Introduction
Understanding the requirement for the appearance of life on Earth is an essential step for searching for it elsewhere, given that terrestrial life is the only scientific source matter available. Key requisites have been identified in the presence of liquid water, of biologically essential elements (C, H, N, O, P, S) and of an energy source (Cottin et al., Reference Cottin, Kotler, Bartik, Cleaves, Cockell, de Vera, Ehrenfreund, Leuko, Ten Kate, Martins, Pascal, Quinn, Rettberg and Westall2017a).
The search for life beyond Earth needs to define what makes a planet habitable. A definition of habitability was defined as the ability of an environment to support the activity of at least one known organism (Cockell et al., Reference Cockell, Bush, Bryce, Direito, Fox-Powell, Harrison, Lammer, Landenmark, Martin-Torres, Nicholson, Noack, O'Malley-James, Payler, Rushby, Samuels, Schwendner, Wadsworth and Zorzano2016). A definition of habitability is as given for exoplanets that implies a distance from the star at which liquid water can exist on the planet surface. For the Solar System in addition to surface habitability, a sub-surface one refers to celestial bodies with liquid water only in their interior, such as Europa and Enceladus, two icy moons of Jupiter and Saturn, both being of great astrobiological interest (Horneck et al., Reference Horneck, Walter, Westall, Grenfell, Martin, Gomez, Leuko, Lee, Onofri, Tsiganis, Saladino, Pilat-Lohinger, Palomba, Harrison, Rull, Muller, Strazzulla, Brucato, Rettberg and Capria2016; Deamer and Damer, Reference Deamer and Damer2017).
The boundaries of habitability are being reshaped by our knowledge of the physical–chemical limits that life can withstand on Earth (Harrison et al., Reference Harrison, Gheeraert, Tsigelnitskiy and Cockell2013). Hints were given by the occurrence of microorganisms, called extremophiles, thriving in hostile environments such as hot and cold deserts considered as planetary field analogues for Mars or the Antarctic's sub-glacial lakes considered an analogue for the icy moons of Jupiter and Saturn (Martins et al., Reference Martins, Cottin, Kotler, Carrasco, Cockell, de la Torre Noetzel, Demets, de Vera, d'Hendecourt, Ehrenfreund, Elsaesser, Foing, Onofri, Quinn, Rabbow, Rettberg, Ricco, Slenzka, Stalport, ten Kate, van Loon and Westall2017). New insights into what makes a planet habitable are provided by experiments in which extremophiles are challenged with exposure to laboratory simulations that mimic planetary and deep space conditions.
Desert cyanobacteria as model system for astrobiology
In nature cyanobacteria belonging to the genus Chroococcidiopsis thrive in extreme environments such as the Dry Valleys in Antarctica or hot deserts worldwide, where they colonize porous rocks forming endolithic communities (Fig. 1(a)), or the soil–rock interface, forming hypolithic communities (Fig. 1(b)), where they were first discovereed (Friedmann et al., Reference Friedmann, Lipkin and Ocampo-Paus1967; Friedmann and Ocampo, Reference Friedmann and Ocampo-Friedmann1976).

Fig. 1. Examples of endolithic (a) and hypolithic growth of Chroococcidiopis in rocks collected in the Negev Desert by E. Imre Friedann and Roseli-Ocampo Friedmann.
Under laboratory conditions, they tolerate at least 4 years of air-drying (Billi, Reference Billi2009; Fagliarone et al., Reference Fagliarone, Mosca, Ubaldi, Verseux, Baqué, Wilmotte and Billi2017), up to 13 kJ m−2 of UVC irradiation (Baqué et al., Reference Baqué, Viaggiu, Scalzi and Billi2013a), 15 kGy of X-rays (Billi et al., Reference Billi, Friedmann, Hofer, Grilli Caiola and Ocampo-Friedmann2000) and 12 kGy of γ-radiation (Verseux et al., Reference Verseux, Baqué, Cifariello, Fagliarone, Raguse, Moeller and Billi2017). Remarkably when irradiated in the dried state, these extremophiles exhibit an enhanced radiation resistance, being able to cope with 30 kJ m−2 of a simulated Martian UV flux (Cockell et al., Reference Cockell, Schuerger, Billi, Friedmann and Panitz2005), 24 kGy of γ-radiation and 2 kGy of Fe ions (Verseux et al., Reference Verseux, Baqué, Cifariello, Fagliarone, Raguse, Moeller and Billi2017).
