1. Introduction
The fluorite (U–Th)/He thermochronology (FHe) technique was introduced by Evans et al. (Reference Evans, Wilson, Cline, McInnes and Byrne2005) and applied by Pi et al. (Reference Pi, Solé and Taran2005) as a geochronometer for dating mineralization events in the Taxco mining district in Mexico. This method has been developed and validated by Wolff (Reference Wolff2015) and Wolff et al. (Reference Wolff, Dunkl, Kempe and von Eynatten2015, Reference Wolff, Dunkl, Kempe, Stockli, Wiedenbeck and von Eynatten2016) as a new geo- and thermochronometer for dating hydrothermal mineralization, as well as subsequent low-temperature thermal events that may reset the FHe system. Recently, Alaminia et al. (Reference Alaminia, Tadayon, Griffith, Sol’e and Corfu2021) used fluorite thermochronometry in a Triassic carbonate-hosted fluorite-rich Mississippi-Valley-type (MVT) deposit in Central Iran. The very scattered FHe ages were interpreted without their uncertainty (437.6–4.6 Ma) as formation ages of fluorite during multiple mineralization events. These events were found to be linked to the successive extensional and compressional tectonic regimes during Cretaceous–Pliocene time, rather than exhumation-related cooling ages. The dual utility of the fluorite (U–Th)/He dating method (i.e. as both geo- and thermochronometer) is facilitated by the relatively large range of closure temperatures from 46 ± 14°C to 169 ± 9°C, attributed to different rates of helium diffusivity in the fluorite crystal lattice (Wolff et al. Reference Wolff, Dunkl, Kempe, Stockli, Wiedenbeck and von Eynatten2016). One of the major controls on helium diffusion and fluorite closure temperature is the substitutions of Ca2+ by rare earth elements (REEs) and yttrium (Y) in the fluorite crystal lattice (Wolff et al. Reference Wolff, Dunkl, Kempe, Stockli, Wiedenbeck and von Eynatten2016). Higher closure temperatures (up to c. 170°C) are attributed to a reduction of F vacancies in the fluorite crystal lattice due to increasing substitutions of Ca2+ by higher concentrations of ΣREEs + Y (Wolff et al. Reference Wolff, Dunkl, Kempe, Stockli, Wiedenbeck and von Eynatten2016). The relatively large range in fluorite closure temperature, together with the low-temperature hydrothermal conditions under which fluorite-rich MVT ore deposits form (mostly 90–150°C; Leach et al. Reference Leach, Apodaca, Repetski, Powell and Rowan1997, Reference Leach, Sangster, Kelley, Large, Garven, Allen, Gutzmer, Walters, Hedenquist, Thompson, Goldfarb and Richards2005; Wilkinson, Reference Wilkinson, Holland and Turekian2014), make the fluorite geo- and thermochronometry an ideal method for dating (1) the mineralization (i.e. formation age) and (2) subsequent exhumation events (i.e. cooling age related to exhumation) in ore deposits situated in fold and thrust belts. A fluorite with a high closure temperature should therefore be able to yield the formation age, and a fluorite with a low closure temperature will show a cooling age at the same sample.
We applied the FHe dating method to the Triassic carbonate-hosted fluorite ore deposits of the Mazandaran Fluorspar Mining District (MFMD) located in the east Central Alborz Mountains, northern Iran (Fig. 1b). Our main aims in using this newly established geo- and thermochronologic method were to evaluate the efficiency of this method for dating the fluorite mineralization in the MFMD, and elucidating the exhumation history of the east Central Alborz Mountains, where thermochrometric data are absent. In this study we show that fluorite He chronometry is an effective geochronometer for dating the mineralization in fluorite ore deposits (as indicated by Evans et al. Reference Evans, Wilson, Cline, McInnes and Byrne2005; Pi et al. Reference Pi, Solé and Taran2005; Wolff et al. Reference Wolff, Dunkl, Kempe and von Eynatten2015). We also extend the thermochronometric database to the east of the Alborz Mountains to reveal exhumation events related to Cenozoic regional deformation in the mainly carbonate rock units.

Fig. 1. (a) Simplified tectonic map of northern Iran and neighbouring regions (modified from Zanchetta et al. Reference Zanchetta, Zanchi, Villa, Poli, Muttoni, Brunet, Wilmsen and Granath2009). The box refers to the MFMD. (b) Map showing all published thermochronometric data in Central and west Central Alborz Mountains. There is a gap in thermochronometric data in the east Central Alborz Mountains, where we used the newly established fluorite-based (U–Th)/He thermochronometry to produce FHe ages and complete the Alborz Mountains thermochronometric database.
2. Geological and geodynamic background
2.a. Geological setting of the Alborz Mountains
The Alborz Mountains are c. 600 km long and c. 120 km wide intra-continental double-verging mountain range, located along the northern margin of the Arabia–Eurasia collision zone adjacent to the South Caspian Basin (SCB; Rezaeian et al. Reference Rezaeian, Carter, Hovius and Allen2012; Ballato et al. Reference Ballato, Landgraf, Schildgena, Stockli, Fox, Ghassemie, Kirby and Strecker2015; Fig. 1a). Major orogenic events affecting the Alborz Mountains comprise (1) Late Neoproterozoic subduction-related magmatism and Early Palaeozoic collision (i.e. Proto-Tethys Ocean closure between Peri-Gondwanan terrains); (2) the Late Triassic Cimmerian orogeny (i.e. Palaeo-Tethys ocean closure and collision of Iran, a micro-plate of Gondwanan affinity, with the Turan block along the southern margin of Eurasia plate); (3) Late Cretaceous compression and folding as recorded in the southern Alborz Mountains (e.g. Taleghan area); (4) late Paleocene – Eocene extension and volcanism; (5) continued deformation associated with Oligocene–Miocene uplift and exhumation related to the Arabia–Eurasia collision; (6) Pliocene exhumation; and (7) Pleistocene shift of deformation from right-lateral transpression to left-lateral transtension documented through palaeo-seismological studies along major faults (i.e. Taleghan and Mosha faults) in the west Central Alborz Mountains (Şengör & Kidd, Reference Şengör and Kidd1979; Berberian & King, Reference Berberian and King1981; Şengör, Reference Şengör1984; Alavi, Reference Alavi1994, Reference Alavi1996; Axen et al. Reference Axen, Lam, Grove, Stockli and Hassanzadeh2001; Allen et al. Reference Allen, Ghassemi, Shahrabi and Qorashi2003; Guest et al. Reference Guest, Axen, Lam and Hassanzadeh2006 a, b; Ritz et al. 2006; Hassanzadeh et al. Reference Hassanzadeh, Stockli, Horton, Axen, Stockli, Grove, Schmitt and Walker2008; Horton et al. Reference Horton, Hassanzadeh, Stockli, Axen, Gillis, Geust, Amini, Fakhri, Zamanzadeh and Grove2008; Brunet et al. Reference Brunet, Granath, Wilsmen, Brunet, Wilmsen and Granath2009; Ballato et al. Reference Ballato, Mulch, Landgraf, Strecker, Dalconi, Friedric and Tabatabaei2010, Reference Ballato, Stockli, Ghassemi, Landgraf, Strecker, Hassanzadeh, Friedrich and Tabatabaei2013, Reference Ballato, Landgraf, Schildgena, Stockli, Fox, Ghassemie, Kirby and Strecker2015; Nazari & Shahidi, Reference Nazari and Shahidi2012; Rezaeian et al. Reference Rezaeian, Carter, Hovius and Allen2012; Madanipour et al. Reference Madanipour, Ehlers, Yassaghi, Rezaeian, Enkelmann and Bahroudi2013, Reference Madanipour, Ehlers, Yassaghi and Enkelmann2017).
