antiviral effectors (Ref. Reference Kingsolver57). Overall, as a result of the delicate balance between viral action and mosquito counteraction, the viruses can efficiently replicate and spread in the mosquito tissues without eliciting significant adverse effects. In nature, this kind of mutual adaptation makes mosquitoes an ideal reservoir for many human viruses.
Mosquitoes bear long-lasting viral infections without substantial deleterious effects. The previous studies found that the virus-derived DNA (vDNA) plays an essential role of mosquito vector tolerance to arboviral infection, thereby facilitating arbovirus dissemination and transmission (Ref. Reference Goic49). Indeed, insects have a sophisticated systemic RNAi-based immunity mediated by macrophage-like haemocytes. Haemocytes take up dsRNA from infected cells, and then produce virus-derived complementary DNAs (vDNA) through endogenous transposon reverse transcriptases. These vDNAs template de novo synthesis of secondary viral siRNAs, which are secreted in exosome-like vesicles RNA viruses produce vDNAs through the activity of endogenous retrotransposons. These vDNAs boost the RNAi-mediated antiviral immune response and are indispensable for establishing persistent viral infections in Drosophila melanogaster (Refs Reference Tassetto58, Reference Goic59).
From mosquito to host: arbovirus transmission by the mosquito blood meal
After robust replication in the mosquito salivary glands, viruses are now ready for transmission via the next mosquito blood meal. Mosquitoes transmit the viruses by intra-dermal injection of the infectious particles into hosts together with some mosquito saliva proteins. The intradermal immune cells, such as dendritic cell subsets (Ref. Reference Cerny60), monocytes (Ref. Reference Schmid and Harris61) and macrophages (Refs Reference Cerny60, Reference Hamel62, Reference Blackley63), are regarded as the predominant permissive targets for the initial arbovirus infection in hosts. After the primary replication in these cells, the viruses spread into the host blood circulation for further systemic dissemination. Indeed, mosquito saliva can facilitate arbovirus transmission to hosts and contributes to the subsequent disease sequelae. For example, inoculation of WNV with mosquito salivary gland extract led to a higher viraemia and faster neuroinvasion compared with that of WNV inoculation by needles (Ref. Reference Styer64). In addition, mice bitten by DENV-infected mosquitoes showed higher and sustained viraemia compared with that of animals with direct DENV injection (Ref. Reference Cox65). Indeed, the discovery of mosquito saliva factors in arboviral transmission has received great attention due to their potential exploitation for disease transmission control. A 34-kDa protein identified from A. aegypti saliva can enhance DENV replication in human keratinocytes by suppressing interferon signalling (Ref. Reference Surasombatpattana66). Mosquito salivary gland extracts modulate antiviral activity and cytokine responses to enhance SINV infection (Ref. Reference Schneider67). Recently, a study found that an A. aegypti salivary factor, LTRIN, facilitates ZIKV transmission by interfering with nuclear factor-κB signalling and the downstream inflammatory cytokine production (Ref. Reference Jin68). In addition to regulating immune responses, salivary proteins can also influence viral entry into host cells. A serine protease in A. aegypti saliva augmented DENV infection by proteolysing extracellular matrix proteins, thereby increasing viral attachment to heparan sulphate proteoglycans and inducing cell migration (Ref. Reference Conway69). Interestingly, the oedema caused by mosquito saliva may promote viral transmission by enhancing the infection in the host's virus-permissive neutrophils and myeloid cells (Ref. Reference Pingen70). In addition, mosquito saliva may dysregulate antiviral signalling of antigen-presenting cells to reduce T lymphocyte and antiviral activities at the inoculation site, therefore supporting WNV replication (Ref. Reference Schneider71). Taken together, these observations show that arboviruses comprehensively utilise mosquito salivary proteins to enhance their transmission from infected vectors to naive hosts.
Concluding remarks
Mosquito-borne viruses maintain their lifecycle between permissive hosts and mosquito vectors. The viruses have evolved sophisticated strategies to sequentially accomplish their complicated life journey in mosquitoes, such as overcoming the barriers in the mosquito midguts, robustly disseminating and replicating in the tissues, and finally moving to a permissive host by blood feeding. Mosquitoes have multiple immune systems to limit invasion of these viral pathogens, although the viruses still successfully exploit either host or vectorial factors to achieve their efficient replication and transmission. Although our understanding of the interactions among mosquitoes, viruses and hosts has rapidly expanded, there are numerous puzzling questions that must be studied further. The major remaining challenge is to understand the mechanisms that regulate the permissiveness of mosquitoes to viral infection. The mechanisms that control viral infection in mosquitoes, including vectorial genetic status, host blood components, gut microbiota and environmental variations, are still poorly understood. Additionally, additional mosquito salivary factors that facilitate viral transmission must be further identified. Interplay between host immunity and mosquito salivary proteins should also be a target for future investigation. Furthermore, an interesting scientific question is how mosquitoes successfully control persistent arbovirus replication without dramatic pathological sequelae in the mosquito tissues. Altogether, an understanding of the interplay between mosquito-borne viruses and their mosquito vectors will provide us with a better understanding of the basis of arboviral survival and may provide novel strategies for limiting arboviral transmission in nature.
Author ORCIDs
Gong Cheng 0000-0001-7447-5488.
Acknowledgements and funding
This study was supported by grants from the National Key Research and Development Plan of China (2016ZX10004001-008, 2016YFC1201000 and 2016YFD0500400), the National Natural Science Foundation of China (81730063, 81571975 and 31825001), and the Shenzhen San-Ming Project for prevention and research on vector-borne diseases (SZSM201611064).