Such impressive desiccation and radiation tolerance of desert isolates of Chroococcidiopsis is relevant for a least two reasons: They can cope with both space and Martian simulated conditions in low Earth orbit (LEO), and second, they can be used in the current available space exposure facilities (Billi, Reference Billi, Stan-Lotter and Fendrihan2012a; Billi et al., Reference Billi, Baqué, Verseux, Rothschild, de Vera, Stan-Lotter and Fendrihan2017). In fact, in space and on the Martian surface, organisms must cope with extremely hostile conditions, such as intense radiation of cosmic and solar origin (Horneck et al., Reference Horneck, Klaus and Mancinelli2010). The use of desiccation-tolerant extremophiles is also necessitated by the fact that the space platforms currently used allow exposure of biological samples in the dried state, post flight analysis being carried after their return to Earth (Cottin et al., Reference Cottin, Kotler, Billi, Cockell, Demets, Ehrenfreund, Elsaesser, d'Hendecourt, van Loon, Martins, Onofri, Quinn, Rabbow, Rettberg, Ricco, Slenzka, de la Torre, de Vera, Westall, Carrasco, Fresneau, Kawaguchi, Kebukawa, Nguyen, Poch, Saiagh, Stalport, Yamagishi, Yano and Klamm2017b). In the past few years, astrobiology experiments have been performed by using the European Space Agency (ESA) EXPOSE facility that is installed outside the International Space Station (ISS) and that allows long-term exposure (up to 2 years) in LEO (Fig. 2(a)). Previously astrobiology experiments were carried out using the BIOPAN, an ESA scientific payload fixed to the exterior of the Russian Earth-orbiting FOTON satellite, and allowing short-term space exposure (Fig. 2(b)).
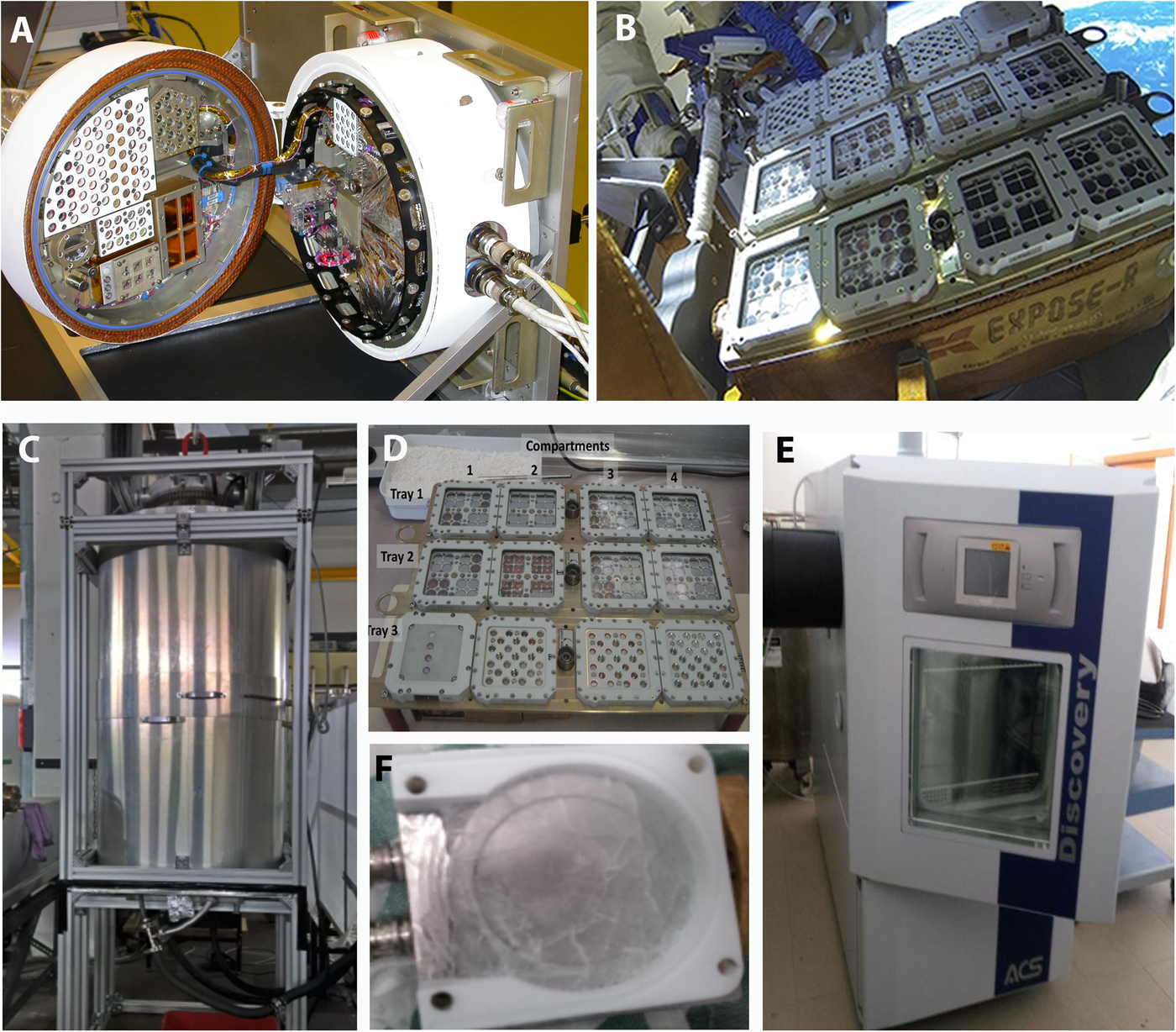