The stratigraphic successions preserved in the Alborz Mountains vary in thickness from c. 7 to c. 12 km, and include the Palaeozoic epicontinental/shallow-marine successions, Permian – Middle Triassic carbonate ramp/platform strata, Upper Triassic – Lower Jurassic foreland sediments, Middle Jurassic – Cretaceous epicontinental shelf carbonates and Cenozoic siliciclastic and pyroclastic sediments (Assereto, Reference Assereto1966; Alavi, Reference Alavi1991; Fig. 2). The pre-Late Triassic successions were deposited along a passive margin of the Palaeo-Tethys Ocean (Stampfli et al. Reference Stampfli, Marcoux and Baud1991). The aforementioned successions that were deformed during the Late Triassic Eo-Cimmerian orogeny (Fursich et al. Reference Fursich, Wilmsen, Seyed-Emami, Majidifard, Brunet, Wilmsen and Granath2009; Wilmsen et al. Reference Wilmsen, Fursich, Seyed-Emami, Majidifard and Taheri2009; Fig. 2) are overlain by the siliciclastic sediments of the Shemshak Formation with angular unconformable contact. Upper Jurassic–Cretaceous shallow-water limestone and marl, intercalated with uppermost Jurassic basaltic flows, have also been locally preserved along the length of the Alborz. The folded and thrusted Cretaceous limestones are covered by the Paleocene Fajan conglomerates (e.g. Guest et al. Reference Guest, Stockli, Grove, Axen, Lam and Hassanzadeh2006 b). The Eocene volcanic and pyroclastic rocks of the Karaj Formation generally represent a back-arc magmatism in the Alborz Mountains (Asiabanha & Foden, Reference Asiabanha and Foden2012) related to N-wards subduction of the Neo-Tethys oceanic lithosphere below Central Iran during the Eocene Epoch (i.e. 49–36 Ma; CS Verdel, unpub. Ph.D. thesis, California Institute of Technology, USA, 2008; Ballato et al. Reference Ballato, Mulch, Landgraf, Strecker, Dalconi, Friedric and Tabatabaei2010). The Neogene syn-orogenic deposits have developed within major intermountain basins (e.g. Taleqan, Alamout and Manjil) and also in the frontal parts of the Alborz Mountains (e.g. Guest et al. Reference Guest, Axen, Lam and Hassanzadeh2006 a; Ballato et al. Reference Ballato, Nowaczyk, Landgraf, Strecker, Friedrich and Tabatabaei2008, Reference Ballato, Uba, Landgraf, Strecker, Sudo, Stockli, Friedrich and Tabatabaei2011) (Fig. 2). Quaternary alluvial deposits form thick accumulations in northern and southern flanks of the Alborz Mountains, where they strongly deformed along major orogen-scale active faults such as North Teheran and Qazvin faults in the south and Khazar and Talesh faults in the north (e.g. Ritz et al. 2006; Nazari et al. Reference Nazari, Ritz and Oghbaee2007).

Fig. 2. Simplified tectonostratigraphy scheme of the Alborz Mountain Range (modified from Allen et al. Reference Allen, Ghassemi, Shahrabi and Qorashi2003) and location of the fluorite mineralization in Middle Triassic and Lower Cretaceous carbonate sequences.
Based on the available thermochronometric data (Fig. 1b), the Alborz–Talesh Mountains experienced several deformation and related exhumation events during Cenozoic time (Axen et al. Reference Axen, Lam, Grove, Stockli and Hassanzadeh2001; Guest et al. Reference Guest, Stockli, Grove, Axen, Lam and Hassanzadeh2006 b, Reference Guest, Horton, Axen, Hassanzadeh and McIntosh2007; Rezaeian et al. Reference Rezaeian, Carter, Hovius and Allen2012; Ballato et al. Reference Ballato, Stockli, Ghassemi, Landgraf, Strecker, Hassanzadeh, Friedrich and Tabatabaei2013, Reference Ballato, Landgraf, Schildgena, Stockli, Fox, Ghassemie, Kirby and Strecker2015; Madanipour et al. Reference Madanipour, Ehlers, Yassaghi, Rezaeian, Enkelmann and Bahroudi2013, Reference Madanipour, Ehlers, Yassaghi and Enkelmann2017). The first published study on the cooling history of the Alborz Mountains has been restricted to small areas from two plutons in the west Central Alborz (Axen et al. Reference Axen, Lam, Grove, Stockli and Hassanzadeh2001). The age-elevation plot of the (U–Th)/He data from the plutons implies that c. 5–7 km of exhumation occurred after c. 7 Ma in the west Central Alborz Mountains. However, this estimation has been modified in later studies, where in the west Central Alborz Mountains an exhumation rate of c. 0.1 km Ma–1 before 12 Ma, that accelerated to c. 0.45 km Ma–1 after c. 12 Ma, was suggested; this led to c. 5 km of exhumation in this part of the Alborz Mountains (Guest et al. Reference Guest, Axen, Lam and Hassanzadeh2006 a).
The reconstruction of the history of the Alborz Mountains mainly from Apatite Fission Tracks (AFT) analysis shows the oldest cooling ages at c. 39 Ma, preserved only in samples of intrusive rocks of Middle Eocene age (Rezaeian et al. Reference Rezaeian, Carter, Hovius and Allen2012). The oldest AFT ages are probably due to magmatic cooling related to magmatic activity that was still very intense in the southern flank of the Alborz Mountain (Vincent et al. Reference Vincent, Allen, Ismail-Zadeh, Flecker, Foland and Simmons2005; Ballato et al. Reference Ballato, Uba, Landgraf, Strecker, Sudo, Stockli, Friedrich and Tabatabaei2011; Verdel et al. Reference Verdel, Wernicke, Hassanzadeh and Guest2011). The youngest AFT ages between c. 32 and 16 Ma were detected in samples from pre-Tertiary Formations (i.e. Jurassic siliciclastics of Shemshak Formation), and have been attributed to cooling events associated with two erosional exhumation phases coinciding with unconformities and related coarse clastic sedimentation (Lower Red and Upper Red formations, referred to as LRF and URF, respectively).
Detrital and bedrock AFT, apatite and zircon helium thermochronometry (AHe and ZHe) cooling ages from the Talesh Mountains at the northwestern continuation of the Alborz Mountains also indicate three major cooling events during the late Oligocene Epoch (c. 27–23 Ma), middle Miocene (c. 12 Ma) and early Pliocene (c. 5 Ma) epochs. Thermochronological data indicate cooling at c. 27–23 Ma in the Talesh Mountains, similar to other parts of the Alborz Mountains; this has been attributed to a deformation event related to the early collision of the Afro-Arabian and Eurasian plates (Madanipour et al. Reference Madanipour, Ehlers, Yassaghi, Rezaeian, Enkelmann and Bahroudi2013, Reference Madanipour, Ehlers, Yassaghi and Enkelmann2017). Middle Miocene (c. 12 Ma) rapid cooling has also been attributed to deformation events related to a more advanced stage of collision between the Afro-Arabian and Eurasian plates. Early Pliocene cooling may also be due to the reorganization of the collision zone or exhumation related to base level fall of the Caspian Sea (Rezaeian et al. Reference Rezaeian, Carter, Hovius and Allen2012; Madanipour et al. Reference Madanipour, Ehlers, Yassaghi, Rezaeian, Enkelmann and Bahroudi2013; Ballato et al. Reference Ballato, Landgraf, Schildgena, Stockli, Fox, Ghassemie, Kirby and Strecker2015). All previously published thermochronometric data are from the central and western parts of the Alborz Mountains; there are no data from the east Central Alborz Mountains (Fig. 1b).
2.b. Geology of the MFMD
Neoproterozoic–Ordovician epi-continental sediments are the oldest rock units in the MFMD, followed by Devonian–Carboniferous continental shelf successions (i.e. Geiroud and Doroud formations). The latter are found in the southern part of the studied area adjacent to the Firouzkuh Fault (Fig. 3a). Continental ramp and shelf carbonate Permo-Triassic (Ruteh Formation), Middle Triassic (Elika Formation), Middle–Upper Jurassic (Delicahy and Lar formations) and Lower Cretaceous (TizKuh Formation) strata, together with Upper Triassic – Lower Jurassic siliciclastic sediments (Shemshak Formation), form the major lithological units in the MFMD (Fig. 3a). Alkaline mafic igneous rocks (i.e. basalt, diabase and gabbro) are mainly exposed in the central part of the MFMD (Fig. 3a). The Paleocene Fajan conglomerate occurs as limited outcrops in the central part of the MFMD, and is overlain unconformably by the Jurassic–Cretaceous carbonate strata of the Delichay, Lar and TizKuh formations (Fig. 3a). These clastic sediments and the older deformed sequences are unconformably overlain by the Eocene volcanic and pyroclastic rocks of the Karaj Formation throughout the MFMD. After a cessation of sedimentation during the Oligocene Epoch, Miocene clastic sediments of the URF were preserved adjacent the North Alborz Fault to the north and Firouzkuh Fault to the south of the MFMD (Fig. 3a). The upper part of the Middle Triassic Elika Formation occurs below a major erosional unconformity covered by the Upper Triassic – Lower Jurassic siliciclastic sediments of Shemshak Formation (Figs 2, 3). This unit hosts a large number of fluorite-barite-galena deposits (Fig. 3a; Vahabzadeh et al. Reference Vahabzadeh, Khakzad, Rasa and Mosavi2008, Reference Vahabzadeh, Khakzad, Rasa and Mosavi2009; Rajabi et al. Reference Rajabi, Rastad and Canet2013; Zabihitabar & Shafiei, Reference Zabihitabar and Shafiei2015; Mehraban et al. Reference Mehraban, Shafiei and Shamanian2016; B. Shafiei, unpub. research report, Golestan University Gorgan, Iran, 2016; Nabiloo et al. Reference Nabiloo, Shafiei and Amini2017).

Fig. 3. (a) Simplified geological map of the Mazandaran Fluorite Mining District (MFMD) in the northern flank of the Alborz Mountains and location of fluorite ore deposits and samples. Litho-facies units derived from Alavi (Reference Alavi1996), main structural features from the 1:250000 scale geological maps (Sari, Semnan, Amol and Tehran published by Geological Survey of Iran). (b) Three cross sections through most litho-facies units of the MFMD showing structural and stratigraphic position of the studied fluorite deposits, samples and their FHe average ages.
