1. Introduction
The presence, and sometimes abundance, of bedding plane discoidal structures in Ediacaran marine strata has been historically reported as the imprints and moulds of soft-bodied organisms and regarded as important elements of Ediacaran fossil assemblages (Sprigg, Reference Sprigg1947; Sun, Reference Sun1986; Gehling et al. Reference Gehling, Narbonne and Anderson2000; MacGabhann, Reference MacGabhann2007). Preserved in positive or negative relief on the top or base of sedimentary beds, these structures were first interpreted as fossils of medusoid animals of cnidarian affinity (Sprigg, Reference Sprigg1947; Wade, Reference Wade1969; Sun, Reference Sun1986) but later interpreted as mostly representing moulds and casts of holdfast structures of various epibenthic organisms (Ford, Reference Ford1958; Jenkins & Gehling, Reference Jenkins and Gehling1978; Gehling et al. Reference Gehling, Narbonne and Anderson2000, Tarhan et al. Reference Tarhan, Droser and Gehling2010, Reference Tarhan, Droser and Gehling2015). Studies of the past two decades have highlighted the importance of taphonomic assessment in the interpretation of these morphologically simple forms, with several taxa considered to represent taphonomic variants within the form genus Aspidella (Gehling et al. Reference Gehling, Narbonne and Anderson2000; Tarhan et al. Reference Tarhan, Droser and Gehling2010, Reference Tarhan, Droser and Gehling2015).
The preservation of many of these taphomorphs (as well as of other taxa in distinct Ediacaran fossil assemblages) is argued as being strongly influenced by microbial processes and the presence of well-developed matgrounds on Ediacaran seafloors (Gehling, Reference Gehling1999; Narbonne, Reference Narbonne2005; Laflamme et al. Reference Laflamme, Schiffbauer, Narbonne and Briggs2011; Liu, Reference Liu2016; Liu et al. Reference Liu, McMahon, Matthews, Still and Brasier2019, Droser et al. Reference Droser, Tarhan, Evans, Surprenant and Gehling2020; but see Tarhan et al. Reference Tarhan, Hood, Droser, Gehling and Briggs2016; Bobrovskiy et al. Reference Bobrovskiy, Krasnova, Ivantsov, Luzhnaya (Serezhnikova) and Brocks2019 and Slagter et al. Reference Slagter, Tarhan, Hao, Planavsky and Konhauser2020 for alternative interpretations). Indeed, benthic microbial communities, rich in filamentous components and extracellular polymeric substances (EPS), the cohesiveness of surficial sediments increases, protecting them from erosion (Noffke et al. Reference Noffke, Gerdes, Klenke and Krumbein1996, Reference Noffke, Gerdes, Klenke and Krumbein2001, Reference Noffke, Gerdes and Klenke2003; Porada & Bouougri, Reference Porada and Bouougri2007; Callow & Brasier, Reference Callow and Brasier2009; Laflamme et al. Reference Laflamme, Schiffbauer, Narbonne and Briggs2011). Similarly, events of rapid burial can lead to the early diagenetic formation of authigenic clay and/or iron sulphide minerals in these matgrounds, further enhancing the preservation of fossils (Gehling, Reference Gehling1999; Narbonne, Reference Narbonne2005; Laflamme et al. Reference Laflamme, Schiffbauer, Narbonne and Briggs2011; Liu, Reference Liu2016), trace fossils (Jensen et al. Reference Jensen, Droser and Gehling2005; Buatois & Mángano, Reference Buatois, Mángano, Noffke and Chafetz2011, Reference Buatois, Mángano, Mángano and Buatois2016) and physical abiotic structures (Tarhan, Reference Tarhan2018) present at or near the sediment–water interface.
Throughout the geological record, these microbially influenced processes have conditioned the development and preservation of a whole class of sedimentary features, collectively known as MISS (microbially induced sedimentary structures) (Noffke et al. Reference Noffke, Gerdes, Klenke and Krumbein1996, Reference Noffke, Gerdes and Klenke2003; Porada & Bouougri, Reference Porada and Bouougri2007), including: (1) wrinkled and pustular textures (Hagadorn & Bottjer, Reference Hagadorn and Bottjer1997; Porada & Bouougri, Reference Porada and Bouougri2007); (2) intricate linear and branching structures (e.g. arumberiamorphs, Kolesnikov et al. Reference Kolesnikov, Grazhdankin and Maslov2012, Reference Kolesnikov, Danelian, Gommeaux, Maslov and Grazhdankin2017); or (3) different types of discoidal features (Menon et al. Reference Menon, McIlroy, Liu and Brasier2015, Reference Menon, McIlroy and Brasier2016). Based on their morphology, preservation and sedimentary context, this last category has been interpreted as (a) bacterial colonial structures (Grazhdankin & Gerdes, Reference Grazhdankin and Gerdes2007; Ivantsov et al. Reference Ivantsov, Gritsenko, Konstantinenko and Zakrevskaya2014; Bobrovskiy et al. Reference Bobrovskiy, Hope, Krasnova, Ivantsov and Brocks2018); (b) the product of mat decay-related processes (Dornbos et al. Reference Dornbos, Noffke, Hagadorn, Schieber, Bose, Eriksson, Banjeree, Sarkar, Altermann and Catuneau2007; Tu et al. Reference Tu, Chen, Retallack, Huang and Fang2016); (c) the result of build-up, migration and expulsion of fluids (Sun, Reference Sun1986; Gerdes et al. Reference Gerdes, Klenke and Noffke2001; Hagadorn & Miller, Reference Hagadorn and Miller2011; Taj et al. Reference Taj, Aref and Schreiber2014; Menon et al. Reference Menon, McIlroy, Liu and Brasier2015, Reference Menon, McIlroy and Brasier2016); or, as commonly noticed in Ediacaran marine strata (d) as structures of interaction between soft-bodied organisms and biomats (e.g. holdfast casts and ‘mop structures’) (Gehling et al. Reference Gehling, Narbonne and Anderson2000; MacGabhann, Reference MacGabhann2007; Tarhan et al. Reference Tarhan, Droser and Gehling2010, Reference Tarhan, Droser and Gehling2015). Caution is therefore necessary when interpreting most of them as of biological origin and/or as fossils of multicellular organisms. Indeed, recent studies have highlighted the importance of detailed, multiproxy investigations to assess problematic sedimentary structures, such as Ediacaran discoidal forms (Menon et al. Reference Menon, McIlroy, Liu and Brasier2015, Reference Menon, McIlroy and Brasier2016; Inglez et al. Reference Inglez, Warren, Okubo, Simões, Quaglio, Arrouy and Netto2019; Schwid et al. Reference Schwid, Xiao, Nolan and An2021).
Within this context, the upper Ediacaran Cerro Negro Formation (Tandilia System, NE Argentina) contains numerous discoidal structures preserved in association with sub- to intertidal massive and thinly laminated sandstone beds commonly rich in MISS (Arrouy et al. Reference Arrouy, Warren, Quaglio, Poiré, Simões, Rosa and Gómez-Peral2016, Reference Arrouy, Gaucher, Poiré, Xiao, Gómez-Peral, Warren, Bykova and Quaglio2019). Their abundance and dense distribution on bedding planes, and their intricate radial morphology, led to their previous interpretation as possible moulds of discoidal holdfasts, with an affinity to Aspidella (Arrouy et al. Reference Arrouy, Warren, Quaglio, Poiré, Simões, Rosa and Gómez-Peral2016).
Given the implications in understanding the preservation and significance of metazoan–biomat interactions in this crucial period of life evolution, we performed a detailed re-analysis of some of those structures from the Cerro Negro Formation based on their: (1) sedimentary facies association; (2) size distribution; (3) morphology and internal textures; and (4) composition. We therefore propose alternative hypotheses for the origin of those disc-like structures in this contribution, discussing their possible biological origin and significance for sedimentary dynamics in biomat-stabilized substrates.
2. Stratigraphy, age constraints and palaeobiological record of the Ediacaran Tandilia System
The Tandilia System is a narrow outcrop belt of c. 60 km in width and 350 km in length, located in the SE region of the Buenos Aires Province, Argentina (Dalla Salda et al. Reference Dalla Salda, Bossi and Cingolani1988; Cingolani, Reference Cingolani2011). The unit exposes igneous and metamorphic rocks from the Palaeoproterozoic basement of the Río de La Plata Craton, which is discordantly covered by Neoproterozoic and lower Palaeozoic sedimentary deposits (Fig. 1a, b) (Cingolani, Reference Cingolani2011; Rapela et al. Reference Rapela, Fanning, Casquet, Pankhurst, Spalletti, Poiré and Baldo2011). The Neoproterozoic sedimentary sequence is divided into two main successions, separated by a regional unconformity. The lower and upper successions encompass the Sierras Bayas Group (Villa Monica, Cerro Largo, Olavarría and Loma Negra formations) and La Providencia Group (Avellaneda, Alicia and Cerro Negro formations), respectively (Iñiguez, Reference Iñiguez and Caminos1999; Poiré & Spalletti, Reference Poiré, Spalletti, De Barrio, Etcheverry, Llambías and Caballé2005; Gómez-Peral et al. Reference Gómez-Peral, Poiré, Strauss and Zimmermann2007; Arrouy et al. Reference Arrouy, Poiré, Gómez-Peral and Canalicchio2015, Reference Arrouy, Warren, Quaglio, Poiré, Simões, Rosa and Gómez-Peral2016, Reference Arrouy, Gaucher, Poiré, Xiao, Gómez-Peral, Warren, Bykova and Quaglio2019) (Fig. 1c).