Fig. 2. Facilities for experiments carried out in low Earth orbit (a–b) or in ground-based space and planetary simulations (c–f). ESA Biopan facility fixed to the exterior of a Russian FOTON retrievable satellite (a) credits: Roscosmos, ESA/Kayser-Threde. ESA EXPOSE facility installed outside the International Space Station (b) credits: Roscosmos, ESA/Kayser-Threde. Mars atmosphere and pressure simulation at the Planetary and Space Simulation Facilities at DLR Cologne (c) and EXPOSE-R2 flight hardware with integrated biological samples (d) credits: DLR CC-BY 3.0. Climatic chamber for icy-moon laboratory simulations (e) and laboratory-grown ice simulating salt/ice mixtures mimicking Europa's icy crust (f) credits: Elena Pettinelli and Barbara Cosciotti, Department of Mathematics and Physics, Roma Tre University.
To date extremophiles able to survive the complete water removal when dried in the vegetative forms, the so-called anhydrobiotes, have been exposed to space or Martian-simulated conditions in space (Cottin et al., Reference Cottin, Kotler, Billi, Cockell, Demets, Ehrenfreund, Elsaesser, d'Hendecourt, van Loon, Martins, Onofri, Quinn, Rabbow, Rettberg, Ricco, Slenzka, de la Torre, de Vera, Westall, Carrasco, Fresneau, Kawaguchi, Kebukawa, Nguyen, Poch, Saiagh, Stalport, Yamagishi, Yano and Klamm2017b). Upon drying, anhydrobiotes enter a dormant state and resume an active metabolism upon rehydration: This is a requirement for tackling the vacuum of space. In the dried state, anhydrobiotes exhibit an enhanced resistance to both UV and ionizing radiations: This is a requirement for coping with non-Earth radiation environments. During the latest astrobiology experiments performed during the EXPOSE-R2 space mission (2014–2016) the total accumulated dose was of about 409.8 mGy (Dachev et al., Reference Dachev, Bankov, Tomov, Matviichuk, Dimitrov, Häder and Horneck2017), while the UV (200–400 nm) radiation varied according to the position of the trays inside the EXPOSE facility and ranged from 458 to 731 MJ m−2, although attenuated by the presence of 0.1% neutral density (ND) filter (Rabbow et al., Reference Rabbow, Rettberg, Parpart, Panitz, Schulte, Molter, Jaramillo, Demets, Weiß and Willnecker2017).