Geographically, the fluorite mineralization in the MFMD can be divided into three major districts: (1) the Savadkuh district (Pachi-Miana, SheshRoodbar, Baijan, Deraseleh and Ashjal mines); (2) the Khatirkuh district (KamarPosht, SarChalashk, Shoorakchal and Kharch mines); and (3) the Kiasar district (Era and Alikola mines). In addition, there is evidence of fluorite mineralization in Lower Cretaceous carbonate rocks of the TizKuh Formation (i.e. Kerman and Emaft fluorite deposit; Vahabzadeh et al. Reference Vahabzadeh, Khakzad, Rasa and Mosavi2008, Reference Vahabzadeh, Khakzad, Rasa and Mosavi2009), only 2 km from KamarPosht deposit (Fig. 3a). All districts cover more than 2400 km2 of the east Central Alborz Mountains forming the MFMD (Fig. 3a).
The MFMD had been in production since 1990, with estimated ore reserves of c. 6×105 tonnes fluorite and several tens of thousands of tonnes of galena, barite and celestine. These strata-bound deposits have similar host rock lithology, orebody geometry, mineral assemblage and ore textures, and are genetically linked to sedimentary basins and the tectonic evolution of the central Alborz Mountain (B Shafiei, unpub. research report, Golestan University, Gorgan, Iran, 2016). The fluorite ore deposits of the MFMD have been classified as fluorite-rich MVT deposits based on the above-mentioned characteristics (Rajabi et al. Reference Rajabi, Rastad and Canet2013). Structurally, the MFMD is bounded to the north by the North Alborz reverse fault (Rajabi et al. Reference Rajabi, Rastad and Canet2013) and to the south by the Firuzkuh and Astaneh strike-slip faults (Fig. 3a). The strike-slip faulting and deformation is also dominant in the MFMD; however, the northern boundary of the area is restricted with E–W-trending thrust faults (Fig. 3a, b). Fluorite deposits are mostly exposed along the limbs of anticlines that have been cut through by thrust and strike-slip faults (Fig. 3b).
Past mining in the MFMD was restricted to surficial outcrops of ore bodies, dominantly aggregates of fluorites, barite, celestine and galena filling cavities and fissure within Middle Triassic carbonate rocks of the Elika Formation, but they are also currently being excavated by underground tunnelling (Fig. 4). On a deposit scale, the mineable zones are mostly close to the Upper Triassic – Lower Jurassic shale units of Shemshak Formation (Fig. 4). Ore bodies can be massive and lenticular or veins, cavity and fracture fills, and/or can occur as full or partial replacement zones within thick-bedded limestone and dolomitic limestone. The economic ore zones are often fault-related breccia, composed of fluorite, barite, celestine and galena as the main ore mineral, with scarce sphalerite and calcite as common gangue mineral (Rastad & Shariatmadar, Reference Rastad and Shariatmadar2001; Rajabi et al. Reference Rajabi, Rastad and Canet2013; Zabihitabar & Shafiei, Reference Zabihitabar and Shafiei2015; Mehraban et al. Reference Mehraban, Shafiei and Shamanian2016; Nabiloo et al. Reference Nabiloo, Shafiei and Amini2017; Fig. 5).

Fig. 4. Field photographs of mineable zones in fluorite deposits of the MFMD. (a) Mining in altered Middle Triassic carbonate host rock of Elika Formation overlain by Upper Triassic shale unit of Shemshak Group, KamarPosht deposit. (b) Boulders of mineralized Middle Triassic host rock within Upper Triassic carbonaceous shale unit as basal part of Shemshak Group, KamarPosht deposit. (c) Faulted contact between altered Middle Triassic carbonate host rock and Upper Triassic carbonaceous shale unit, KamarPosht deposit. (d) Lens-shaped orebody within Middle Triassic limestone located in contact with altered Upper Triassic shale unit, Era deposit. (e) Massive orebody located in altered carbonate rocks near to a faulted zone, SheshRoodbar deposit.
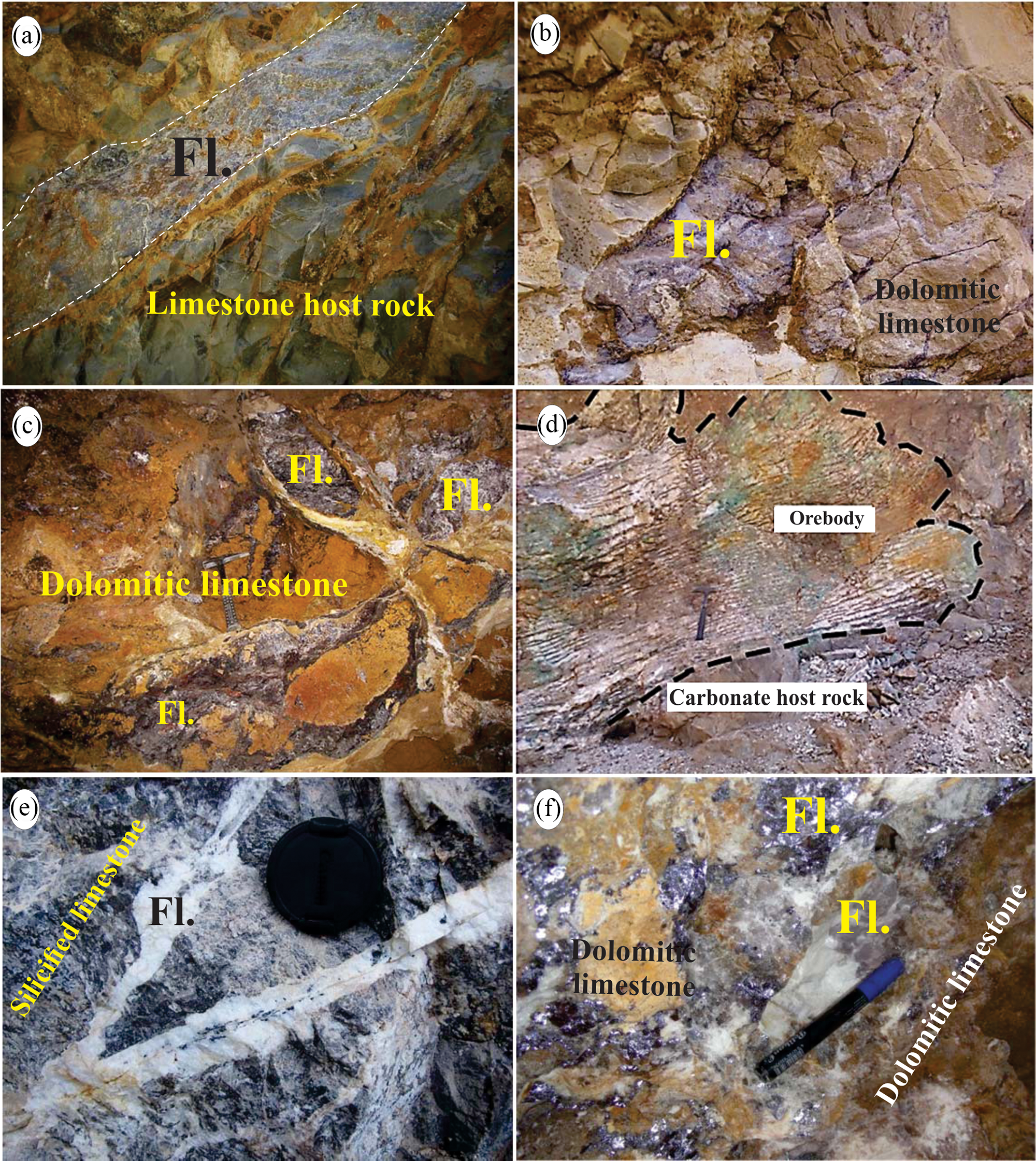
Fig. 5. Outcrop photographs showing the mode of occurrence of fluorite in mineable zones of the MFMD. (a) Vein of fluorite (Fl) discordant to bedding of limestone host rock, KamarPosht deposit. (b) Vein and veinlets of purple fluorite (Fl) in limestone host rock, Era deposit. (c) Dissolution breccia and open-space-filling textures of fluorite mineralization in dolomitic limestone host rock, KamarPosht deposit. (d) Zebra texture as an epigenetic stage of mineralization in limestone host rock, SheshRoodbar deposit. (e) Veins of white and sacrosic fluorite (Fl) within silicified limestone host rock, Era deposit. (f) Open-space filling and dissolution breccia texture for fluorite (Fl) mineralization in dolomitic limestone host rock, KamarPosht deposit.