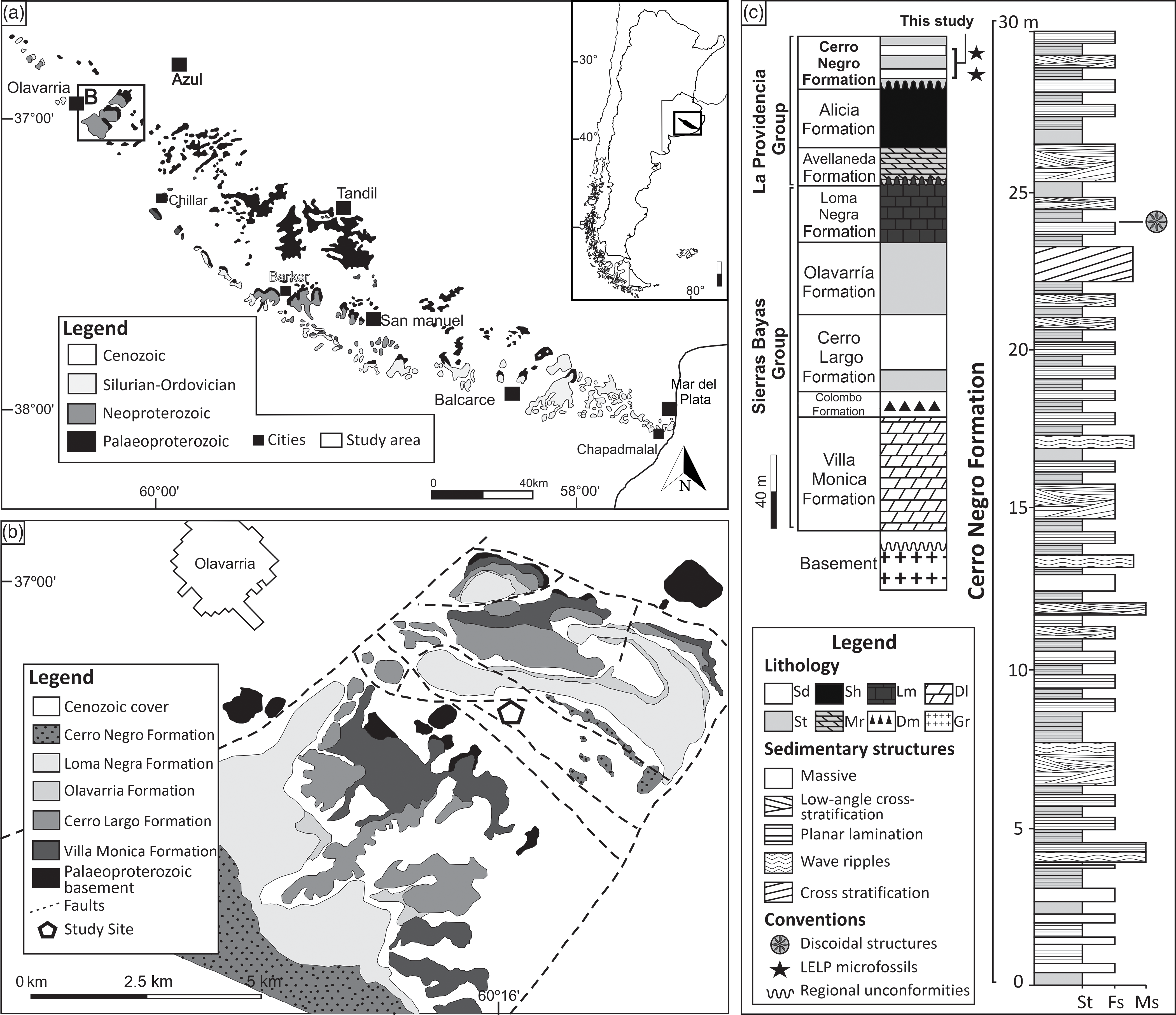
Fig. 1. Geologic and stratigraphic context of the study area. (a) Simplified geological map of the Tandilia System in the southern part of Buenos Aires Province, Argentina. (b) Geological map of the studied site. White pentagon marks the location of the La Cabañita quarry, where the rocks of the Cerro Negro Formation are exposed. (c) Lithostratigraphic column of the Neoproterozoic succession of the Tandilia System. Late Ediacaran Leiosphaeridia Palynoflora (LELP) is indicated. On the right, columnar section of the studied interval from the middle part of the Cerro Negro Formation. St – siltstone; Fs – fine sandstone; Ms – medium sandstone; Sd – sandstone; Sh – shale; Mr – marl; Lm – limestone; Dm – diamictite; Dl – dolostone; Gr – granitoid.
The Loma Negra Formation is the uppermost unit of the Sierras Bayas Group and comprises a c. 40-m-thick carbonate-dominated succession, with greenish laminated mudstones (c. 8 m), overlain by black thinly laminated mudstones in its upper part (Gómez-Peral et al. Reference Gómez-Peral, Sial, Arrouy, Richiano, Ferreira, Kaufman and Poiré2017, Reference Gómez-Peral, Kaufman, Arrouy, Richiano, Sial, Poiré and Ferreira2018, Reference Gómez-Peral, Arrouy, Poiré and Cavarozzi2019). Its age was inferred as late Ediacaran, and available 87Sr/86Sr ratios and δ13C data suggest deposition between 590 and 580 Ma (Gomez Peral et al. Reference Gómez-Peral, Poiré, Strauss and Zimmermann2007, Reference Gómez-Peral, Arrouy, Poiré and Cavarozzi2019). The putative presence of cloudinomorphs in this unit (Gaucher et al. Reference Gaucher, Poiré, Gómez-Peral and Chiglino2005) could safely indicate an age of between 550 and 538 Ma (Grotzinger et al. Reference Grotzinger, Bowring, Saylor and Kaufman1995; Amthor et al. Reference Amthor, Grotzinger, Schroder, Bowring, Ramezani, Martin and Matter2003; Linnemann et al. Reference Linnemann, Ovtcharova, Schaltegger, Gärtner, Hautmann, Geyer, Rich, Plessen, Hofmann, Zieger, Krause, Kriesfeld and Smith2018), but the occurrence of these index fossils needs to be confirmed.
The Cerro Negro Formation occurs most commonly as subsurface deposits but is fairly exposed at the exploitation fronts of the La Cabañita quarry. In this locality, the unit is represented by a c. 30-m-thick succession overlying an erosional karstic surface that separates it from the underlying units (Barker Surface from Poiré et al. Reference Poiré, Gaucher and Germs2007) (Fig. 1c). The Cerro Negro Formation is separated from the underlying Alicia Formation by another less pronounced unconformable surface (Arrouy et al. Reference Arrouy, Poiré, Gómez-Peral and Canalicchio2015). Terrigenous deposits characterize the unit, including massive micaceous sandstone and siltstone, fine sandstone with swaley cross-stratification, wave-rippled sandstone, trough cross-bedded sandstone and heterolithic facies (Arrouy et al. Reference Arrouy, Poiré, Gómez-Peral and Canalicchio2015). This facies association suggests deposition by currents and waves, alternated with times of low energy coupled with fine-grained sediment (silt and mud) settling under shallow-water conditions (Arrouy et al. Reference Arrouy, Poiré, Gómez-Peral and Canalicchio2015, Reference Arrouy, Warren, Quaglio, Poiré, Simões, Rosa and Gómez-Peral2016). MISS are common, preferably occurring associated with fine- and very-fine-grained sandstone facies with planar lamination. Such features include the ‘Kinneyia’, ‘Elephant Skin’ and possible ‘Arumberia’-type structures (Arrouy et al. Reference Arrouy, Warren, Quaglio, Poiré, Simões, Rosa and Gómez-Peral2016).
Organic walled microfossils have been described in both the Alicia and Cerro Negro formations (Gaucher et al. Reference Gaucher, Poiré, Gómez-Peral and Chiglino2005; Arrouy et al. Reference Arrouy, Gaucher, Poiré, Xiao, Gómez-Peral, Warren, Bykova and Quaglio2019). In the latter, these include Leiosphaeridia tenuissima, L. jacutica and Synsphaeridium sp. at the intermediate part of the succession and Siphonophycus solidum at the base, suggesting a late Ediacaran Leiosphaeridia Palynoflora (LELP) (Gaucher et al. Reference Gaucher, Poiré, Gómez-Peral and Chiglino2005; Grey, Reference Grey2005; Xiao & Laflamme, Reference Xiao and Laflamme2009; Arrouy et al. Reference Arrouy, Gaucher, Poiré, Xiao, Gómez-Peral, Warren, Bykova and Quaglio2019). The Cerro Negro Formation is discordantly succeeded by marine sandstones of the Balcarce Formation, in which U–Pb detrital zircon ages indicate a maximum depositional age of 480 Ma (Rapela et al. Reference Rapela, Pankhurst, Casquet, Fanning, Baldo, González-Casado, Galindo and Dahlquist2007). This unit is also rich in Palaeozoic marine trace fossils, such as arthropod furrows, trackways and scratch marks such as Cruziana furcifera, Diplichnites isp. and Monomorphichnus isp. (Poiré et al. Reference Poiré, Spaletti and Del Valle2003).
3. Sampling and analytical methods
To understand the spatial distribution and size frequency variation of the discoidal structures, 477 discs preserved in positive epirelief structures were measured and quantified in the field. Discs were collected at the La Cabañita mining pit, being mostly retrieved from float blocks. Based on field observations, they are interpreted as coming from one single bed. Specimens with their fragmented smooth domed-shaped tops allowed us to access the internal structures of the discs. Samples were cleaned, prepared and imaged using a digital camera (Nikon D3100) coupled to a fixed camera tower (Kaiser RTX). In order to assess the three-dimensional (3D) internal features, 17 specimens were selected for sectioning at intervals of 5–10 mm using a conventional electrical rock saw with diamond-cutting blades. The resulting slabs were then meticulously polished at intervals of 1–2 mm using a carborundum powder-based manual grinder, progressively revealing the internal structures of the discs. Diametrical and peripherical sections contain the central vertical tube and the radial ridges, respectively. Polished slabs were scanned in 3200 dpi resolution using a digital scanner (Epson Perfection V700 PHOTO). The petrographic analysis included transmitted and reflected microscopy (Zeiss Axioscope 40) with an attached digital camera (Canon EOS 5D Mark II). The chemical and mineralogical composition of the sedimentary grains and cement were identified in polished thin-sections coated with carbon powder (JEOL SSM 6010LA) scanning electron microscope (SEM), equipped with energy-dispersive spectroscopy (EDS) calibrated for 15 kV and 15 nA. For a complementary 3D reconstruction of the structures, a selected sample was scanned using a Skycan 1172 X-ray microtomographer at the Paraná Federal University, Laboratório de Análise de Minerais e Rochas (LAMIR). Scanning was calibrated with a source voltage of 80 kV and a current of 124 μA. The 3D reconstruction was performed using CT-analyser software of Bruker Corporation, which allowed us to highlight slight density contrasts between distinct minerals present at different lamina within the analysed samples (see online Supplementary Material S1, available at http://journals.cambridge.org/geo). The statistical description was carried out using the software OriginPro8, and the image treatment was performed using the software CorelDRAW. Analysed specimens are housed at the Department of Geology, São Paulo State University, Rio Claro campus, São Paulo, Brazil and identified by their respective numbers in the figure captions.