This suggests that the ionizing dose adsorbed over more than 1 year in LEO does not limit the capability of dried Chroococcidiopsis cells, once back to Earth, to reactivate their metabolism upon rehydration and repair accumulated damage. In addition, taking into consideration the dose rate of 76 mGy year−1 reported by the NASA's Mars Science Laboratory rover on the Martian surface at Gale Crater (Hassler et al., Reference Hassler, Zeitlin, Wimmer-Schweingruber, Ehresmann, Rafkin, Eigenbrode, Brinza, Weigle, Böttcher, Böhm, Burmeister, Guo, Köhler, Martin, Reitz, Cucinotta, Kim, Grinspoon, Bullock, Posner, Gómez-Elvira, Vasavada and Grotzinger2013), it might be speculated that dried Chroococcidiopsis could cope with a radiation environment equivalent to 200 000 years on the Martian surface (Billi et al., Reference Billi, Baqué, Verseux, Rothschild, de Vera, Stan-Lotter and Fendrihan2017). Indeed, concerning radiation, for extremophiles the limiting factor in space or on the Martian surface is UV radiation rather than ionizing radiation, and shielding against UV radiation provided by top-cell layers in bacterial multilayers or by clay or meteorite powder is enough to guarantee extended periods of survival (Horneck et al., Reference Horneck, Klaus and Mancinelli2010).
Desert cyanobacteria under space and planetary laboratory simulations
Exposure to space and planetary laboratory simulations was performed to select the most suitable extremophiles and test the experimental set up before the space mission. Laboratory simulations are carried out at the Planetary and Space Simulation Facilities (DLR-Institute of Aerospace Medicine in Köln), known as Experiment Verification Tests (EVTs) and Scientific Verification Tests (SVTs). Mission Ground Reference (MGR) experiments were realized as parallel to the space mission by using the PSI facilities to simulate the environmental parameters recorded during a given space mission and using the same flight hardware (Fig. 2(c) and (d)).
EVTs and SVTs were conducted for the EXPOSE-R2 space mission, in the contest of two ESA experiments: Biolfilm Organisms Surfing Space (BOSS) and BIOMEX (BIOlogy and Mars EXperiment). In BOSS, the hypothesis is tested if biofilm-forming bacteria are more resistant to space and Martian conditions than their planktonic counterparts (Panitz et al., Reference Panitz, Rettberg, Rabbow, Bauermeister, Barczyk, Billi, Cockell, Flemming, Stan-Lotter and Venkateswaran2012). BIOMEX aims at investigating the survival of selected extremophiles and the stability/degradation of their biological components (pigments, cell wall components, etc.), when mixed with Martian and lunar mineral analogues (de Vera et al., Reference de Vera, Boettger, de al Torre, Sanchez, Grunow, Schmitz, Lange, Hübers, Billi, Baque, Rettberg, Rabbow, Reitz, Berger, Möller, Bohmeier, Horneck, Westall, Jänchen, Fritz, Meyer, Onofri, Selbmann, Zucconi, Kozyrovska, Leya, Foing, Demets, Cockell, Bryce, Wagner, Serrano, Edwards, Joshi, Huwe, Ehrenfreund, Elsaesser, Ott, Meessen, Feyh, Szewzyk, Jaumann and Spohn2012). EVTs included the exposure to a Mars-like atmosphere (780 Pa of Mars-like atmosphere), space vacuum (2 × 10−4 Pa), temperature cycles (−10 + 45°C), temperature extremes (60°C), UVC254nm radiation up to 10 kJ m−2 and polychromatic UV (200–400 nm) radiation from 1.5 × 103 to 8 × 105 kJ m−2. SVTs were performed by exposing the samples to 5 × 105 kJ m−2 of polychromatic UV200–400nm, attenuated with 0.1% ND filter a dose expected after 1 year in LEO, under space (2 × 10−4 Pa) and Mars (780 Pa of Mars-like atmosphere) conditions.
Several pieces of evidence suggest that UV is the most limiting factor for microbial survival in LEO (Horneck et al., Reference Horneck, Klaus and Mancinelli2010); this is consistent with the results obtained for desert strains of Chroococcidiopsis exposed to space and Mars laboratory simulations.
When biofilms or planktonic samples made of dried, thick layers of Chroococcidiopsis cells (about 50 µm) were exposed to EVTs and SVTs biofilms, an enhanced performance of biofilms was reported, being able to cope with 5 × 102 kJ m−2 of UV polychromatic radiation (e.g. 500 MJ m−2 attenuated with 0.1% ND filter) combined with space vacuum or Martian atmosphere of 780 Pa.