Ore morphology and fabrics indicate that mineralization is younger that the Middle Triassic carbonate host rocks. This is supported by a three-point Sm–Nd isotope isochron age of fluorite samples from the Kamarposht deposit that yielded an age of 90 ± 23 Ma (mean square weighted deviation, MSWD = 1.9) with a relatively large range of initial Nd (ϵNd = −4.61 ± 2.57; B Shafiei, unpub. research report, Golestan University, Gorgan, Iran, 2016). This age is at least 100 Ma younger than the host rock, and indicates that the mineralization age cannot be related to the Eo-Cimmerian (Late Triassic) orogenic event as previously suggested (Rajabi et al. Reference Rajabi, Rastad and Canet2013). Considering the localization of fluorite mineralization in carbonate strata of the Middle Triassic Elika Formation (i.e. Pachi-Miana, SheshRoodbar, KamarPosht, Era and Alikola fluorite ore deposits) and the Early Cretaceous TizKuh Formation (i.e. Kerman and Emaft fluorite ore deposits), it can be concluded that epigenetic mineralization formed throughout the MFMD during the Late Cretaceous Epoch (Fig. 3a). This is further corroborated by Sr–Nd–Pb isotope data showing higher 87Sr/86Sr values in fluorite and barite (0.7086–0.7089) than in the Triassic marine water (c. 0.7079) and carbonate host rocks (c. 0.7082) (A. Davoodi, unpub. M.Sc. thesis, Tehran University, Tehran, Iran, 1997).
Model ages of galena Pb isotopes younger than 200 Ma (i.e. Mirnejad & MolaSalehi, Reference Mirnejad and MolaSalehi2009) and variable initial Nd isotopic ratios (143Nd/144Nd) of fluorite are also indicative of a younger mineralization event than the Triassic in the MFMD (B Shafiei, unpub. research report, Golestan University, Gorgan, Iran, 2016). Available fluorite fluid inclusion data from the MFMD have yielded homogenization temperatures ranging between 80°C and 190°C, and salinities of 12 and 33 wt% NaCl equivalent (A Shariatmadar, unpub. M.Sc. thesis, Tarbiat Modaress University, Tehran, Iran, 1999; Z Salemi, unpub. M.Sc. thesis, Tehran University, Tehran, Iran, 1997). Such microthermometric data suggest that mineralization in the MFMD is governed by slightly hot and saline ore-forming solutions containing heterogenic Sr–Nd–Pb isotope composition originated from continental basinal brine rather than syn-deposition from marine water simultaneously with deposition of the Middle Triassic Elika Formation (B Shafiei, unpub. research report, Golestan University, Gorgan, Iran, 2016). Similar hydrothermal fluid conditions have been reported from the Illinois–Kentucky fluorspar district in the USA and the South Pennine Orefield in England (Chesley et al. Reference Chesley, Halliday, Kyser and Spry1994; Bau et al. Reference Bau, Romer, Luders and Dulski2003; Barker et al. Reference Barker, Bennett, Cox, Norman and Gagan2009) as well as fluorite-barite Komsheche deposit in Central Iran (Alaminia et al. Reference Alaminia, Tadayon, Griffith, Sol’e and Corfu2021).
3. Materials and methods
Fluorite (U–Th)/He thermochronology was performed on 32 aliquots of 6 ore samples, including 5 colourless and 1 purple variety (4 and 6 crystal aliquots per sample; Tables 1, 2; Fig. 6) from four carbonate-hosted fluorite ore deposits. The fluorite single crystals were inspected for inclusions under 250× magnification and cross-polarized light. Only inclusion-free fragments were selected. The crystals were wrapped in platinum capsules of c. 1 × 1 mm size and were heated in the full-metal extraction line by an infrared laser for 2 min in high vacuum. The extracted gas was purified using a SAES Ti-Zr getter kept at 450°C. The chemically inert noble gases and a minor amount of other gases were then expanded into a Hiden triple-filter quadruple mass spectrometer equipped with a positive ion-counting detector. Beyond the detection of helium, the partial pressures of some restite gases were continuously monitored (H2, CH4, H2O, N2, Ar and CO2). The crystals were checked for degassing of helium by sequential re-heating and helium measurement. The amount of helium extracted in the second runs is usually below 1%. Following degassing, the samples were retrieved from the gas extraction line, and spiked with calibrated 230Th and 233U solutions. The fluorite crystals were dissolved in ultrapure 30% HCl in Savillex Teflon vials. Each sample batch was prepared with a series of procedural blanks to check the purity and calibration of the reagents and spikes. Spiked solutions were analysed by a Perkin Elmer Elan DRC II or by a Thermo iCAP Q inductively coupled plasma mass spectrometer (ICP-MS) with an APEX micro-flow nebulizer. Ca, Sm, Pt, REEs and Y were determined by external calibration. The (U–Th)/He ages were determined by the Taylor expansion method, and the Sm content was also considered for the age calculation. The concentration of U, Th, REEs + Y in fluorite single-crystal aliquots along with the FHe ages are presented in Tables 1 and 2.
Table 1. REEs, Y, U and Th concentrations (in ppm) in the single-fragment fluorite aliquots from the ore deposits in the MFMD
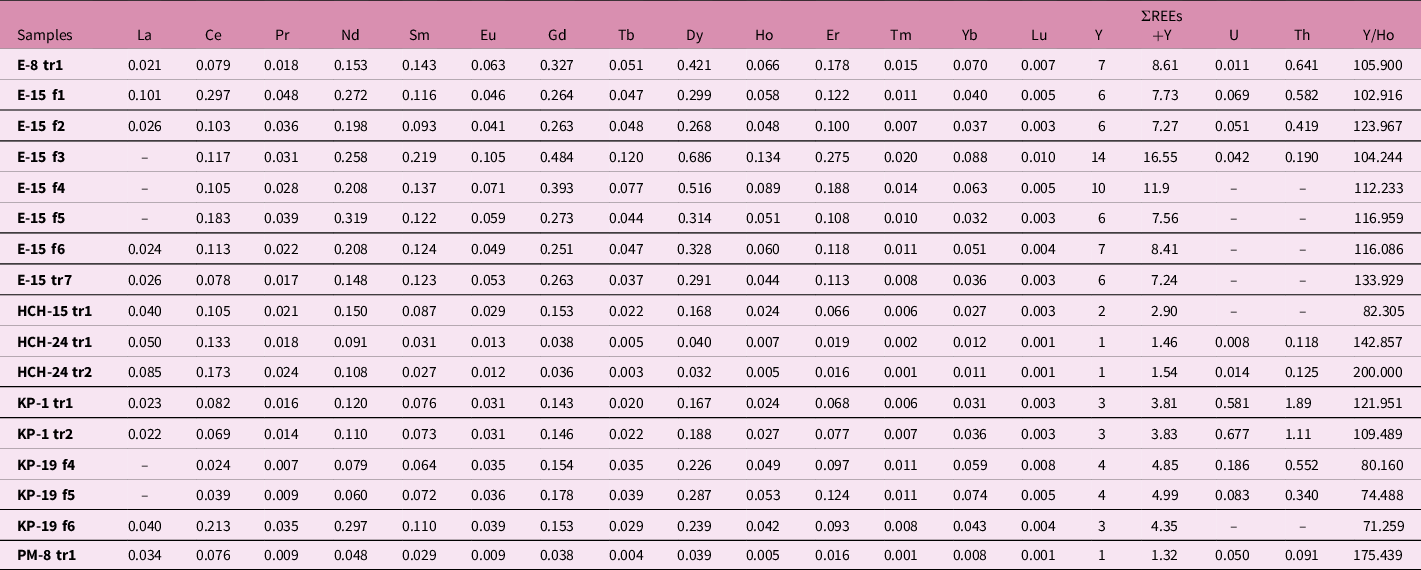
Table 2. Fluorite (U–Th)/He data obtained on samples from the ore deposits of east Central Alborz Mountain Range, northern Iran. The amount of helium is given in nanograms per cubic centimetre at standard temperature and pressure, while the amounts of radioactive elements are given in nanograms

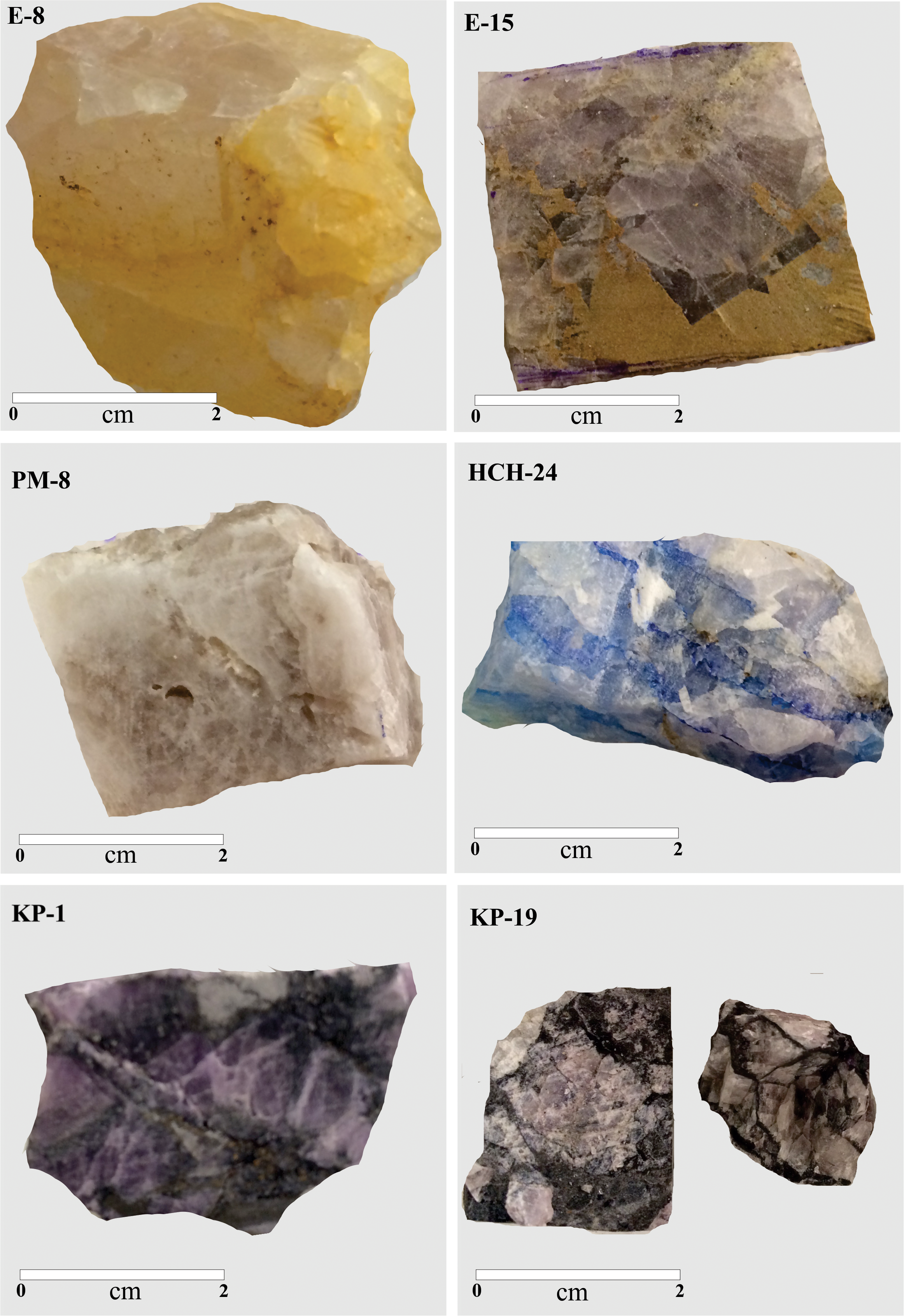
Fig. 6. Hand specimens of fluorites dated in this study. Ore deposits location of the samples is Era (samples E-8 and E-15), SheshRoodbar (sample HCH-24), Pachi-Miana (sample PM-8) and KamarPosht (samples KP-1 and KP-19).
4. Results
4.a. U, Th, Sm and REEs + Y concentrations in the dated fluorite samples