4. Results
4.a. Sedimentary aspects from the Cerro Negro Formation and associated discoidal structures
Deposits of the lowermost and intermediate part of the Cerro Negro Formation represent a sand-dominated shallow-marine platform (Arrouy et al. Reference Arrouy, Poiré, Gómez-Peral and Canalicchio2015, Reference Arrouy, Gaucher, Poiré, Xiao, Gómez-Peral, Warren, Bykova and Quaglio2019), mainly formed by decimetre-thick tabular beds of cross-bedded, ripple cross-laminated and planar-laminated sandstone (Fig. 2a). These facies are occasionally rich in mudstone intraclasts (Fig. 2b) and are interbedded with centimetre- to decimetre-thick beds of massive to finely laminated siltstone and mudstone beds. This facies association is related to shallow waters in intertidal to subtidal settings (Arrouy et al. Reference Arrouy, Poiré, Gómez-Peral and Canalicchio2015). Planar-laminated tabular beds of sandstone are particularly rich in surface sedimentary textures (SST, sensu Davies et al. Reference Davies, Liu, Gibling and Miller2016) that strongly resemble MISS, including ‘Kinneyia’ and ‘Old Elephant Skin’ textures (Arrouy et al. Reference Arrouy, Warren, Quaglio, Poiré, Simões, Rosa and Gómez-Peral2016), and possibly ‘Arumberia’, which are suggestive of deposition in very shallow-water conditions (Kolesnikov et al. Reference Kolesnikov, Grazhdankin and Maslov2012, Reference Kolesnikov, Danelian, Gommeaux, Maslov and Grazhdankin2017) (Fig. 2c, d). Although further investigation is important to interpret the biogenicity of all these features, petrographic observations of some of them suggest at least a minor microbial role in their development. In multiple stratigraphic horizons several discoidal, positive-epirelief structures are observed, but one single bed is marked by a dense concentration of these features (Figs 3c, 4a, 5a, b), most often possessing a smooth upper surface, a discrete depression in their central region and occasional internal radial grooves and ridges, as seen in fragmented specimens (Fig. 4a). This occurrence (which is comparable to that presented by Arrouy et al. Reference Arrouy, Warren, Quaglio, Poiré, Simões, Rosa and Gómez-Peral2016) is represented by a fine- to very-fine-grained planar-laminated sandstone bed (c. 50 cm thick) with occasional mudstone intraclasts, here informally referred to as Disc Bed 1 (DB-1).
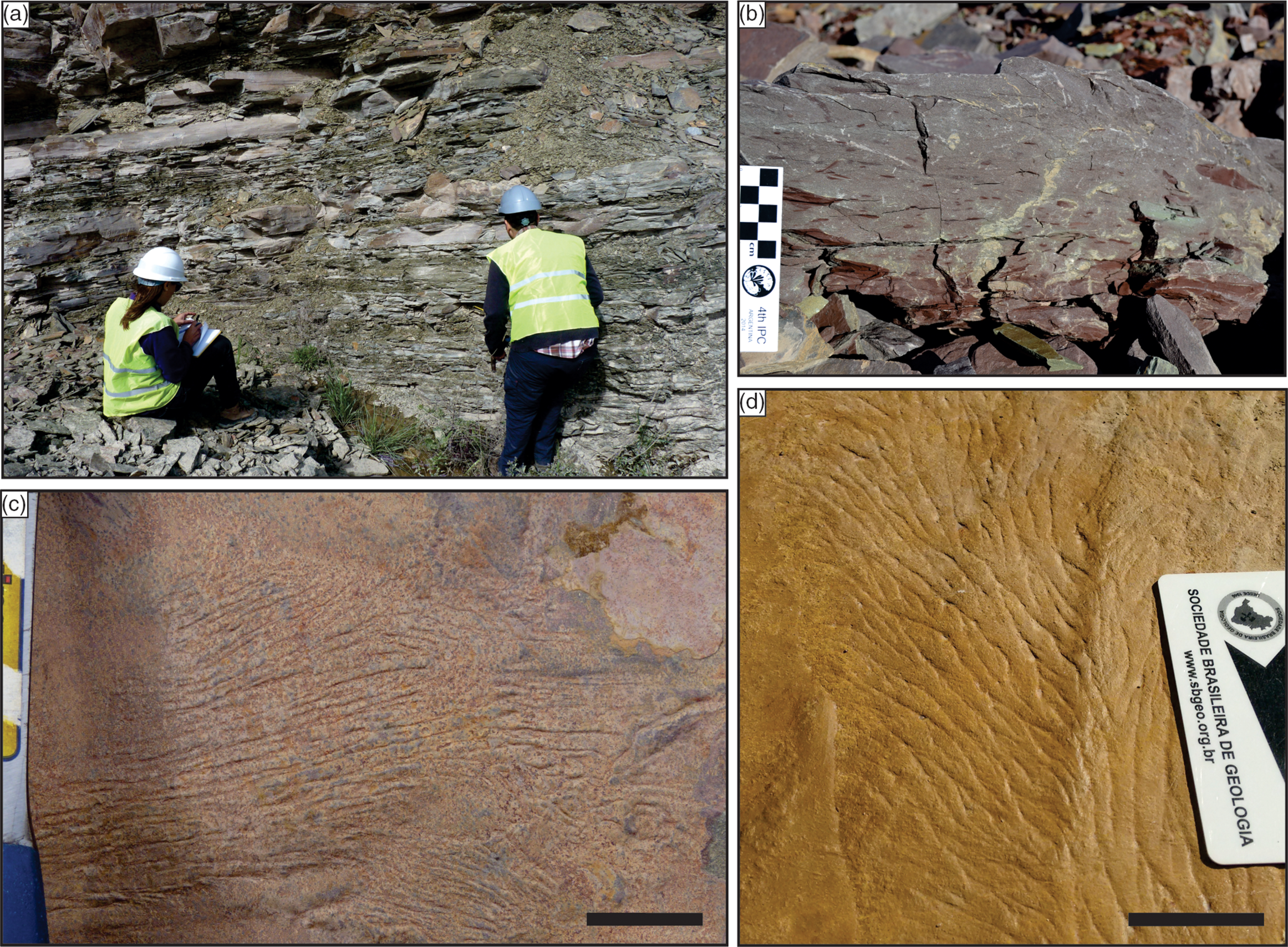
Fig. 2. Sedimentary facies and microbially induced sedimentary structures (MISS) from the Cerro Negro Formation. (a) Fine sandstone-dominated succession with subordinate decimetre-scale intercalations of siltstone and claystone. (b) Massive fine sandstone facies rich in reddish claystone intraclasts. (c, d) Well-preserved wrinkled to filamentous texture typical of arumberiamorph structures, in fine sandstone facies. (a) Person on right is 1.85 m tall; scale bars: (c) 2 cm and (d) 4 cm.
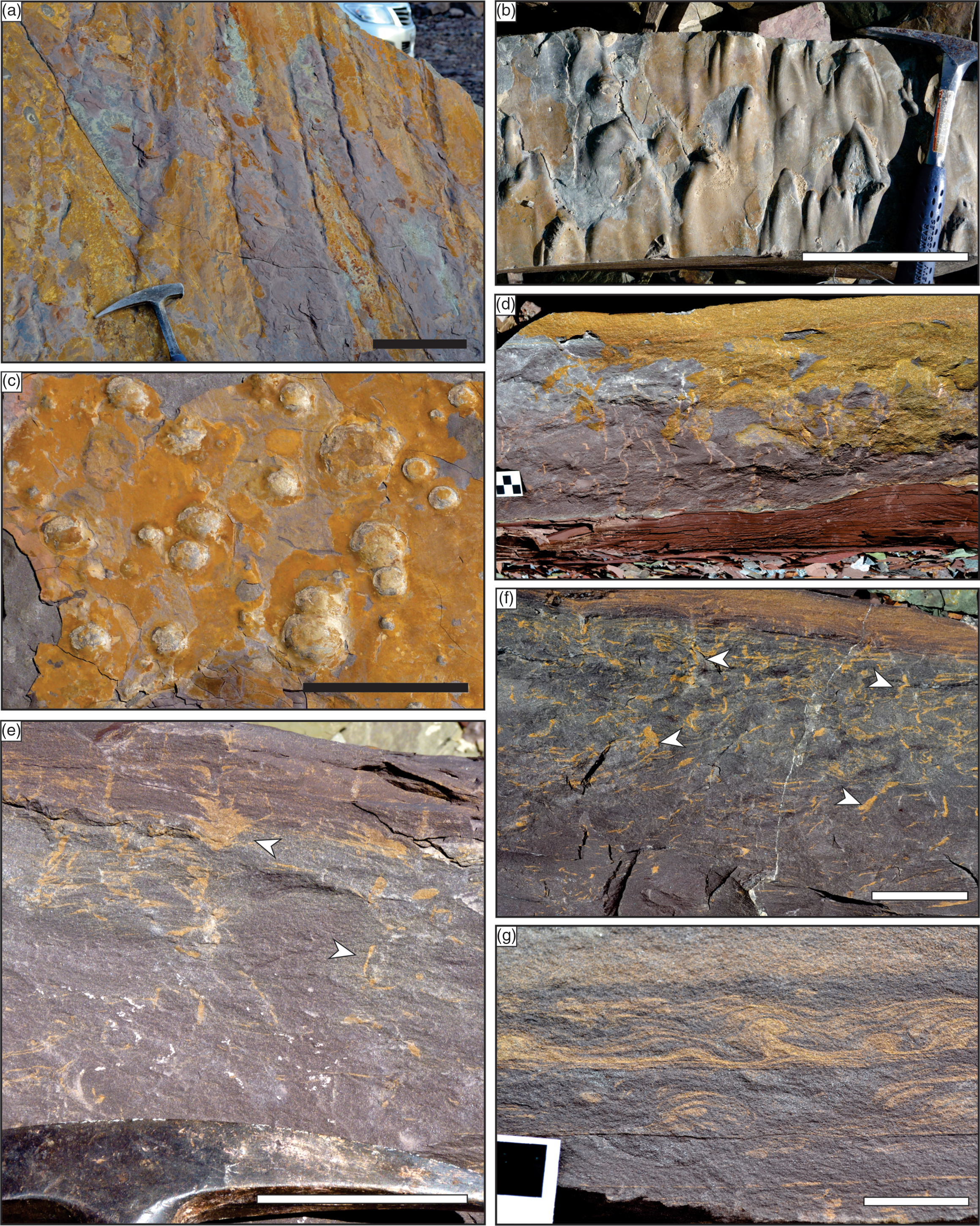
Fig. 3. Sedimentary structures from the fine-grained sandstone disc-bearing bed (DB-1). (a, b) Sandstone blocks with bottom surfaces rich in scour and flute marks, indicative of erosion under high-energy events. (c) Discoidal structures in positive epirelief in the top surface of a fine-grained sandstone bed. (d) Erosive contact between fine-grained sandstone bed and reddish claystone. Note several irregular disruptive sub-vertical structures in the lower bed. (e, f) Cross-section detail view of disrupted lamination and numerous irregular and sub-vertical structures (white arrows). (g) Convolute lamination probably associated with synsedimentary deformation. Scale bars: (a–c) 15 cm; (e, f) 5 cm; and (g) 2 cm.