(Baqué et al., Reference Baqué, de Vera, Rettberg and Billi2013b, Reference Baqué, Scalzi, Rabbow, Rettberg and Billi2013c); this confirms that bacteria in biofilms exhibit a set of properties that differ substantially from free-living bacteria, largely due to the presence of abundant extracellular exopolysaccharides (Flemming et al., Reference Flemming, Wingender, Szewzyk, Steinberg, Rice and Kjelleberg2016).
When EVTs and SVTs were performed on planktonic dried samples made of thin layers of Chroococcidiopsis cells (about 20 µm) mixed with Martian or Lunar mineral simulants, UV shielding due to minerals allowed survival and/or biomarkers detection. When damage accumulated during the simulations exceeded the repair capability of dried cells upon rehydration, potential biomarkers such as DNA, photosynthetic (chlorophyll a and phycobiliproteins) and photoprotective (β-carotene) pigments were still detectable. In the absence of minerals mixed with cells, DNA was undetectable after doses of polychromatic UV radiation ranging from 1.5 × 103 to 8 × 105 and 10 kJ m−2 of UVC (Baqué et al., Reference Baqué, Verseux, Rabbow, de Vera and Billi2014). DNA was still detectable in cells mixed with Lunar regolith simulant and exposed to 8 × 105 kJ m−2 (e.g. 800 MJ m−2; Baqué et al., Reference Baqué, Verseux, Rabbow, de Vera and Billi2014). Dried cells of Chroococcidiopsis retained their colony-forming ability after 10 kJ m−2 of UVC, whereas cells mixed with lunar mineral simulant formed colonies only after the lowest polychromatic UV dose tested, e.g. 1.5 × 103 kJ m−2, while colony-forming ability was lost after 5 × 102 kJ m−2 in combination with space vacuum (Baqué et al., Reference Baqué, Verseux, Rabbow, de Vera and Billi2014). DNA was also detectable in dried cells mixed with Martian regolith simulants after exposure to 5.7 × 102 kJ m−2 of polychromatic UV (e.g. 570 MJ m−2 with 0.1% ND filter) combined with a Mars-like atmosphere, although colony-forming ability was lost (Baqué et al., Reference Baqué, Verseux, Böttger, Rabbow, de Vera and Billi2016). On the other hand, overlain by 3 mm of Antarctic sandstone guaranteed the survival of dried Chroococcidiopsis cells exposed to 1.5 × 105 kJ m−2 of polychromatic UV radiation (Billi et al., Reference Billi, Viaggiu, Cockell, Rabbow, Horneck and Onofri2011).
The resistance of extremophiles to space radiation was further investigated in the frame of the STARLIFE (intercomparison study of astrobiological model systems in their response to major components of the galactic cosmic radiation) project, by using radiations mimicking single components of the cosmic radiation, e.g. accelerated heavy ions and high doses of X- and γ-rays (Moeller at al., Reference Moeller, Raguse, Leuko, Berger, Hellweg, Fujimori, Okayasu and Horneck2017). When dried cells of desert Chroococcidiopsis were irradiated with 1 kGy of He or Si ions, 2 kGy of Fe ions, 5 kGy of X-rays or with increasing doses of γ-rays up to 113 kGy, survival occurred after heavy ion and X-ray irradiation and up to 24 kGy of γ-rays (Verseux et al., Reference Verseux, Baqué, Cifariello, Fagliarone, Raguse, Moeller and Billi2017).