The colourless fluorites have very low concentrations of U, Th and Sm (0.005–0.2 , 0.09–0.8 and 0.06–0.46 ppm, respectively; Table 1). In contrast, aliquots of single purple fluorite in this study (sample KP-1 from the KamarPosht deposit) are characterized by a relatively constant but higher concentrations of actinides (U = 0.34–0.8 ppm; Th = 1.04–2.0 ppm). Moreover, all 32 fluorite single-fragment aliquots show very low ∑REEs + Y concentrations of 1.3–16.6 ppm. This range is within the concentration range of ∑REEs observed in fluorites from the Illinois–Kentucky fluorspar district in the USA (0.6–19 ppm; Chesley et al. Reference Chesley, Halliday, Kyser and Spry1994) and also matches the data obtained from samples in the Eastern Alps (0.1–17 ppm; Schneider et al. Reference Schneider, Moller and Parekh1975; Table 1; Fig. 7a). All fluorite single-crystal aliquots displayed similar REE patterns and have a general depletion in light REEs (LREEs) and a relative enrichment in middle REEs (MREEs) and Y (Fig. 7b). The average concentrations of REEs in fluorite samples are significantly lower than those of magmatic fluorites (Fig. 7c). The fluorites of the MFMD also have a relatively narrow range of Y/Ho ratios (71–200), within the range of hydrothermal fluorite-rich MVT deposits in South Pennine Orefield (30–200), but larger than the ranges for seawater (20–40) and igneous rocks (40–75; Bau & Dulski, Reference Bau and Dulski1995) (Fig. 7d).

Fig. 7. Geochemical plots of the dated fluorite samples from the MFMD in this study. (a) ∑REEs + Y concentration, which mostly falls into the range of typical sedimentary basin fluorites around the world. (b) NASC (North American shale composite) -normalized REE pattern for dated fluorites suggests a similar origin. (c) Comparative NASC-normalized REE variation trends for average samples of dated fluorites in this study and sedimentary basin, and magmatic fluorites around the world. (d) Comparison between the range of Y/Ho ratios of the dated fluorites compared with those of hydrothermal, magmatic or sedimentary basin origin. Range and average REEs for fluorites derived from Schneider et al. (Reference Schneider, Moller and Parekh1975), Chesley et al. (Reference Chesley, Halliday, Kyser and Spry1994), Bau & Dulski (Reference Bau and Dulski1995) and Bau et al. (Reference Bau, Romer, Luders and Dulski2003). Calculated average of REEs for magmatic hydrothermal fluorites derived from REE data reported in Ekambaram et al. (Reference Ekambaram, Brookins, Rosenburg and Emanuel1986), Constantopoulos (Reference Constantopoulos1988), Ronchi et al. (Reference Ronchi, Tauray, Michard and Dardenne1993), Palmer & Williams-Jones (Reference Palmer and Williams-Jones1996), Williams-Jones et al. (Reference Williams-Jones, Samson and Olivo2000), Schwinn & Markl (Reference Schwinn and Markl2005), Schönenberger et al. (Reference Schönenberger, Köhler and Markl2008), Sanchez et al. (Reference Sanchez, Cardellach and Corbella2010) and Dill et al. (Reference Dill, Hansen and Weber2011).
4.b. FHe age data and their distribution
Despite the low concentration and low variability of U and Th, and assuming the same formation age of the fluorite samples in this study (c. 90 Ma age of Sm/Nd geochronometry), our FHe ages have large variations ranging from 84.5 ± 3.6 to 19.5 ± 1.1 Ma. This age spread is also observed within the aliquots of the same sample and between samples from the same deposit (e.g. samples KP-1 and Kp-19), and this age variation is more significant in colourless fluorite crystals compared with the purple crystals (i.e. sample KP-1; Table 2; Fig. 8a).

Fig. 8. (a) Histogram showing the abundance distribution of fluorite (U–Th)/He ages obtained in four ore deposits from the MFMD, Alborz Mountains, northern Iran and three identified events based on the interpretation of FHe ages in this study. (b) The FHe ages date the age of the fluorite mineralization as Late Cretaceous, and show two subsequent low-temperature tectono-thermal events interpreted as exhumation-related cooling ages. The first exhumation event probably took place during the Eocene Epoch and was related to the extensional tectonic regime or volcanic thermal relaxation, and the second is due to Oligo-Miocene compressional exhumation cooling. References for major geological events or conditions known to have occurred in the Alborz Mountains during Triassic–Tertiary time are derived from Stocklin (Reference Stocklin1968), Berberian & King (Reference Berberian and King1981), Alavi (Reference Alavi1996), Axen et al. (Reference Axen, Lam, Grove, Stockli and Hassanzadeh2001), Guest et al. (Reference Guest, Axen, Lam and Hassanzadeh2006a, b, Reference Guest, Horton, Axen, Hassanzadeh and McIntosh2007), Zanchi et al. (Reference Zanchi, Berra, Mattei, Ghassemi and Sabouri2006, Reference Zanchi, Zanchetta, Garzanti, Balini, Berra, Mattei, Muttoni, Brunet, Wilmsen and Granath2009), Brunet et al. (Reference Brunet, Granath, Wilsmen, Brunet, Wilmsen and Granath2009), Fursich et al. (Reference Fursich, Wilmsen, Seyed-Emami, Majidifard, Brunet, Wilmsen and Granath2009), Wilmsen et al. (Reference Wilmsen, Fursich, Seyed-Emami, Majidifard and Taheri2009), Ballato et al. (Reference Ballato, Uba, Landgraf, Strecker, Sudo, Stockli, Friedrich and Tabatabaei2011, Reference Ballato, Stockli, Ghassemi, Landgraf, Strecker, Hassanzadeh, Friedrich and Tabatabaei2013, Reference Ballato, Landgraf, Schildgena, Stockli, Fox, Ghassemie, Kirby and Strecker2015), Rezaeian et al. (Reference Rezaeian, Carter, Hovius and Allen2012) and Nazari & Shahidi (Reference Nazari and Shahidi2012). The grey bars indicate growing of the formation of the Alborz Mountains and its growth from the Middle Mesozoic throughout Cenozoic time.