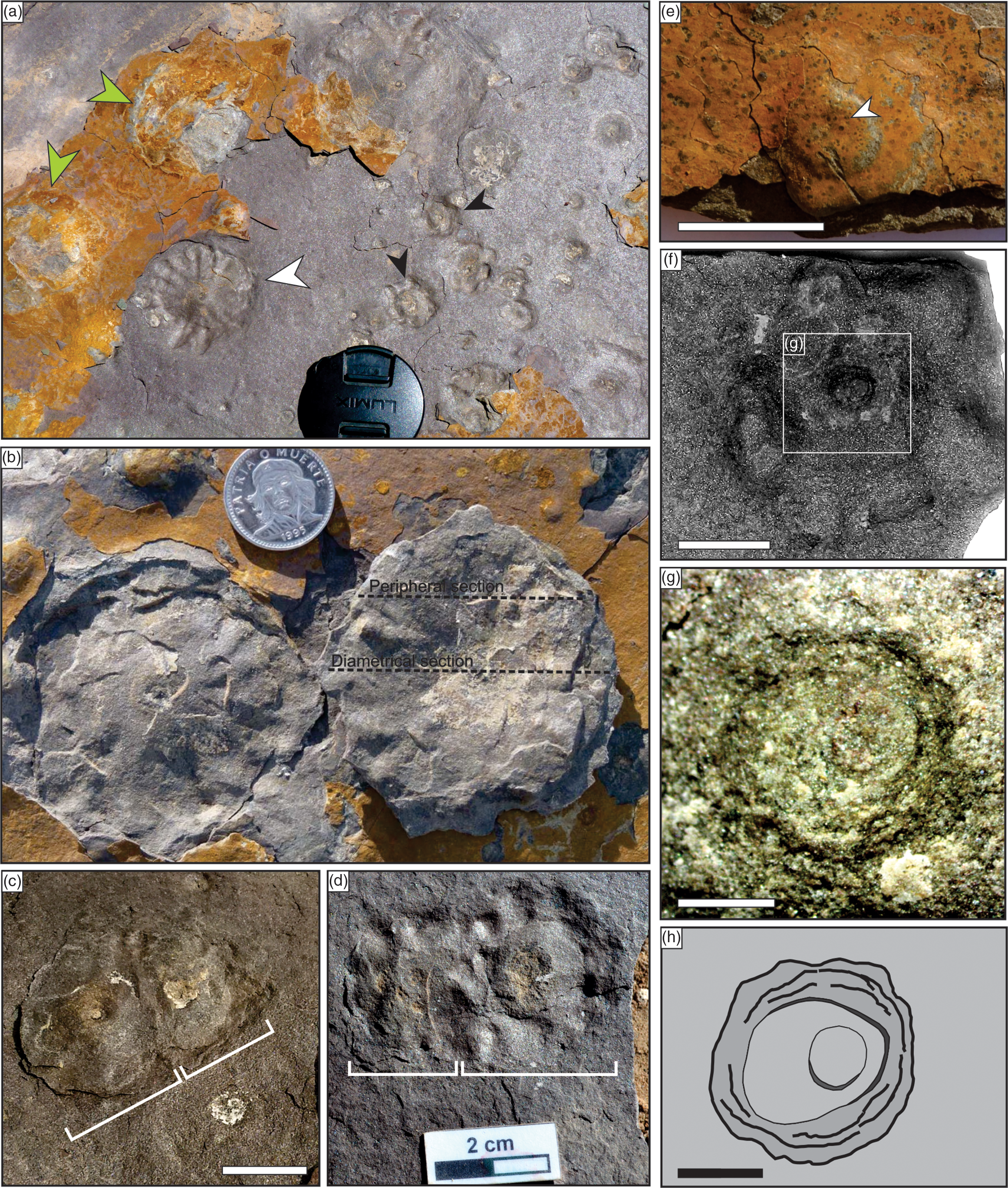
Fig. 4. The morphological diversity of the discoidal structures of the Cerro Negro Formation. (a) Top surface showing positive (green arrow) and negative relief (white and black arrows), with exposed subsurface radial ridges (white arrow) and poorly preserved lobes (black arrows). (b) Detached positive-relief dome of a specimen with well-preserved radial morphology. Dotted lines indicating diametrical (below) and peripheral (above) sections made in similar structures (as seen in Figs 7b, f, 8a, respectively). (c, d) Mutually deformed adjacent discoidal structures, in (c) positive epirelief and (d) negative epirelief (LC-10). (e, f) Lobed disc (LC-26) in (e) positive epirelief and (f) counterpart view in hyporelief of its detached fragment. Note slight central depression in (e) (white arrow). (g, h) Detail of (f), showing (g) the small central concentric structure, marked by dark, fine-grained films and (h) a sketch of the same structure. The diameter of the camera lens cap in (a) and the coin in (b) is 5 cm and 2.3 cm, respectively. Scale bars: (c–f) 1 cm and (g, h) 2 mm.
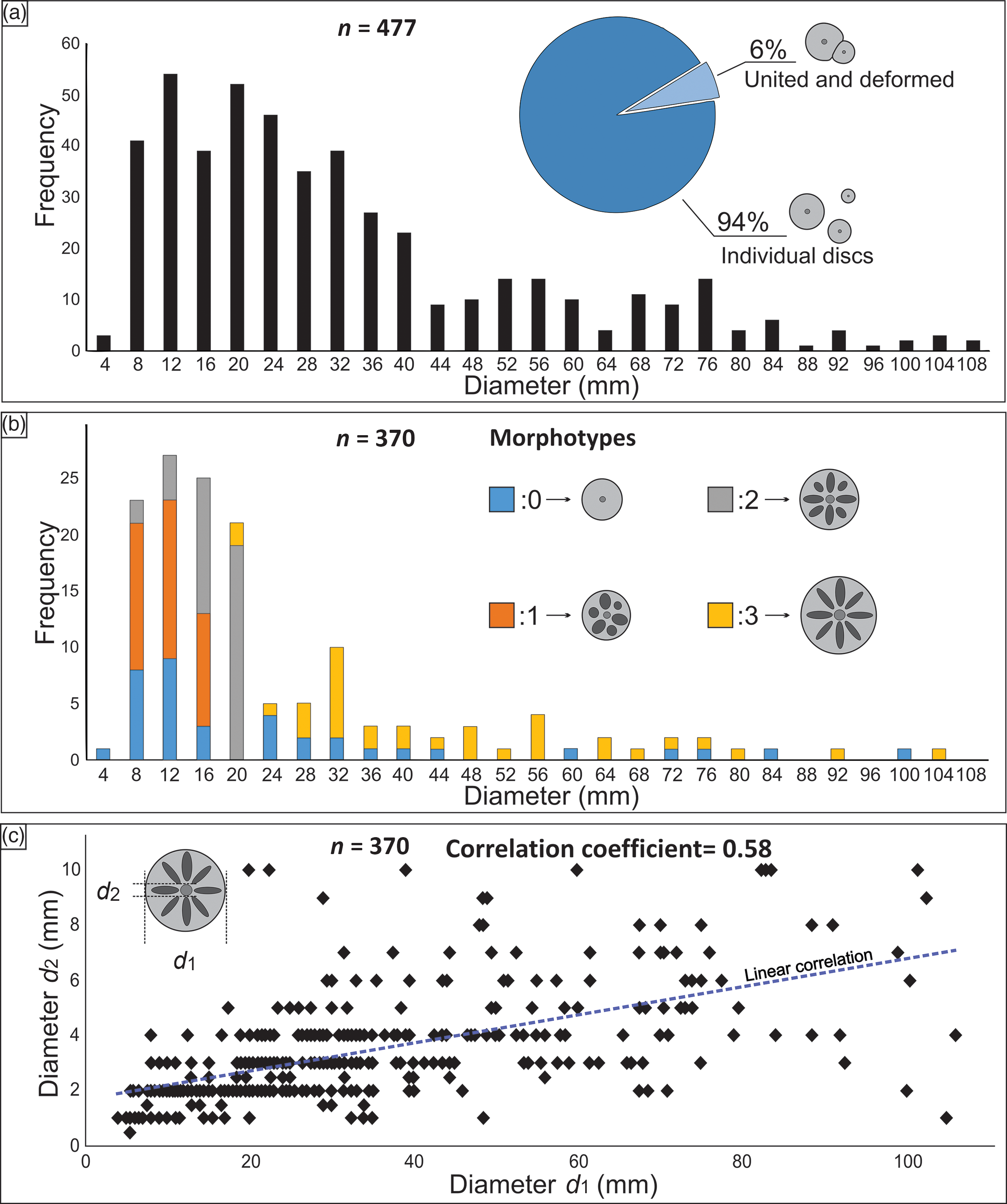
Fig. 5. Size distributions of discoidal structures with distinct morphology. (a) Size class distribution of discs showing the proportion of individualized (94%, n = 448) and united and mutually deformed specimens (6%, n = 29). (b) Distribution of different morphotypes within distinct size classes. Note that morphotypes 1 and 2 tend to be concentrated on the smaller specimens, while morphotype 3 is normally associated with the larger specimens. (c) Correlation between total diameter (d 1) and the diameter of central vertical structure (d 2).
The presence of irregular gutter casts and flute marks at the base of this bed indicates the erosion of muddy cohesive substrates by strong unidirectional bottom currents during episodic high-energy events (e.g. floods or debris flows) (Fig. 3a, b). Soft-sediment deformation structures occur throughout DB-1, such as convoluted lamination, as well as a series of sub-vertical irregular features that intersect sedimentary strata (Fig. 3d, g). In many cases, these deformational features obliterated the original sedimentary structures, providing a characteristic massive appearance to the bed. Locally, these sedimentary structures are observed to vary from base to top of the bed, with synsedimentary folds at the base (Fig. 3g), succeeded by disrupted lamination and massive textured sediments to the top, culminating in a centimetre-thick interval with several vertical irregular structures similar to small-scale clastic dykes (or pipes) (Figs 3d–f, 6d). In some examples, these cross-cutting structures end at the surface of the bed as a positive discoidal mound (Figs 4c, 6a–c) although, given their irregular geometries, it is difficult in most cases to trace the continuity of these vertical features for more than a few centimetres up or down. Discs at the top of DB-1 are dome-shaped and covered by a smooth finely grained oxidized material (Figs 3c, 4a, e, 5a). The upper surfaces of the discs are smooth, except for a discrete central depression and faint lobes visible in some specimens (Fig. 4e). When these positive-epirelief discs are detached from the bedding surface (Fig. 2a, b), they commonly reveal a well-marked pattern of ridges and grooves radiating from their central region (Fig. 4a–d). These features are absent in some specimens, or seem to be irregularly and poorly developed (or preserved) in others that, instead of radial ridges, show a series of lobular protrusions distributed along the area of the discs (Fig. 4f).