Desert strains of Chroococcidiopsis might represent suitable microorganisms in planetary ground-based simulations aimed at investigating the effects of radiation sources on photosynthesis, as expected for planets orbiting around M-dwarf stars (Claudi et al., Reference Claudi, Erculiani, Galletta, Billi, Pace, Schierano, Giro and D'Alessandro2016). A red shift in the photosynthetic pigment emission was detected in Chroococcidiopsis cells in hypolithic communities under quartz, carbonate and talc rock samples from the Mojave deserts (Smith et al., Reference Smith, Baqué, Duncan, McKay and Billi2014). Indeed the paradigm of photosynthesis occurring in the visible light is brought into question by the discovery of terrestrial cyanobacteria isolated from environments where they receive strongly filtered light, and able to use far-red light for oxygenic photosynthesis thanks to the capability of synthesizing novel chlorophylls (Ho et al., Reference Ho, Shen, Canniffe, Zhao and Bryant2016). Knowing the spectral features of photosynthetic pigments under non-Earth-simulated conditions might contribute to the search for spectroscopic surface biosignatures on exoplanets (Kaltenegger, Reference Kaltenegger2017; Schwieterman et al., Reference Schwieterman, Kiang, Parenteau, Harman, DasSarma, Fisher, Arney, Hartnett, Reinhard, Olson, Meadows, Cockell, Walker, Grenfell, Hegde, Rugheimer, Hu and Lyons2018).
Finally, ongoing experiments are investigating the capability of Chroococcidiopsis from the Dry Valleys in Antarctica to thrive in potentially habitable niches obtained by simulating liquid-water vein systems with a climatic chamber and using salt (or acid)/ice mixtures (Fig. 2(e) and (f)), as expected for the icy crusts of Europa (Pettinelli et al., Reference Pettinelli, Cosciotti, Di Paolo, Lauro, Mattei, Orosei and Vannaroni2015).
Desert cyanobacteria under space conditions and Martian conditions simulated in LEO
Even though the exposure to laboratory space and planetary analogues remains irreplaceable for astrobiology investigations, the complex combination of space conditions cannot be accurately simulated. Access to environmental conditions that encompass space vacuum, galactic cosmic rays and solar particle events is only possible through direct exposure in space (Cottin et al., Reference Cottin, Kotler, Billi, Cockell, Demets, Ehrenfreund, Elsaesser, d'Hendecourt, van Loon, Martins, Onofri, Quinn, Rabbow, Rettberg, Ricco, Slenzka, de la Torre, de Vera, Westall, Carrasco, Fresneau, Kawaguchi, Kebukawa, Nguyen, Poch, Saiagh, Stalport, Yamagishi, Yano and Klamm2017b).
Akinetes of the cyanobacterium Anabaena cylindrica were first exposed in LEO for 10 days during the BIOPAN 6 mission, and survivors occurred when not exposed to UV radiation (Olsson-Francis et al., Reference Olsson-Francis, de la Torre, Towner and Cockell2009). In the context of experiments aimed at testing the lithopanspermia theory, i.e. the interplanetary transport of microbial life form inside rocks (Nicholson, Reference Nicholson2009), Chroococcidiopsis cells were inoculated within rocks (at 5 mm depth) and installed on the exterior of the Foton satellite. Since no survivors were scored upon re-entry into the Earth atmosphere, it was hypothesized that the likelihood of transfer between planets for endolithic phototrophs might be less than for chemolithotrophic or heterotrophic bacteria (Cockell et al., Reference Cockell, Brack, Wynn-Williams, Baglioni, Brandstätter, Demets, Edwards, Gronstal, Kurat, Lee, Osinski, Pearce, Pillinger, Roten and Sancisi-Frey2007).
During the EXPOSE-E space mission (2008–2009) outside the ISS (Rabbow et al., Reference Rabbow, Rettberg, Barczyk, Bohmeier, Parpart, Panitz, Horneck, von Heise-Rotenburg, Hoppenbrouwers, Willnecker, Baglioni, Demets, Dettmann and Reitz2012), the survival of a desert strain of Chroococcidiopsis after a prolonged period (548 days) in space was reported (Cockell et al., Reference Cockell, Rettberg, Rabbow and Olsson-Francis2011). In the EXPOSE-R space mission (2009–2011), it was proved that impact-shocked gneiss-protected Chroococcidiopsis during 22 months of UV radiation exposure outside the ISS (Bryce et al., Reference Bryce, Horneck, Rabbow, Edwards and Cockell2015). Finally in the EXPOSE-R2 space mission (2014–2016), a selection of extremophiles, including desert strains of Chroococcidiopsis, was exposed to space conditions and simulated Mars-like environment (CO2 atmosphere and UV > 200 nm). This space mission started after EVTs and SVTs had been successfully performed, and extremophiles were exposed to space and Martian-simulated conditions outside the ISS for about 16 months, while MGR was performed by using a set of samples and flight hardware replicating those used during the space mission and reproducing the mission data (Rabbow et al., Reference Rabbow, Rettberg, Parpart, Panitz, Schulte, Molter, Jaramillo, Demets, Weiß and Willnecker2017).