The distribution of FHe ages in aliquots of samples E-8 from the Era deposit have smaller age ranges (c. 55–35 Ma with an average age of 45 ± 6 Ma), whereas sample E-15 is characterized by the large inter-aliquots FHe age variation (c. 72.3–33.1 Ma with an average of c. 46 ± 3.6 Ma; Table 2; Fig. 8a). However, the calculated average age for both samples are close to each other and are mostly scattered around Eocene time (c. 55.4–33.1 Ma; Table 2; Fig. 8a). Also, aliquots of two samples from KamarPosht deposit have the largest inter-sample FHe age variation in this study, ranging from the oldest (c. 84.5 Ma, with an average age of 61 Ma in sample KP-19) to the youngest ages (19.5 Ma, with an average age of 23.20 Ma in sample KP-1). If we exclude the oldest age of 66 ± 19 Ma, the FHe ages of sample HCH-24 from the ShedhRoodbar deposit are relatively constant and range from 48 to 34 Ma (with an average age of 42 Ma). Finally, in the Pachi-Miana deposit, the inter-aliquots ages of sample PM-8 display a rather large variability ranging from 53 to 22 Ma (Table 2; Fig. 8a), with an average age of 39 Ma close to those of the other samples of the studied ore deposits.
5. Discussion
5.a. Reliability of the FHe age data
The large variation in FHe ages in aliquots of fluorite single crystals is an important issue in fluorite (U–Th)/He thermochronometry and has a strong impact on their geological interpretation (Wolff, Reference Wolff2015; Wolff et al. Reference Wolff, Dunkl, Kempe and von Eynatten2015). In our study, with the exception of samples E-8 and KP-1, the FHe ages between aliquots in most samples have a limited reproducibility (e.g. 84–39 Ma in sample KP-19, 53–22 Ma in sample PM-8 and 66–34 Ma in sample HCH-24). Possible factors that could explain the spread of FHe ages include: (1) analytical uncertainty; (2) the presence of parentless (non-radiogenic) helium; (3) the heterogeneous distribution of the alpha-emitting elements; and (4) the local variability of helium diffusivity in fluorite crystal lattice (Wolff et al. Reference Wolff, Dunkl, Kempe and von Eynatten2015, Reference Wolff, Dunkl, Kempe, Stockli, Wiedenbeck and von Eynatten2016).
The analytical uncertainties are the most verifiable sources of the bias in the ages. The typical uncertainty of the He, U and Th amounts are around 2–3%, as they are determined by isotope dilution technique. According to standard errors reported for FHe ages in this study, with the exception of the age of 66 Ma that has a high uncertainty of c. 20 Ma, the rest of our FHe ages are reliable, and the contribution of the analytical error to the spread of the ages can be considered as negligible. This unreliable age of 66 Ma has therefore been excluded from the average age calculation and the geological interpretation. Parentless helium can be trapped in the fluorite lattice and/or in fluid inclusions. Lattice-trapped helium cannot be accounted for, but the analysed samples were selected with extreme care and do not contain fluid inclusions down to the optical resolution of the microscopes (c. 1 µm); the latter effect can therefore be ruled out. Heterogeneity in the natural fluorite crystals could be an important issue, and by the optical screening techniques the heterogeneity within the dated crystal fragment and the influence of its potential ‘bad neighbours’ (Brown et al. Reference Brown, Beucher, Roper, Persano, Stuart and Fitzgeraldd2013) cannot be detected. We therefore consider that such unexplored factors may have played a role in generating the inter-aliquots FHe age variation.
5.b. Data interpretation
Even by excluding the unreliable FHe age of 60 Ma due to its high uncertainty, our FHe ages show a large age range between c. 85 and c. 20 Ma, and mostly scatter around Eocene time. On the one hand, the FHe ages are close to the mineralization age; on the other hand, they are comparable with previously published AHe, ZrHe and AFT thermochronometric data of the west and Central Alborz Mountains (Guest et al. Reference Guest, Axen, Lam and Hassanzadeh2006 a, b; Rezaeian et al. Reference Rezaeian, Carter, Hovius and Allen2012; Ballato et al. Reference Ballato, Stockli, Ghassemi, Landgraf, Strecker, Hassanzadeh, Friedrich and Tabatabaei2013, Reference Ballato, Landgraf, Schildgena, Stockli, Fox, Ghassemie, Kirby and Strecker2015; Madanipour et al. Reference Madanipour, Ehlers, Yassaghi, Rezaeian, Enkelmann and Bahroudi2013, Reference Madanipour, Ehlers, Yassaghi and Enkelmann2017, Reference Madanipour, Yassaghi, Ehlers and Enkelmann2018). If we consider the oldest FHe ages (84.5 ± 6, 78.8 ± 4 and maybe c. 72.3 ± 3.5 Ma) as mineralization ages, and exclude these three ages from the average age calculation, the rest of the ages yielded average values between 48–39 and c. 28–20 Ma, and can be considered as cooling ages after fluorite formation. Because three Late Cretaceous ages (c. 85, 79 and 72 Ma) and Late Cretaceous FHe ages have been reported in two fluorite deposits in this study, we conclude that at least two of the four fluorite deposits in this study have experienced pre-Cenozoic thermal events (i.e. hydrothermal mineralization). By this logic, we considered these three close ages as an age group in this study. These three age groups (i.e. c. 85–72, 48–39 and 28–20 Ma) therefore indicate that our samples have kept some memory of their formation (crystallization age) and multiple cooling events after crystallization (cooling ages), probably in response to episodic uplift and exhumation. Our stratigraphic and structural observations show that all samples are hosted in the carbonate strata of the Middle Triassic Elika and the Lower Cretaceous Tizkuh formations, mostly localized along the limbs of the anticlines, thrust and strike-slip faults (Fig. 3c; cross-sections). Therefore, taking into account the non-reset (c. 85–72 Ma) to completely reset (c. 48–20 Ma) thermochronometric ages in this study, our geologic interpretation of FHe ages includes a Late Cretaceous mineralization followed by two exhumation-related cooling events in Eocene and Oligo-Miocene times, as discussed in the following sections.
5.b.1. Late Cretaceous fluorite mineralization in east Central Alborz Mountains
The Late Cretaceous FHe ages of c. 85–72 Ma can be considered as mineralization ages for the MFMD, and could be explained by a genetic scenario proposed for similar fluorite mining districts around the world (the Illinois–Kentucky fluorspar district in the USA and the South Pennine ore field in England), where mineralization was formed by basinal brine fluid flow a few million years after the formation of the carbonate host rocks (Chesley et al. Reference Chesley, Halliday, Kyser and Spry1994; Bau et al. Reference Bau, Romer, Luders and Dulski2003). The migration of the ore-forming basinal fluids at shallow crustal levels could have been driven by magmatic or orogenic events that can generate thermally and tectonically driven fluid flow, respectively (Garven et al. Reference Garven, Ge, Person and Sverjensky1993; Garven & Raffensperger, Reference Garven, Raffensperger and Barnes1997; Leach et al. Reference Leach, Sangster, Kelley, Large, Garven, Allen, Gutzmer, Walters, Hedenquist, Thompson, Goldfarb and Richards2005; Pollyea et al. Reference Pollyea, Van Dusen and Fischer2015; Bahnan et al. Reference Bahnan, Carpentier, Pironon, Ford, Ducoux, Barré, Mangenot and Gaucher2020; Alaminia et al. Reference Alaminia, Tadayon, Griffith, Sol’e and Corfu2021). In the absence of orogenic process around the time of fluorite mineralization in the Alborz Mountains, burial heating plus magmatic heat flow (Nazari & Shahidi, Reference Nazari and Shahidi2012; Shekarifard et al. Reference Shekarifard, Baudin, Seyed-Emami, Schnyder, Laggoun-Defarge, Riboulleau, Brunet and Shahidi2012) could have resulted in basinal brine circulation and the formation of hydrothermal ore solution.
According to palaeo-heat-flow modelling of the Alborz basin during Jurassic–Cretaceous sedimentation by Shekarifard et al. (Reference Shekarifard, Baudin, Seyed-Emami, Schnyder, Laggoun-Defarge, Riboulleau, Brunet and Shahidi2012), east Central Alborz experienced a thermal anomaly and high geothermal gradients during this time interval. The high palaeo-heat-flow recorded in east Central Alborz is mainly related to higher temperatures resulting from greater depth of sedimentary burial as a result of the great thickness of the strata (c. 6 km thickness including 4 km thickness of Shemshak Formation + 2 km thickness of Jurassic–Cretaceous carbonate strata of Delichay, Lar and TizKuh formations) overlying the Middle Triassic carbonate host rocks of fluorite deposits (i.e. Elika Formation) relative to the western and eastern marginal parts of the Alborz Mountains (Shekarifard et al. Reference Shekarifard, Baudin, Seyed-Emami, Schnyder, Laggoun-Defarge, Riboulleau, Brunet and Shahidi2012). Although the lithostratigraphy and thickness of the strata overlying the Elika Formation in the MFMD are not available (because of the forested area), the proximity of the studied area to the sections where thermal modelling has shown the thermal anomaly (Shekarifard et al. Reference Shekarifard, Baudin, Seyed-Emami, Schnyder, Laggoun-Defarge, Riboulleau, Brunet and Shahidi2012) suggest that the MFMD probably experienced the same burial history and high heat-flow regime during the Middle Jurassic – Early Cretaceous epochs. This is nearly contemporaneous with the deposition of the epi-continental to continental platform carbonates of Delichay and Lar formations.