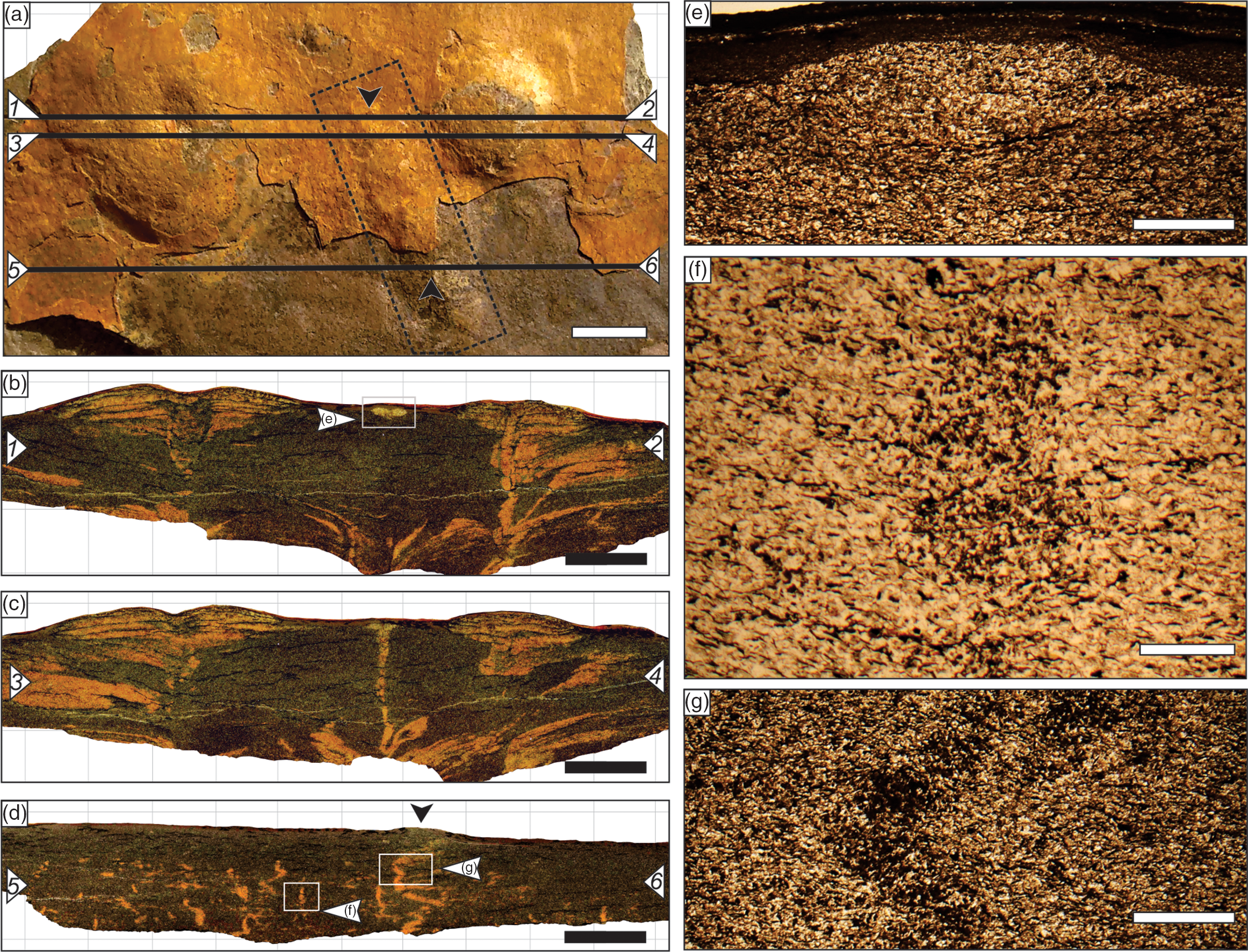
Fig. 6. Polished slab from the upper part of the sandstone bed containing small discs (LC-24). (a) Bedding surface with indicated positions of sections near the diametrical axes of two discs (sections 1–2 and 3–4), and section outside the disc perimeter (section 5–6). The dotted polygon indicates a linear elevation (black arrows) cut by sections 1–2, 3–4 and 5–6. (b, c) Sections near the diametrical axes of the discs, revealing a central funnel-shaped vertical structure and an upper laminated region. Note the vertical feature in the central part of the image, which culminates in a small lenticular structure (depicted in (e)), corresponding to the nearly linear elevation in (a). (d) Polished section showing several irregular sub-vertical structures detailed in petrographic views in (f) and (g). (e–g) Photomicrographs under transmitted light depicting small-scale disruptive structures on the quartz-rich, calcite-cemented very fine sandstone. (f) Detail of small discordant structures in (d) that upwardly deform the lamination of the rock. (g) Detail of a small curved, discordant dyke in (d). Note concentrations of very fine, dark-coloured heavy minerals (mostly iron and titanium oxide, but also biotite grains) within the vertical structures. Scale bars: (a–d) 1 cm; (e, g) 1 mm; and (f) 0.5 mm.
Discs can be broadly divided according to their ornamentation or absence thereof, into four main categories (Fig. 5b) as follows. Type 0 is represented by simple unornamented discs with an occasional discrete central depression (as illustrated in Arrouy et al. Reference Arrouy, Warren, Quaglio, Poiré, Simões, Rosa and Gómez-Peral2016, fig. 2c). Type 1 is characterized by the presence of lobes which vary from 4 relatively large lobes to 14 small protrusions, usually providing the structure with a flower-like morphology (Fig. 4e, f). Type 2 is characterized by discrete radial ridges and grooves, sometimes difficult to identify. Type 3 structures show a well-defined radial pattern, with high-relief ridges varying in number from 5 to 16 (Fig. 4a, b). In the central region of all morphotypes there is a well-pronounced dark-coloured, millimetric (1–10 mm wide), subcircular structure (Fig. 4f, g), sometimes characterized by a group of two to four concentric rings (Fig. 4g, h). These subcircular structures are marked by the alternation of light-coloured fine-grained sandstone and dark-coloured fine-grained material (see petrographic description in Section 4.c below), frequently showing eccentric inner fine-grained sandstone infill (Fig. 4g, h).
When two or more discs occur in adjacent lateral contact, their borders are distinctly deformed in a pattern suggesting plastic rheology (Fig. 4c, d). This pattern was previously noticed by Arrouy et al. (Reference Arrouy, Warren, Quaglio, Poiré, Simões, Rosa and Gómez-Peral2016) and used as a positive argument in favour of the Aspidella holdfast model interpretation. Indeed, it is a common feature associated with other Ediacaran discoidal fossils worldwide (Gehling et al. Reference Gehling, Narbonne and Anderson2000; Tarhan et al. Reference Tarhan, Droser and Gehling2010, Reference Tarhan, Droser and Gehling2015).
4.b. Population density and size distribution of discs within DB-1
In total, we analysed 477 discs that are densely distributed at the top surface of DB-1. Their density on the bedding surface has a mean of 108 individuals per square metre (range, 80–152 m–2), with 94% of the discs occurring as discrete structures and only 6% occurring in adjacent lateral contact with other discs (Fig. 5a). Nevertheless, in some parts of the bed surface, their distribution shows that discs are grouped in clusters of three or more individuals. Most discs show a near-circular geometry, with eccentricity ranging between 0.0 and 0.473 (mean, 0.2). Discs with more ellipsoidal shapes are mostly those with deformed borders as a result of being in contact with other discs. Mean total diameter (d 1) varies between 4 and 108 mm (mean, 31.1 mm; n = 477), with size classes following a clear positively skewed distribution (Fig. 5a).
Analyses of disc morphotypes have revealed an apparent correlation between the mean diameter of the disc and their internal morphological traits identified as morphotypes 0 to 3. The larger discs are less abundant and usually show clear radial markings (morphotype 3; Fig. 5b), whereas the smaller discs are predominant, showing poorly preserved ridges and lobes (morphotypes 0 and 1; Fig. 5b). The diameter of the inner dark-coloured circular structure (d 2) at the central region of discs varies over 1–10 mm (mean, 3 mm; n = 370). There is a low positively skewed correlation coefficient between total diameter (d 1) and inner circular structure diameter (d 2) of 0.58 (Fig. 5c).
4.c. Internal architecture and composition of the discoidal structures
Analysis of diametrical sections performed in 17 specimens revealed that the dome-shaped discs are internally laminated with a cylindrical to a funnel-shaped vertical tube at their central region (Figs 6a–c, 7b, c, e, f). Polished slabs show that these vertical features extend downwards into the massive sediment below (with an observed maximum depth of 5 cm). Locally, the vertical tubes occur in the same level of the irregular sub-vertical structures analogous to small-scale clastic dykes common in the upper part of the bed (Fig. 6b–d). These discordant cross-cutting dykes constitute zones of loose-packed, very-fine-grained sand, cemented by iron oxide with slightly vertically orientated grains (Fig. 6f, g). Towards the top, they are either truncated in specific levels (Fig. 6d), with no visible terminations (Fig. 6f) or culminate in discrete linear protrusions in the bedding planes (suggesting a tabular 3D morphology) (Fig. 6a–d). In some laminated domes, the central vertical tube shows a wider upper part, filled with massive or slightly vertically oriented sand-size grains, indicating a 3D funnel-like morphology (Fig. 7b, c, e). These funnels are in some cases sectioned by an internal rectilinear to a slightly curved tube with a constant diameter (Figs 7b, c, 8b, c). Dark-coloured claystone films, with vertically oriented phyllosilicate grains mantled by iron-rich minerals (Fig. 7c), constitute the margins of both the internal tube and external funnel (Fig. 7f). In a few specimens (n = 3) it was possible to mechanically extract these cylindrical structures from the rest of the host rock, probably because of the contrasting grain size and composition of its margins. When detached from the matrix, these central cylinders have a preferential orientation of placoid grains perpendicular to their axes, which provides their surface with a pattern of discrete transversal striation (Fig. 8a–c).

Fig. 7. Polished slabs of dome-shaped disc structures showing complex internal architecture. (a) Sample LC-27 seen in plan view with indicated diametrical section (grey line). (b) Diametrical polished section 1–2 indicated in (a). (c) Detail and sketch of the central cylindrical structure within a wider funnel highlighted in (b). Note that these structures are somewhat discordant from the lateral lamination. (d) Sample LC-17 in plan view, with indicated diametrical and peripheral sections (grey lines). (e) Diametrical (1–2) and peripheral (3–4) polished sections indicated in (d). (f) Photomicrograph of vertical funnel-shaped structure of specimen illustrated in (e), with its borders comprising fine-grained sediment cemented by oxidized material. Note the subtle convex-down lamination and vertically oriented grains outside the funnel perimeter. Scale bars: (a, b) 2 cm; (c–e) 1 cm; (f) 1 mm. The slight differences in morphology between sample depicted as a scanned rock slab and in the photomicrograph are due to polishing during the manufacture of thin-sections.

Fig. 8. Fragmented specimen LC-34 with its central cylindrical structure three-dimensionally preserved. (a) Plan view photograph of disc with its near central fracture indicated. (b) Internal cylindrical structure showing a gentle upwards-curving morphology. (c) Detail of the structure showing its subtle striated surface (white arrows). Scale bars: (a) 1 cm; (b) 0.5 cm; (c) 2 mm.