During the EXPOSE-R2 mission, ample were exposed to about 0.5 Gy of ionizing radiation (Dachev et al., Reference Dachev, Bankov, Tomov, Matviichuk, Dimitrov, Häder and Horneck2017), while the mean polychromatic UV radiation fluence for trays 1 and 2 of the EXPOSE-R2 facility was about 458 and 492 MJ m−2, respectively, both attenuated with the 0.1% ND filter (Rabbow et al., Reference Rabbow, Rettberg, Parpart, Panitz, Schulte, Molter, Jaramillo, Demets, Weiß and Willnecker2017). Additional calculations provided by RedShift revealed that each sample was exposed to a different UV dose depending on its position. Hence Chroococcidiopsis samples part of the BIOMEX experiment were exposed to polychromatic (UV and visible light) radiation doses ranging from about 2 × 103 to 4 × 103 kJ m−2; while Chroococcidiopsis samples part of the BOSS experiment were exposed to polychromatic (UV and visible light) radiation doses ranging from 3 × 103 to 5 × 103 kJ m−2. Analysis of the EXPOSE-R2 space mission is ongoing. However based on the ground simulations, it can be anticipated that a desiccation- and radiation-tolerant life form like that investigated here could survive a few hours of exposure on the Martian surface, when it occurs as a dried thin-cell layers mixed with minerals or as a thick biofilm. These results have implications for the hypothesis that during Mars’ climatic history, desiccation-tolerant life forms could have survived for long periods in specific habitable niches and that opportunistic colonization of protected niches could have occurred on the condition that life forms remained viable during transportation (Westall et al., Reference Westall, Loizeau, Foucher, Bost, Betrand, Vago and Kminek2013).
Future astrobiology investigations beyond LEO, using platform allowing exposure experiments outside the proactive Earth magnetosphere and performed on metabolically active extremophiles, are being planned (Cottin et al., Reference Cottin, Kotler, Billi, Cockell, Demets, Ehrenfreund, Elsaesser, d'Hendecourt, van Loon, Martins, Onofri, Quinn, Rabbow, Rettberg, Ricco, Slenzka, de la Torre, de Vera, Westall, Carrasco, Fresneau, Kawaguchi, Kebukawa, Nguyen, Poch, Saiagh, Stalport, Yamagishi, Yano and Klamm2017b).
Desert cyanobacteria from astrobiology to synthetic biology and life support
Apart from contributing to the question on the limits of life as we know it, knowledge gathered from the exposure of desert strain of Chroococcidiopsis to space and Martian conditions makes these cyanobacteria promising phototrophs for developing biotechnologies beyond Earth. Humans’ exploration of deep space is only feasible if the crew is maintained with minimal/or no supply from Earth. Current biological life support systems (BLSS) technologies cannot sustain long-term planetary bases without re-supply from Earth; indeed the MELiSSA (Micro-Ecological Life Support System Alternative) project was designed based on higher plants and microorganisms, including bacteria and cyanobacteria, for producing oxygen and fixing carbon dioxide (through oxygenic photosynthesis), purify water (through plant transpiration) and possibly to provide food, starting from human waste (Hendrickx et al., Reference Hendrickx, De Wever, Hermans, Mastroleo, Morin, Wilmotte, Janssen and Mergeay2006).