The high palaeo-heat-flow recorded in east Central Alborz is attributed to the rapid sedimentation, high subsidence rate and considerable thickness of the basin during the Late Cretaceous deposition of Elika Formation (Fursich et al. Reference Fursich, Wilmsen, Seyed-Emami, Cecca and Majidifard2005, Reference Fursich, Wilmsen, Seyed-Emami, Majidifard, Brunet, Wilmsen and Granath2009; Wilmsen et al. Reference Wilmsen, Fursich, Seyed-Emami, Majidifard and Taheri2009; Shekarifard et al. Reference Shekarifard, Baudin, Seyed-Emami, Schnyder, Laggoun-Defarge, Riboulleau, Brunet and Shahidi2012; Sheikholeslami, Reference Sheikholeslami2018). Another possible heat source in the MFMD could be associated with the Jurassic–Cretaceous alkaline mafic extrusive and intrusive igneous units that occur near the fluorite ore deposits and post-date the strata of the Elika and the Shemshak formations (Fig. 3a). Apparently, these are formed in an extensional back-arc basin setting and crustal thinning due to oblique subduction of Neo-Tethys Oceanic lithosphere beneath Central Iran (Wilmsen et al. Reference Wilmsen, Fursich, Seyed-Emami, Majidifard and Taheri2009; Doroozi et al. Reference Doroozi, Vaccaro, Masoudi and Petrini2016; Ghasemi et al. Reference Ghasemi, Rostami Hossuri and Sadeghian2018). This rift-related magmatism later caused the birth of the South Caspian Oceanic Basin during the late Early Jurassic Epoch, seafloor spreading was established by the Middle Jurassic Epoch, and the sedimentation continued into Cretaceous time in a shallow-marine setting (Zanchi et al. Reference Zanchi, Berra, Mattei, Ghassemi and Sabouri2006; Fursich et al. Reference Fursich, Wilmsen, Seyed-Emami, Majidifard, Brunet, Wilmsen and Granath2009; Sheikholeslami, Reference Sheikholeslami2018).
Given the high heat-flow prevailing in east Central Alborz during the Jurassic–Cretaceous time interval, the basinal brines circulated through pre-Late Cretaceous carbonate-evaporate and organic-siliciclastics potential source rocks and acquired the ore-forming elements (F, Ba, Pb, Zn, Sr). Ore deposition occurred at the beginning of the Late Cretaceous Epoch (c. 90 Ma based on Sm–Nd method and c. 85 Ma FHe age) within suitable host rocks (i.e. carbonate rocks of Middle Triassic Elika Formation and Lower Cretaceous TizKuh Formation). If we accept the Late Cretaceous FHe ages as the age of the fluorite mineralization, we can use the upper fluorite closure temperature (169 ± 9°C; Wolff et al. Reference Wolff, Dunkl, Kempe, Stockli, Wiedenbeck and von Eynatten2016) as the crystallization temperature of fluorite from the mineralizing hydrothermal solution. This temperature is comparable with the temperature of formation of the fluorite ore deposits in the MFMD (i.e. 80°C and 190°C; A Shariatmadar, unpub. M.Sc. thesis, Tarbiat Modaress University, Tehran, Iran, 1999; Z Salemi, unpub. M.Sc. thesis, Tehran University, Tehran, Iran, 1997) and also fluorite-rich MVT deposits around the world (mostly 90–150°C; Leach et al. Reference Leach, Apodaca, Repetski, Powell and Rowan1997, Reference Leach, Sangster, Kelley, Large, Garven, Allen, Gutzmer, Walters, Hedenquist, Thompson, Goldfarb and Richards2005; Wilkinson, Reference Wilkinson, Holland and Turekian2014; Alaminia et al. Reference Alaminia, Tadayon, Griffith, Sol’e and Corfu2021).
The epigenetic origin for fluorite mineralization by hydrothermal ore fluids originated from basinal brine during the Late Cretaceous Epoch in the MFMD, similar to the Illinois–Kentucky fluorspar district in the USA and South Pennine Orefield in England (Chesley et al. Reference Chesley, Halliday, Kyser and Spry1994; Bau et al. Reference Bau, Romer, Luders and Dulski2003) as well as in the fluorite-barite Komsheche deposit in Central Iran (Alaminia et al. Reference Alaminia, Tadayon, Griffith, Sol’e and Corfu2021), is also supported by: (1) highly variable ∑REE+Y concentrations; the hydrothermal signature of Y/Ho ratios and the positive Y anomalies of dated fluorite samples in this study indicate prolonged circulation and long migration pathway for the ore-forming solution (Jiang et al. Reference Jiang, Wang, Xu and Zhao2005; Jian et al. Reference Jian, Sun and Gao2015); (2) the heterogeneous Sr–Nd–Pb isotopic composition of fluorite, indicating the origin of the ore-forming elements as source rocks with different isotopic compositions; and (3) the moderate temperature and low salinity of fluorite-hosted fluid inclusions.
5.b.2. Eocene cooling related to extensional tectonic or magmatic thermal relaxation
Most of the FHe ages in the MFMD are scattered around Eocene time (c. 55–33 Ma), and this might represent one of the main cooling events recorded in the east Central Alborz Mountains. There is no evidence for prolonged exhumation related to the compressional regime during the Eocene Epoch in the Alborz Mountains and in the MFMD. In contrast, a regional extensional regime with prolonged volcanic and pyroclastic deposition related to the N-wards subduction of the Neo-Tethys Oceanic lithosphere further south beneath Central Iran (Berberian & King, Reference Berberian and King1981; Alavi, Reference Alavi1996; Guest et al. Reference Guest, Stockli, Grove, Axen, Lam and Hassanzadeh2006 b) prevailed in the Alborz Mountains (especially in the southern flank of the mountains as well as in the MFMD; Fig. 3a). This was accompanied by syn-depositional normal faulting and sedimentation in a highly active and rapidly subsiding back-arc extensional basin associated with the deposition of pyroclastics known as the Karaj Formation during Early–Middle Eocene time (e.g. Berberian & King, Reference Berberian and King1981; Davoudzadeh et al. Reference Davoudzadeh, Lammere and Weber-Diefenbach1997; Brunet et al. Reference Brunet, Korotaev, Ershov and Nikishin2003; Vincent et al. Reference Vincent, Allen, Ismail-Zadeh, Flecker, Foland and Simmons2005; Guest et al. Reference Guest, Stockli, Grove, Axen, Lam and Hassanzadeh2006 b, Reference Guest, Horton, Axen, Hassanzadeh and McIntosh2007; Ballato et al. Reference Ballato, Uba, Landgraf, Strecker, Sudo, Stockli, Friedrich and Tabatabaei2011; Nazari & Shahidi, Reference Nazari and Shahidi2012; Javadi et al. Reference Javadi, Kouhpeyma, Gholami Zadeha, Naeimi, Sheikholeslami and Ghassemi2020).
Eocene volcanism is relatively widespread in the study area (Fig. 3a); it could therefore have played an important role as a significant thermal event that effected the fluorite ore deposits. Since the volcanic magma forming the Karaj Formation had low water content (unlike the granitic magma containing 1−3 wt% magmatic water; Middlemost, Reference Middlemost1987), it is unlikely to have produced a hydrothermal fluid that could have overprinted the fluorites. In contrast, we suggested that the fluorite mining areas, which were heated due to an increased geothermal gradient by Eocene volcanism, re-cooled due to the geothermal gradient re-equilibration after the end of volcanism. This process would explain the Eocene FHe cooling ages in this study.
Alternatively, exhumation during extensional tectonics and normal faulting (e.g. Fitzgerald et al. Reference Fitzgerald, Fryxell and Wernicke1991; Stockli et al. Reference Stockli, Farley and Dumitru2000; Wolff et al. Reference Wolff, Hetzel, Dunkl, Anczkiewicz and Pomella2020) could also explain the observed Eocene cooling ages. For example, in the case of rapid extension, deep crustal levels can cool and be exposed at the surface near the fault plane with limited erosion. Since all our samples were collected near major faults in the eastern Alborz Mountains, the faults might experience normal kinematics during regional extension. The observed cooling ages may then reflect extension-related exhumation during the Eocene Epoch.