Cross-sectioned discs also revealed a well-pronounced internal lamination that follows the curvature of their external positive relief (Fig. 7e). Thin alternations of 0.5–2-mm-thick fine-grained sandstone lenses cemented by calcite, and very-fine-grained sandstone laminae rich in phyllosilicate grains (e.g. muscovite, biotite and chlorite), usually mantled by iron and manganese oxides (Fig. 9a, b), characterizes this lamination. Euhedral pyrite crystals are locally observed through reflected light microscopy, occurring as an interstitial cement between muscovite and biotite crystals. The thin laminae rich in phyllosilicate grains lack carbonate cementation. However, the intergranular regions between angular quartz, unaltered feldspar and phyllosilicate grains are cemented by iron-rich clay minerals (Fig. 9b, d). These lenses are laterally limited to the outermost perimeter of the discoidal domes. At the central part of the discs, these lenses are sharply truncated by a funnel-shaped tube or have a slight downwards deflection of their upper and lower limits (Fig. 7b, c, e, f). At their terminal parts, the lenses show a kind of wedge-shaped morphology and a sharp contact with the fine-grained phyllosilicate-richer host sediment (Fig. 7e). Slabs sectioned across mutually deformed discs (n = 3) show that the internal phyllosilicate-rich films seem to plastically bend over themselves, encapsulating the thin fine-grained sandstone lenses at their terminal parts (Fig. 10a–c). This deformation region is marked by a discrete enlargement in thickness of these lenses, and a slight increase in grain packing (Fig. 10b, c). Further, the analysed clusters (n = 3) in thin-section show that the lenses of at least one of the discs are deformed and shortened in the contact zone when compared with their morphology on the other extremity (Fig. 10a). No evidence of fusion or mixture of sediment between the discs is observed.
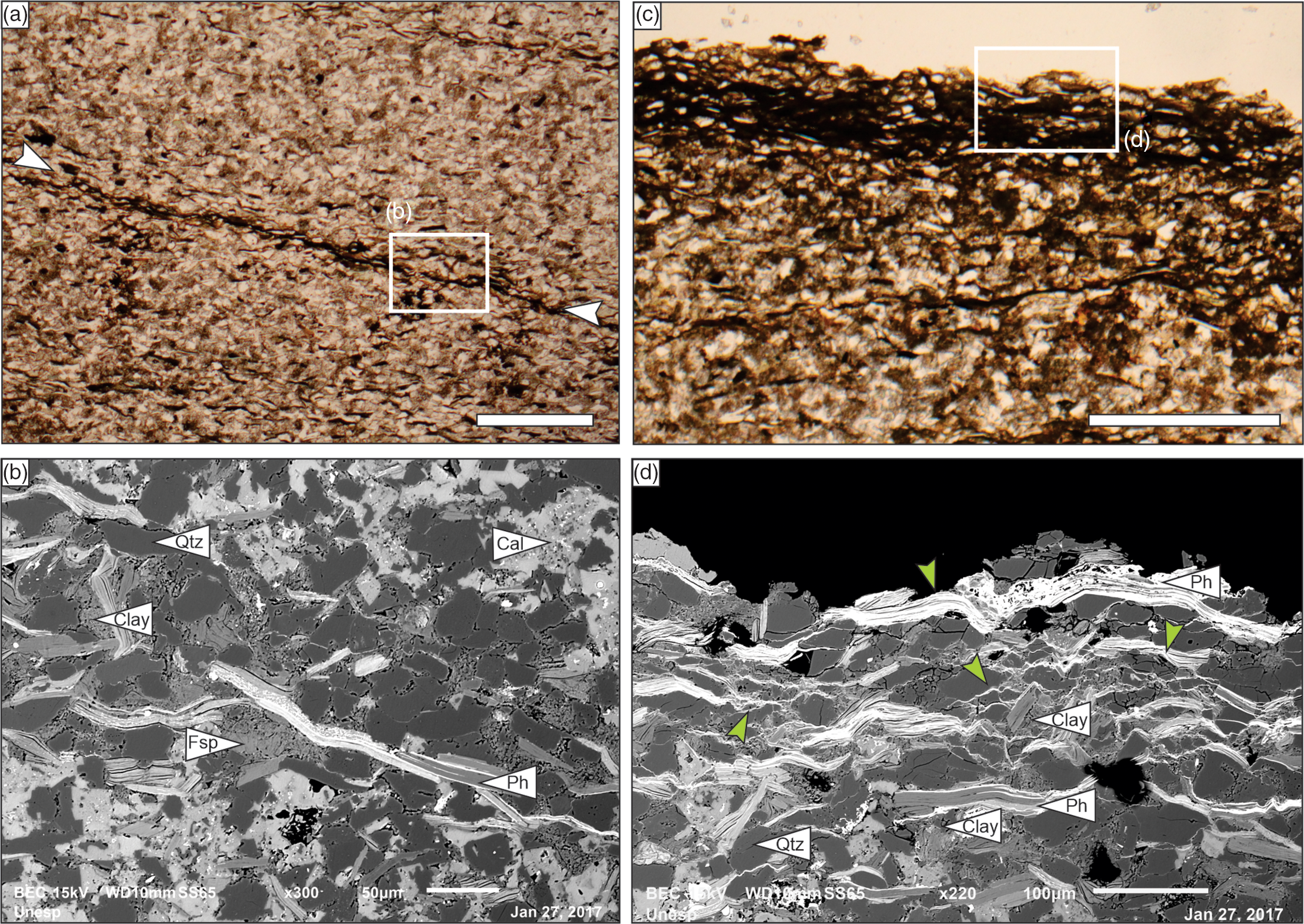
Fig. 9. Microphotographs and SEM images of sample LC-17. (a) Detail under transmitted light microscopy of internal laminated pattern highlighted in Figure 7e. Note the presence of surfaces rich in phyllosilicate plates and dark-coloured clay-rich levels (white arrows). (b) SEM image of small area in (a) (white rectangle) showing details of the clay-rich film. Note the absence of carbonate cement in the middle band, rich in intergranular patches of clay minerals and with horizontal platy grains of phyllosilicate. (c) Close-up under transmitted light of the uppermost surface of disc highlighted in Figure 7e, rich in dark-coloured clay material. Note that some quartz grains seem to float in the dark-coloured matrix, typical of preserved microbial textures. (d) SEM image with details of the upper surface highlighted in (c) (white rectangle). White-coloured regions (green arrows) indicate zones rich in high-density elements (mostly iron). Note how these regions develop crinkled intergranular surfaces. Scale bars: (a, c) 0.25 mm; (b) 50 μm; (d) 100 μm. Clay – clay mineral; Qtz – quartz grains; Cal – calcitic cement; Fsp – feldspar grains; Ph – phyllosilicate grains.

Fig. 10. Internal texture and structures observed in diametrical sections of mutually deformed discs and peripheral sections of isolated discs. (a) Diametrical section of sample LC-04, with two mutually deformed discs. Note the shortening of internal lobes and laminae in the specimen on the left. (b) Thin-section details of the contact area between the two structures seen in (a). (c) Same image in black and white, highlighting the geometrical arrangement of the thin well-defined sediment lenses (with black-filled contours marking dark-coloured clay-rich silty sediments that surrounds them). (d) Peripheral section of sample LC-15 showing discontinuous lenses of fine-grained light-coloured sandstone below continuous lamination. (e, f) Thin-section detail of (d) showing well-defined, fine-grained sand lenses surrounded by dark, clay-rich siltstone laminae. Scale bars: (a, d) 1 cm; (b, c, e, f) 1 mm. The slight differences in morphology between sample depicted as a scanned rock slab and in the photomicrograph are due to polishing during the manufacture of thin-sections.
When sectioned through an off-centred axis (cutting the peripheral area of the discs and perpendicular to the ridges or lobes, as indicated in Fig. 4b), a change in the lenticular geometry of the internal framework of the discs is observed (Fig. 10d). In peripheral regions, nearly perpendicular to the ridges or lobes, the thin lenses are restricted to the upper part of the domes, where no ridges are noted. However, in the lower part of the structure, the radial ridges and occasional lobes are laterally restricted lenticular packages of sediment, completely encapsulated by films of phyllosilicate and clay mineral (Fig. 10d–f). This relationship is observed in 3D μCT reconstruction, where phyllosilicate- and heavy-mineral-rich laminae surrounding the lobes and the vertical central structure are highlighted in lighter colours (online Supplementary Videos S1 and S2, and Figure S1 (in Supplementary S1), available at http://journals.cambridge.org/geo).
5. Discussion
We provide various insights into the origin and preservation of Cerro Negro Formation Aspidella-like discoidal forms in the following.
5.a. Hypothesis 1: discs as soft-sediment deformation and fluid migration structures
The presence of planar lamination and erosional and load structures at the base of DB-1 suggests rapid deposition of sediments during high-energy events, associated with strong unidirectional currents during flooding events that affected the tidal flat. In this scenario, the presence of convolute folding near the base of this bed that grades to massive facies to the top (Fig. 4d–g) can be interpreted as the direct result of liquefaction processes (Lowe, Reference Lowe1976). During this phenomenon, an external strain upon nonconsolidated sediments leads to an increase in pore-water pressure, temporarily disrupting the textural integrity of the grains, and annulling the effects of shear stress (Lowe, Reference Lowe1975; Allen, Reference Allen1982). This effect causes the sediment to behave like a high-viscosity liquid, allowing it to accommodate strain and to deform plastically (Lowe, Reference Lowe1975). Fluidization often accompanies liquefaction and is associated with the loss of grain support due to upwards migration and expulsion of interstitial fluid (either water or gas) (Lowe, Reference Lowe1976; Allen, Reference Allen1986; Owen et al. Reference Owen, Moretti and Alfaro2011). This process might be responsible for the development of sediment intrusions, such as clastic dykes and pipes (Lowe, Reference Lowe1976), and sediment extrusion, such as sand volcanoes (Lowe, Reference Lowe1975; Gill & Kuenen, Reference Gill and Kuenen1957). In nature, these extrusive structures may vary in size and abundance according to the intensity of the triggering mechanism, the larger ones (decimetre to metre size) being reported as the result of high-energy seismic events (Van Loon & Maulik, Reference Van Loon and Maulik2011; Reid et al. Reference Reid, Thompson, Irvine and Laird2012; Rodríguez-Pascua et al. Reference Rodríguez-Pascua, Silva, Pérez-López, Giner-Robles, Martín-González and Del Moral2015). Small extrusive structures (millimetre- to centimetre-scale) are expected to be less frequent in the sedimentary record as a result of their intrinsic low preservation potential. These are therefore uniquely preserved when rapid burial by non-destructive processes or early cementation, or entombment by microbial communities, occurs (Põldsaar & Ainsaar, Reference Põldsaar and Ainsaar2014). Sediment liquefaction or fluidization may be triggered by diverse processes, such as tectonic seismicity (Allen, Reference Allen1986), tsunami wave impact (Matsumoto et al. Reference Matsumoto, Naruse, Shiehiro, Surphawajruksakul, Jarupongsakul, Sakakura and Murayama2008) or wave shocks produced by meteorite impacts (Alvarez et al. Reference Alvarez, Staley, O’Connor and Chan1998). However, usual processes related to the day-by-day dynamics of the basin are also suggested as potential triggers of liquefaction, and include the impact of storm waves (Alfaro et al. Reference Alfaro, Delgado, Estévez, Molina, Moretti and Soria2002), strain related to high-amplitude tidal oscillation (Greb & Archer, Reference Greb and Archer2007) or rapid overload of sediment (Moretti et al. Reference Moretti, Soria, Alfaro and Walsh2001).