The use of resources available on the planetary surfaces to produce oxygen, fuel and biomass production might help solve several constraints of the human space exploration. The use of extreme-tolerant cyanobacteria to link on-site resources to BLSS has been proposed (Billi et al., Reference Billi, Baqué, Smith and McKay2013, Reference Billi, Baqué, Verseux, Rothschild, de Vera, Stan-Lotter and Fendrihan2017; Verseux et al., Reference Verseux, Baqué, Lehto, de Vera, Rothschild and Billi2016a). The capability of certain cyanobacteria to release bio-essential elements from analogues of Martian rocks was already reported (Olsson-Francis and Cockell, Reference Olsson-Francis and Cockell2010). However, since the NASA Phoenix Lander's onboard Wet Chemistry Lab discovered the presence of perchlorate anions, at a concentration of 0.4–0.6 wt% (Hecht et al., Reference Hecht, Kounaves, Quinn, West, Young, Ming, Catling, Clark, Boynton, Hoffman, Deflores, Gospodinova, Kapit and Smith2009), which is toxic for most of the organisms, the effects of perchlorates on extreme-tolerant cyanobacteria must be taken into account. The fact that desert strains of Chroococcidiopsis can cope with high level of oxidative stress (Fagliarone et al., Reference Fagliarone, Mosca, Ubaldi, Verseux, Baqué, Wilmotte and Billi2017) suggests that it might cope with the presence of perchlorates in the growing medium, a feature relevant in the contest of their use to link BLSS to on-site resources. This feature, along with their capability to cope with high doses of UV and ionizing radiations, is a key prerequisite for future work aimed at identifying the minimal modifications of the Martian environment (gas composition and pressure, shielding, etc.) necessary for an efficient metabolism under non-Earth conditions.
An important requisite for desert strains of Chroococcidiopsis exploitation in space biotechnology relies in their capability to repair, upon rehydration under Earth conditions, the damage accumulated after a prolonged permanence in LEO or after exposure to extremely high doses of ionizing radiations. Nevertheless, the effects of altered microgravity and different irradiative environments on the repair processes must be taken into consideration. The reported endurance of these cyanobacteria under space conditions is also a prerequisite for their successful transfer to Mars, and for their possible use in the cis-Lunar module and/or as a back-up stock maintained in the dried state for prolonged periods.
On Earth approaches based on microorganism-based biotechnology are used to obtain food and drinks from edible microorganisms and fermented products (e.g., wine and yoghurt), drugs, various chemicals, biomaterials, biofuels and so on (Verseux et al., Reference Verseux, Paulino-Lima, Baqué, Billi, Rothschild, Hagen, Engelhard and Toepfer2016b). Thanks to engineering-driven approaches of modularization, rationalization and modelling, the emerging discipline of synthetic biology has been generating a variety of genetic devices and biological modules, with applications in a wide range of fields (Khalil and Collins, Reference Khalil and Collins2010).
In the context of long-term deep space expeditions and human settlement on Mars, synthetic biology could greatly decrease the cost of human space exploration by providing life support systems, by producing consumables on site and by synthetizing, for instance, structural materials (Rothschild, Reference Rothschild2016). Indeed the finding of a fast-growing perchlorate-resistant halophile is of great interest since it could be used as a new ‘chassis’ organism to be used for synthetic biology applications on Mars (Matsubara et al., Reference Matsubara, Fujishima, Saltikov, Nakamura and Rothschild2017). However, the availability of a robust chassis for synthetic biology under extreme conditions is essential. This requirement could be met by desert strains of Chroococcidiopsis that avoid oxidative damage to proteins after prolonged desiccation, oxygen peroxide treatment and ionizing radiation (Fagliarone et al., Reference Fagliarone, Mosca, Ubaldi, Verseux, Baqué, Wilmotte and Billi2017). The use of these cyanobacterial strains in space synthetic biology is rendered even more viable by the availability of whether a genetic system for their genetic manipulation (Billi et al., Reference Billi, Friedmann, Helm and Potts2001; Billi, Reference Billi and Méndez-Vilas2010) or their capability to maintain exogenous plasmid DNA after prolonged dried storage (Billi, Reference Billi2012b). In the foreseeable future to link BLSS to on-site resources by using these cyanobacteria, synthetic biology could serve three main purposes: (i) by increasing their resistance to conditions found beyond Earth, (ii) by enhancing their ability to use resources available on site and (iii) by endowing them with new abilities, e.g., the ability to produce useful compounds which they do not produce naturally (Verseux et al., Reference Verseux, Paulino-Lima, Baqué, Billi, Rothschild, Hagen, Engelhard and Toepfer2016b).
Acknowledgements
This work was supported by the Italian Space Agency (ASI 2013-053-R.0; ASI 2013-051-R.0) and the National Antarctic Research Program (PNRA2016/00101; PNRA2013/AZ1.17).