Stratigraphic evidence, especially from the Central Alborz Mountains where Eocene deposits are restricted to the Kandovan fault, indicates the basin bounding fault with possible normal kinematics during the Eocene Epoch (Yassaghi & Naeimi, Reference Yassaghi and Naeimi2011). Either of these scenarios (volcanism or exhumation) could be a possible explanation for the observed Eocene cooling ages in the east Central Alborz Mountains. These processes can also act together; however, detailed structural, stratigraphic and volcanological studies need to be applied regionally to determine which of the two possible scenarios could be responsible for the Eocene cooling event.
5.b.3. Late Oligocene – early Miocene exhumation-related cooling
The last major cooling event documented in the MFMD by our FeHe age data occurred at c. 27–20 Ma, as recorded in one sample from the KamarPosht fluorite deposit located close to the hanging wall of the Firozkuh fault system. This possibly records the latest deformation event along the Firozkuh fault as having occurred during Late Oligocene time.
At the Eocene–Oligocene boundary, the onset of continental collision between the Arabian and Iranian micro-plates along the Bitlis–Zagros suture caused an increase in plate coupling with the transmission of tectonic stress on the upper plate over a long distance (Agard et al. Reference Agard, Omrani, Jolivet and Mouthereau2005; Vincent et al. Reference Vincent, Allen, Ismail-Zadeh, Flecker, Foland and Simmons2005; Allen & Armstrong, Reference Allen and Armstrong2008; Hatzfeld & Molnar, Reference Hatzfeld and Molnar2010). This resulted in the compressional deformation of the continental crust and folding, thrusting and mountain-building across the collision zone including the formation of the Alborz Mountains (Ballato et al. Reference Ballato, Uba, Landgraf, Strecker, Sudo, Stockli, Friedrich and Tabatabaei2011, Reference Ballato, Landgraf, Schildgena, Stockli, Fox, Ghassemie, Kirby and Strecker2015; Rezaeian et al. Reference Rezaeian, Carter, Hovius and Allen2012).
Thermochronologic and geochronologic data (Guest et al. Reference Guest, Axen, Lam and Hassanzadeh2006 a, b; Rezaeian et al. Reference Rezaeian, Carter, Hovius and Allen2012; Ballato et al. Reference Ballato, Stockli, Ghassemi, Landgraf, Strecker, Hassanzadeh, Friedrich and Tabatabaei2013, Reference Ballato, Landgraf, Schildgena, Stockli, Fox, Ghassemie, Kirby and Strecker2015; Madanipour et al. Reference Madanipour, Ehlers, Yassaghi, Rezaeian, Enkelmann and Bahroudi2013, Reference Madanipour, Ehlers, Yassaghi and Enkelmann2017, Reference Madanipour, Yassaghi, Ehlers and Enkelmann2018; Figs 1b, 8b), combined with data describing the Late Eocene and Miocene–Pliocene foreland and intermountain basins of the Alborz Mountains (Guest et al. Reference Guest, Horton, Axen, Hassanzadeh and McIntosh2007; Ballato et al. Reference Ballato, Nowaczyk, Landgraf, Strecker, Friedrich and Tabatabaei2008, Reference Ballato, Uba, Landgraf, Strecker, Sudo, Stockli, Friedrich and Tabatabaei2011), indicate that compressional deformation started shortly after the end of the extensional arc magmatism at the Eocene–Oligocene boundary,possibly related to the early stages of continental collision between the Arabia and Eurasia plates (Pirouz et al. Reference Pirouz, Avouac, Hassanzadeh, Kirschvink and Bahroudi2017). The regional deformation and exhumation of the Alborz Mountains continued during late Oligocene – early Miocene time in response to a more advanced stage of continental collision between the Arabia and Eurasia plates (e.g. Ballato et al. Reference Ballato, Uba, Landgraf, Strecker, Sudo, Stockli, Friedrich and Tabatabaei2011). It has been inferred that the decrease in plate convergence rate during middle Miocene time was associated with the final collision of upstretched Arabian lithosphere, which ultimately caused widespread upper-plate deformation (Ballato et al. Reference Ballato, Uba, Landgraf, Strecker, Sudo, Stockli, Friedrich and Tabatabaei2011; Madanipour et al. Reference Madanipour, Ehlers, Yassaghi and Enkelmann2017, Reference Madanipour, Yassaghi, Ehlers and Enkelmann2018). This has been attributed to a shift from soft collision (where the stretched continental crust of Arabia met Eurasia) to hard collision, where thickened crust of two continents converge (e.g. Ballato et al. Reference Ballato, Uba, Landgraf, Strecker, Sudo, Stockli, Friedrich and Tabatabaei2011; Madanipour et al. Reference Madanipour, Ehlers, Yassaghi and Enkelmann2017).
The on-going orogeny in the Alborz Mountain at that time is marked by the Miocene clastic deposits of the URF. The URF sediments were deposited within intra-mountain basins in the southern flank of the Alborz Mountains (and also in the MFMD) that largely covered pre-Neogene successions (Stocklin, Reference Stocklin1968; Berberian & King, Reference Berberian and King1981; Alavi, Reference Alavi1996; Ballato et al. Reference Ballato, Nowaczyk, Landgraf, Strecker, Friedrich and Tabatabaei2008; Rezaeian et al. Reference Rezaeian, Carter, Hovius and Allen2012). In the MFMD, within the mountain-building zone of the middle–upper Mesozoic proto-Alborz high between the North Alborz and the Firuzkuh faults, Miocene clastic sediments of the URF are exposed sporadically and overlain unconformably by pre-Tertiary strata (Fig. 3a). This angular unconformity suggests there was long-lived vertical motion of the proto-Alborz (also including MFMD) from late Oligocene time along this fault system. It also suggests that erosion of the uplifted rocks was the source of the Miocene clastic sediments in adjacent basins. The Oligo-Miocene FHe age detected in the KamarProsht deposit is therefore interpreted as a cooling age related to the initial Arabia–Eurasia collision.
This begs the question: why is the middle Miocene pulse of deformation and uplift not recorded in the MFDM? This might be controlled by the structural architecture of the area where strike-slip faulting was dominant, at least during late Miocene time. New palaeomagnetic analysis suggest pre-late Miocene (> c. 7 Ma) straight E–W-trending chains throughout the Alborz Mountains (Mattei et al. Reference Mattei, Cifelli, Muttoni and Rashid2015). The oroclinal bending of the Alborz during late Miocene time (c. 7 Ma) has led to a change in structural trend of the eastern Alborz from an approximately E–W- to NE–SW-aligned trend. This rotation may have led to a kinematic change from a dominant process of thrusting to strike-slip faulting, which could have reduced the amount of post-late Miocene exhumation in the eastern Alborz Mountains.
6. Conclusion
This study presents the results of fluorite (U–Th)/He geo-thermochronology to reveal its dual utility in dating both ore mineralization and exhumation events at ore deposits formed under low-temperature hydrothermal conditions (i.e. 90–150°C) and hosted by rocks lacking common datable minerals (i.e. carbonate strata). Our FHe ages range between c. 85 Ma, close to the known age for fluorite mineralization (c. 90 Ma by the Sm/Nd method), and c. 20 Ma. This range mostly covers the previously published AHe and ZHe Tertiary exhumation cooling ages in the Alborz Mountains. The oldest FHe ages (c. 85, 79 and 72 Ma) are interpreted to be those of fluorite mineralization in the MFMD and confirm the epigenetic origin of the fluorite deposits hosted by Middle Triassic and Lower Cretaceous carbonate rocks. We suggest the fluorite deposits formed by hydrothermal circulation of a basinal brine through potential source rocks that provided providing the ore-forming elements (i.e. F, Ba and Pb). Our mostly scattered FHe ages between 55 and 34 Ma record an important cooling event after Late Cretaceous mineralization in the MFMD. This coincides with the regional extensional tectonic regime and related post-magmatic thermal relaxation during the Eocene Epoch. Fast cooling of the heated fluorite mining blocks after geothermal gradient re-equilibration in the study area, or exhumation cooling during extensional tectonics and normal faulting characterized by a lower amount of erosion, are the most probable scenarios for Eocene cooling ages in this study.
A late Oligocene – early Miocene cooling event (c. 27–20 Ma) recorded in the samples near the Firuzkuk fault zone is possibly related to the initial Arabia–Eurasia collision. This event reactivated major structural features throughout the Alborz Mountains in the northern margin of the collision zone. The overall consistency between the FHe ages yielded in this study and previously published Cenozoic AHe, ZHe and AFT ages confirm the utility of the low-temperature fluorite-based (U–Th)/He chronology in orogenic belts bearing carbonate-hosted ore deposits for dating both ore mineralization and exhumation events.
Acknowledgements
This paper results from research project no. 951311 of the Research Council of Golestan University, Gorgan, Iran, for which the first author received financial support. Editor Professor O. Lacombe and two reviewers P. Ballato and R. Wolff are gratefully acknowledged for their critical review, constructive comments and helpful suggestions that helped to significantly improve the early version of the manuscript. Many thanks to Sarah Gleeson for editing and polishing the English language of the manuscript.
Conflict of interest
None.