The general morphology and architecture of the discoidal structures of the Cerro Negro Formation share many characters with sand volcanoes, including their domed shape and the central cylindrical disruptive structures (Van Loon & Maulik, Reference Van Loon and Maulik2011; Reid et al. Reference Reid, Thompson, Irvine and Laird2012; Põldsaar & Ainsaar, Reference Põldsaar and Ainsaar2014). We therefore tentatively interpret these vertical structures (Fig. 3d–g) as small conduits of upwards fluid migration. Further, some internal features of the discs suggest active injection and emplacement of sediment: (1) massive infill of central shaft; (2) vertical orientation of phyllosilicate grains, parallel to the margins of the central conduit; (3) contrasting grain size and mineralogy between internal components of the discs (upper lamina, lower lobes and central shaft/conduit) and the host sediment; and (4) increase in grain packing close to the terminal part of the lobes. On the other hand, the presence of multiple stacked laminae and lobes of sediment patches, associated with cross-cutting relationships between the central tubular structure and the outer funnel (Fig. 7b, c, e), strongly indicates successive pulses and/or events of sediment and/or fluid emplacement, a peculiarity hard to explain under a simplistic model of sedimentary liquidization. Given the poor outcrop exposition of the particular interval studied, inferences regarding the possible triggers for these events are still missing. However, the regularity of these sand injections and their small scale allow us to assume the phenomena responsible for their development might have involved cyclic events, intrinsic to a tidally influenced depositional system. Indeed, field observations in modern sedimentary environments, as well as flume experiments, have demonstrated the effects of wave action in the liquefaction and re-suspension of cohesive sediments due to pore-water pressure increase (Foda & Tzang, Reference Foda and Tzang1994; Xu et al. Reference Xu, Sun, Yu, Lin, Hu, Zhao and Guo2012; Jia et al. Reference Jia, Zhang, Zheng, Liu, Jeng and Shan2014). Experimental studies show a positive correlation between the cohesiveness of the material and the development of liquidization conditions, whereas evidence of this phenomenon in DB-1 reinforces the importance of sediment biostabilization in its response to physical sedimentary processes.
5.b. Hypothesis 2: discs as evidence of animal–sediment interactions
Textural and architectural features of the discs in this study indicate their development involved a syndepositional disturbance (or synsedimentary deformational process), rather than a passive moulding or casting of a putative holdfast from a soft-bodied organism (Gehling et al. Reference Gehling, Narbonne and Anderson2000). Excluding the abiotic factors discussed above, a plausible biological origin would imply an animal–sediment active interaction. Indeed, aside from their synsedimentary character, other observed features can indicate a related biological origin for the discs and associated tubes: (1) the approximately normal distribution of size; (2) the recurrence of architectural patterns, which denotes a certain degree of complexity (as indicated by the correlation between internal laminae, radiated lobes, central tubular structures with an apparent concentric arrangement, and multiple infill events); and (3) the intricate deformational pattern observed between laterally adjacent discs. As bioturbation features (i.e. trace fossils), their general architecture (vertical funnel-shaped tube, radially arranged lobes and laminations), limiting borders (discrete iron-rich claystone lining) and internal grain composition and sorting together imply they should be viewed as ichnotaxobases, which could be used to interpret either function or behaviour (Bromley, Reference Bromley1990, p. 165; Bertling et al. Reference Bertling, Bradd, Bromley, Demathieu, Nielsen, Nielsen, Rindsberg, Genise and Mikula2006).
In this regard, most of the analysed specimens can be described as vertical cylindrical passively filled shafts with funnel-shaped tops and discrete mud-lined walls (Fig. 7b, c, e, f) marked by subtle transversal striation as a result of the preferential orientation of phyllosilicate plates (Fig. 8b, c). In some specimens, the shaft is surrounded by a thick funnel-shaped lining formed by fine sandstone with sub-vertically oriented grains accompanying the funnel-like overall geometry (Fig. 7c). In all analysed specimens, radially distributed, possibly actively filled lobes of very-fine-grained sandstone surround the upper part of the funnel-shaped structures. Lobes grade to irregular wrinkled submillimetre laminae towards the top and determine the flattened dome-like geometry of the structures as observed in the bedding plans. These general constructional patterns, and especially the funnel-like morphology and concentric structure of the shaft walls, somewhat resemble those observed in Rosselia socialis and Cylindrichnus concentricus. These are thought to represent the dwelling or dwelling–feeding burrows of detritus-feeding terebellid polychaete worms reported from Cambrian–Holocene deposits (Nara, Reference Nara1995, Reference Nara2002; Nara & Haga, Reference Nara and Haga2007; Desjardins et al. Reference Desjardins, Mángano, Buatois and Pratt2010; Hofman et al. Reference Hofman, Mángano, Elicki and Shinaq2012; Belaústegui & Gibert, Reference Belaústegui and Gibert2013). In these vertical or inclined (bow-shaped), and sometimes striated, burrows, changes in sedimentary dynamics and lateral and vertical adjustments of their inhabitants result in either funnel or spindle-shaped burrows with thick concentric lined walls (Nara, Reference Nara1995; Belaústegui & Gibert, Reference Belaústegui and Gibert2013). In these structures, funnel-shaped tops are considered to be the result of erosion of the upper part of the burrow, which obliterates its original spindle-shaped morphology (Nara, Reference Nara1995, fig. 8; Nara, Reference Nara1997, fig. 5). However, despite morphological similarities here envisaged, the interpretation of the Cerro Negro Formation structures as Rosselia burrows is not entirely consistent. Differently from Rosselia isp. and similar ichnotaxa (e.g. Cylindrichnus isp.), the upper funnel-shaped part of the shafts from the studied samples is marked by the characteristic radial pattern of soft-sediment displacement and injection. The occurrence of these features in all analysed samples strongly suggests a syngenetic correlation for the development of tubes and radial lobes.
On the other hand, the ichnospecies Monocraterion tentaculatum is a vertical cylindrical shaft with a funnel-shaped aperture connected to a radial horizontal burrow system, which has been interpreted as sporadic horizontal excursions of the vertical burrow dweller (Westergård, Reference Westergård1931; Jensen, Reference Jensen1997). This trace fossil is marked by well-defined horizontal burrows, different from the irregularly shaped lobes or ridges of the Cerro Negro discs. Also, the simple discrete-lined wall of Monocraterion isp. differs from the thick funnel-shaped boundary observed in some specimens in this study (Fig. 7b, c) that resemble the concentric architecture of Rosselia isp. Given all morphological and preservational traits mentioned, it can be hypothesized that some vertical (funnel-shaped tubes) and horizontal (lobes and ridges) structures may result from metazoan activity within matgrounds; if this hypothesis is correct, it would hold important biostratigraphical and evolutionary implications.
During late Ediacaran time (c. 565–538 Ma), vagile bilaterians were getting bigger and more mobile, exploring new substrate tiers (Carbone & Narbonne, Reference Carbone and Narbonne2014). These novelties in body plan and behaviour are expressed by the appearance of branching probing shallow burrows (e.g. treptichnids), large horizontal actively filled burrows (e.g. Torrowangea isp. and Parapsammichnites pretzeliformis), and rudimentary tunnel systems with interconnected vertical and horizontal burrows (Jensen et al. Reference Jensen, Saylor, Gehling and Germs2000; Chen et al. Reference Chen, Zhou, Meyer, Xiang, Schiffbauer, Yuan and Xiao2013; Meyer et al. Reference Meyer, Xiao, Gill, Schiffbauer, Chen, Zhou and Yuan2014; Buatois & Mángano, Reference Buatois, Mángano, Mángano and Buatois2016; Buatois et al. Reference Buatois, Almond, Mángano, Jensen and Germs2018; Xiao et al. Reference Xiao, Chen, Zhou and Yuan2019). True vertical burrows are rare and limited to very shallow tiers, and in many cases can be interpreted as inclined sections or vertical branches of horizontal burrow systems of undermat miners (Buatois & Mángano, Reference Buatois, Mángano, Mángano and Buatois2016). However, shallow Skolithos isp. and Bergaueria isp. have been reported in Ediacaran strata (Netto, Reference Netto2012), as well as equilibrium traces with vertically stacking meniscate patterns associated with Aspidella (Fermeuse Formation, c. 565 Ma) (Menon et al. Reference Menon, McIlroy and Brasier2013). On the other hand, the oldest examples of vertical burrows with concentric and well-developed lateral lining were formerly recorded from Cambrian Stage 2 (Mángano & Buatois, Reference Mángano and Buatois2014), a time interval when shallow-marine environments are commonly marked by ichnofabrics containing abundant Skolithos and Monocraterion, locally Rosselia and also Arenicolites and Diplocraterion (Jensen, Reference Jensen1997; Mángano & Buatois, Reference Mángano and Buatois2014, Reference Mángano and Buatois2020). Meanwhile, as discussed previously in this section, several features within the analysed structures (such as the radial pattern of sediment injection) are difficult to interpret within the expected behavioural spectrum represented by any of these ichnotaxa.
The lack of an authentic concentric lining potentially excludes the Rosselia and Cylindrichnus interpretation; however, Skolithos and Monocraterion ichnotaxobases include vertical orientation, passive infill, a mud-lined wall and a funnel-shaped opening (facultative in Skolithos). The lateral deformation of neighbouring burrows is not recorded for these ichnotaxa, being a feature difficult to explain in this scenario. Regardless, the vertical shafts from Cerro Negro Formation show a consistent diameter throughout the tube, with an organized mud-lined wall and a passive infill, as well as their apparent striated surface, which are good indications of a potential trace fossil nature.
5.c. Scratching the discs: seeking a more parsimonious hypothesis for the origin of the Cerro Negro Formation discoidal structures
The original interpretation of the discoidal structures from the Cerro Negro Formation representing Aspidella taphomorphs was given by Arrouy et al. (Reference Arrouy, Warren, Quaglio, Poiré, Simões, Rosa and Gómez-Peral2016) based on their abundance, normal size-class distribution and external morphology. In this re-evaluation, we provide evidence against this proposition, the most compelling being the presence of a vertical cylindrical downwards extension in the disc central region. The absence of well-preserved fronds (see Arrouy et al. Reference Arrouy, Warren, Quaglio, Poiré, Simões, Rosa and Gómez-Peral2016) and other archetypal Ediacaran organisms also argues against discs representing Aspidella.
At the surface of the disc-bearing bed, the radial lobes and plastic deformation observed in the studied discs suggest an increase in the cohesiveness of grains, possibly due to the presence of non-preserved EPS of an endobenthic microbial community (Gerdes et al. Reference Gerdes, Klenke and Noffke2001; Noffke et al. Reference Noffke, Gerdes, Klenke and Krumbein2001; Davies et al. Reference Davies, Liu, Gibling and Miller2016), although typical wrinkled MISS textures are absent. Furthermore, the presence of convoluted, or truncated/disrupted lamination, and discordant small-scale disruptive vertical structures (Fig. 3d–f) in DB-1 suggests partial liquefaction of this bed soon after deposition. As indicated by hypothesis 1 (Section 5.a), we should consider that the analysed discs may be a product of fluid injection and expulsion due to the localized liquefaction of part of this bed. After the increase in pore-fluid pressure following a triggering mechanism, the sedimentary framework becomes supported by fluid and is injected upwards (Lowe, Reference Lowe1975). The movement of fluid throughout a deposit occurs either uniformly, through seepage, or within well-defined conduits, such as clastic dykes and pipes. Variations in grain size, clay content, the presence of phyllosilicates and organic matter, and the degree of compaction might affect sediment permeability (Lowe, Reference Lowe1975). Any of these parameters may act as barriers for fluid migration, resulting in the development of fluidized pockets of sediment, encased by impermeable materials (Lowe, Reference Lowe1975, Reference Lowe1976).
In the studied case, these processes probably occurred below the seafloor that was sealed by microbial mats, producing a confined sand volcano-like extrusive structure. The presence of sediment stabilizing mats at the surface of the bed is suggested by the concentration of phyllosilicate plates parallel to bedding, as well as the iron-rich clay mineral cement which seems to interweave clastic grains (Fig. 9d). As such, the development of the discoidal mounds can be explained according to the following steps (see Fig. 11). (1) First, the bed is rapidly deposited under an upper-flow regime producing horizontal lamination, followed by covering by a microbial mat. (2) A liquefaction event is then triggered by either normal depositional processes (e.g. stresses associated with storm wave impact or tides) or other less likely external factors (e.g. tectonic seismicity). This process increases the pore-fluid pressure, conditioning the partial fluidization of sediments and upwards migration of the fluid through small-scale vertical pipes (Fig. 11a). The flux of fluidized sediments reaches the seafloor and is confined by the microbial mat at the sediment–water interface (Fig. 11b). At the surface, the observed radial pattern of the sediment injections is probably associated with the interaction of materials with distinct viscosities (fluidized sand and microbially stabilized sediment, Fig. 11c). Analogous structures are observed in tank experiments (L. Menon, unpubl. Ph.D. thesis, Oxford University, 2015) and the small Medusinites-like discs from the Longmyndian Supergroup, reinterpreted as fluid injection structures (Menon et al. Reference Menon, McIlroy, Liu and Brasier2015). (3) The incomplete liquefaction and re-sedimentation with remnant pockets of fluid-supported material then results in the partial collapse of the upper part of the conduit, giving it a funnel-shaped morphology. This step is followed by a period of quiescence, allowing the partial stabilization of the cone-shaped conduit by polymeric substances (which may explain the apparent ‘lined-wall’ in these features) (Fig. 11d). (4) After this, a new stress-induced event occurred (e.g. repetitive action of storm waves), producing new fluidization and fluid migration (injection and possibly ejection) through previously formed conduits (e.g. dykes and other anisotropies) (Fig. 11e). This recurrent process might explain the reactivation and reinjection structures observed in the discs, such as the multiple internal laminations and secondarily sectioned tube conduits (see Fig. 7). Finally, after the last fluid injection, further microbial growth (mat recovery, Fig. 11f) can be inferred given the evidence for microbial films at the top surface of the discs (Fig. 9d). In this scenario, we assume that the distribution of distinct morphotypes among distinct size ranges was affected either by differences in the intensity of fluid injection during distinct events or by the number of times a previously formed conduit was re-activated as a preferential path for fluid migration. As such, small unornamented discs (morphotypes 0 and 1) probably result from single, small-scale pulses of fluid injection, while larger structures with well-developed ornamentations (morphotypes 2 and 3) probably formed in response to more intense pulses. On the other hand, large unornamented structures (also of morphotype 0) might be the result of multiple fluid escapes through already developed paths of fluid migration. In this case, later pulses might have potentially destroyed any morphological pattern previously developed. Indeed, the best-preserved evidence of conduit reactivation is recorded in considerably large structures, such as illustrated in Figure 7b and c. The small size of the resultant internal features indicates that this process of fluid expulsion would have occurred on a proportionally reduced scale, which favours less energetic events such as distal storm waves.

Fig. 11. Genetic model for the formation of the discs in the Cerro Negro Formation deposits. (a) Fine sandy sediments capped by a thin microbial mat during the first event of fluidization. (b) Injection of fluidized material through well-defined conduits underneath the microbially bounded surface. (c) Increase in fluid pressure and lateral displacement of material trapped beneath the microbial mat. (d) Fluid pressure relaxation and partial collapse of the conduit, which develops a funnel-like morphology. (e) Second fluidization event and fluid injection through previously formed preferential pathways and development of laminae under less intense confinement (probably due to partial disruption of the surficial mat). (f) Possible mat recovery and covering of the low-relief sand-volcanoes, enhancing their preservation. Open scale bar based on observations of samples (applicable to all stages): 2 cm.
In this integrative model, some of the complex structures associated with the studied discs can be explained, such as the radial pattern in the domes, its internal lamination and central vertical cone. The lateral deformation of adjacent discs is interpreted as resulting from the plastic deformation of the biostabilized sediment in response to concomitant fluid injection in closely spaced conduits. Similar patterns have been described in the past, developed due to the interaction of gas and cohesive plastic sediments (‘compound gas blisters’ of Cloud, Reference Cloud1960, plate 1A). Microbial growth at the conduit margins could also explain the presence of iron-rich clay mineral films, which may recrystallize to form small phyllosilicate grains during diagenesis. The preferential orientation of their long axes parallel to bedding may be related to compaction, putatively resulting in the apparent tube wall striations, similar to those observed in some terebellid polychaete tubes (Nara, Reference Nara1995; Belaústegui & Gibert, Reference Belaústegui and Gibert2013). The size distribution of discs (d 1), as well as the low correlation between these measurements and the conduit (d 2) diameters, is also conformable with this model. After the first event of liquefaction, the bed and the surficial microbial community probably represented a potentially uniform barrier to fluid ejection. However, a second (less intense) fluidization event would preferentially migrate through already formed conduits, feeding them, cross-cutting enlarged collapsed tops and spreading more material laterally (without necessarily enlarging d 2, considering the correlation coefficient of 0.58).
Thin lamination marked by the alternation of very fine-grained sandstone and phyllosilicate-rich laminae at the upper part of the domes is similar to textures produced by sediment trapping and binding by microbial mats. A comparable condition was described from the Longmyndian Supergroup by Menon et al. (Reference Menon, McIlroy and Brasier2016), in which small sand volcanoes acted as a topographic high favouring the colonization of photosynthetic microbes, resembling a miniaturized version of microbial mounds of carbonate settings. It suggests that these radial patterns might be more common than previously considered, and developed in distinct scales, perhaps due to differences in fluid pressure, grain size and specific traits of the microbial community. The small pimples (Intrites-like structure sensu Menon et al. Reference Menon, McIlroy and Brasier2016) of the Longmyndian Supergroup, and possibly other discoidal dubiofossils, are perhaps within a spectrum of structures developed due to similar processes. This includes descriptions of the pseudofossil Astropolithon, long reinterpreted as a possible sand-volcano (Pickerill & Harris, Reference Pickerill and Harris1979). Their similarities even include the smooth top surface and radial patterns in ‘beheaded’ (or detached/fragmented) positive-relief specimens. Although a microbial influence was not considered at the time, biostabilization might also have played a role in their development. Another example includes the discoidal dubiofossil Mawsonites, also reinterpreted as a potential sand-volcano preserved under the influence of microbial mats, that shows a remarkable pattern of radially distributed lobes (Seilacher et al. Reference Seilacher, Buatois and Mángano2005; but see Van Loon Reference Van Loon2008, for a distinct interpretation).
Finally, fluid injection interacting with a microbially bounded substrate allows us to explain the discoidal structures observed in this study. However, we find their remarkable similarity to vertical lined burrows worthy of note, especially the subtle striated, gently curved tubes observed in some specimens (Fig. 8b, c).
6. Concluding remarks
Previous reports of discoidal structures in the Cerro Negro Formation demonstrated their common occurrence at the basal part of the succession, in some cases showing externally and internally complex morphologies. Intricate internal arrangement in the analysed discs, demonstrated in this study, is difficult to explain if considered under the classic taphonomic model for Aspidella, but shows striking resemblances to Longmyndian discoidal structures (Menon et al. Reference Menon, McIlroy, Liu and Brasier2015, Reference Menon, McIlroy and Brasier2016) and other potential pseudofossils, such as Astropolithon and Mawsonites (Pickerill & Harris, Reference Pickerill and Harris1979; Seilacher et al. Reference Seilacher, Buatois and Mángano2005). Given their intimate association with synsedimentary deformational structures, such as convolute bedding, disrupted lamination and vertical clastic pipes, the central vertical cylindrical or funnel-shaped structures are consistent with conduits for fluid migration. Their internal lamination (rich in claystone-enriched films) and cut-and-fill features in conduits indicates that multiple pulses of sediment injection and displacement took place through these already developed preferential pathways. Triggers involved in this process are probably related to wave orbitals or tidal currents that induced stress over a cohesive (biostabilized) sedimentary surface. However, the resulting architecture observed in some samples remains intriguing, given their similarities to vertical funnel-shaped thick-lined burrows typical of those produced by terebellid polychaetes throughout the Phanerozoic Eon (Nara, Reference Nara1997, Reference Nara2002; Netto et al. Reference Netto, Tognoli, Assine and Nara2014). In this regard, we suggest that future studies focus on developing improved age constraints on the Cerro Negro Formation, with radiometric analyses and further palaeontological investigations. Also, expanding the use of sectioning and petrographic analysis to other discoidal assemblages may be useful to reveal similar interior structures, allowing us to compare the processes involved in their development.
Considering the current suggested age for the basal part of the Cerro Negro Formation, the affinity of the studied structures with the ichnogenera discussed here would imply extending their record back into the Ediacaran Period. However, assuming that this occurrence was the result of fluid injection beneath microbial mats seems a far more parsimonious scenario, given all the positive evidence we present for the effects of liquefaction and fluidization.
Acknowledgements
The authors thank the Cementos Avellaneda S.A for logistic support with fieldwork in Olavarría, Argentina, and Professors LF Cury and LKM Ozahata from the Laboratório de Análises de Minerais e Rochas (LAMIR) of the Paraná Federal University for their support with performing µCT analysis. The authors also thank the editor and two anonymous reviewers for their critical reading, comments and suggestions that helped to improve the original version of this manuscript. Financial support was provided by FAPESP (grant no. 2015/24608-3), and CNPq (grant no. 444070/2014-1). LV Warren, MG Simões and RG Netto are fellows of the CNPq.
Conflict of interest
None.
Supplementary material
To view supplementary material for this article, please visit https://doi.org/10.1017/S0016756821000327