1. Introduction
Of the two types of orogens – collisional (or continent–continent collision) and accretionary (or subduction–accretion) – accretionary is the least understood (Windley, Reference Windley and Condie1992; Şengör et al. Reference Şengör, Natal’in and Burtman1993; Condie, Reference Condie, Hatcher, Carlson, McBride and Martínez Catalán2007; Cawood et al. Reference Cawood, Kröner, Collins, Kusky, Mooney, Windley, Cawood and Kröner2009; Xiao et al. Reference Xiao, Han, Yuan, Sun, Zhao and Shan2010 a, b). Accretionary orogens form at the sites of the subduction of oceanic lithosphere against a cratonic backstop that has not been through a Wilson cycle of events, and which will not terminate until final collision with an incoming continent. The prime extant example of an accretionary orogen is the Japanese archipelago (500 Ma to present), on which our current understanding of this type of orogenesis is founded. The synthesis–culmination publications of Isozaki (Reference Isozaki1996) and Maruyama (Reference Maruyama1997) were based on more than a decade of earlier research by many Japanese geologists. Another example is the Central Asian Orogenic Belt (CAOB; 1.1 Ga to 250 Ma) (Jahn et al. Reference Jahn, Wu and Chen2000; Windley et al. Reference Windley, Alexeiev, Xiao, Kröner and Badarch2007; Safonova et al. Reference Safonova, Seltmann, Kröner, Gladkochub, Kim, Schulmann, Xiao, Komiya and Sun2011; Wakita et al. Reference Wakita, Pubellier and Windley2013), which occupies the bulk of eastern–central Asia (Schulmann & Paterson, Reference Schulmann and Paterson2011), and to which the Beishan collage belongs (Xiao et al. Reference Xiao, Windley, Badarch, Sun, Li, Qin and Wang2004, Reference Xiao, Windley, Yuan, Sun, Han, Lin, Chen, Yan, Liu, Qin, Li and Sun2009, Reference Xiao, Mao, Windley, Han, Qu, Zhang, Ao, Guo, Cleven, Lin, Shan and Li2010 c; Tian et al. Reference Tian, Xiao, Windley, Zhang, Zhang and Song2016). The southern part of the CAOB is widely referred to as the Altaids (Şengör et al. Reference Şengör, Natal’in and Burtman1993, Reference Şengör, Natal’in, Sunal and Voo2014; Wilhem et al. Reference Wilhem, Windley and Stampfli2012). The CAOB is made up of accreted prisms, mélanges, island arcs with fore-arcs and back-arcs, ophiolitic remnants that are different from the Penrose-type ophiolites in the Mediterranean (Anonymous, 1972), subducted mid-oceanic ridges and slab windows (Windley & Xiao, Reference Windley and Xiao2018), oceanic plateaus, major oroclines (Xiao et al. Reference Xiao, Windley, Sun, Li, Huang, Han, Yuan, Sun and Chen2015), ocean plate stratigraphy (Kusky et al. Reference Kusky, Windley, Safonova, Wakita, Wakabayashi, Polat and Santosh2013; Safonova & Santosh, Reference Safonova and Santosh2014) and old continental blocks. Palaeomagnetic and palaeogeographic relations show that the southern margin of the Siberian Plate was surrounded by the Palaeo-Asian Ocean during Neoproterozoic–Palaeozoic time (Fig. 1a; Wan & Zhu, Reference Wan and Zhu2011). Subduction of this ocean led to formation of the CAOB and the Beishan collage, and the terminal collision with the North China Craton.
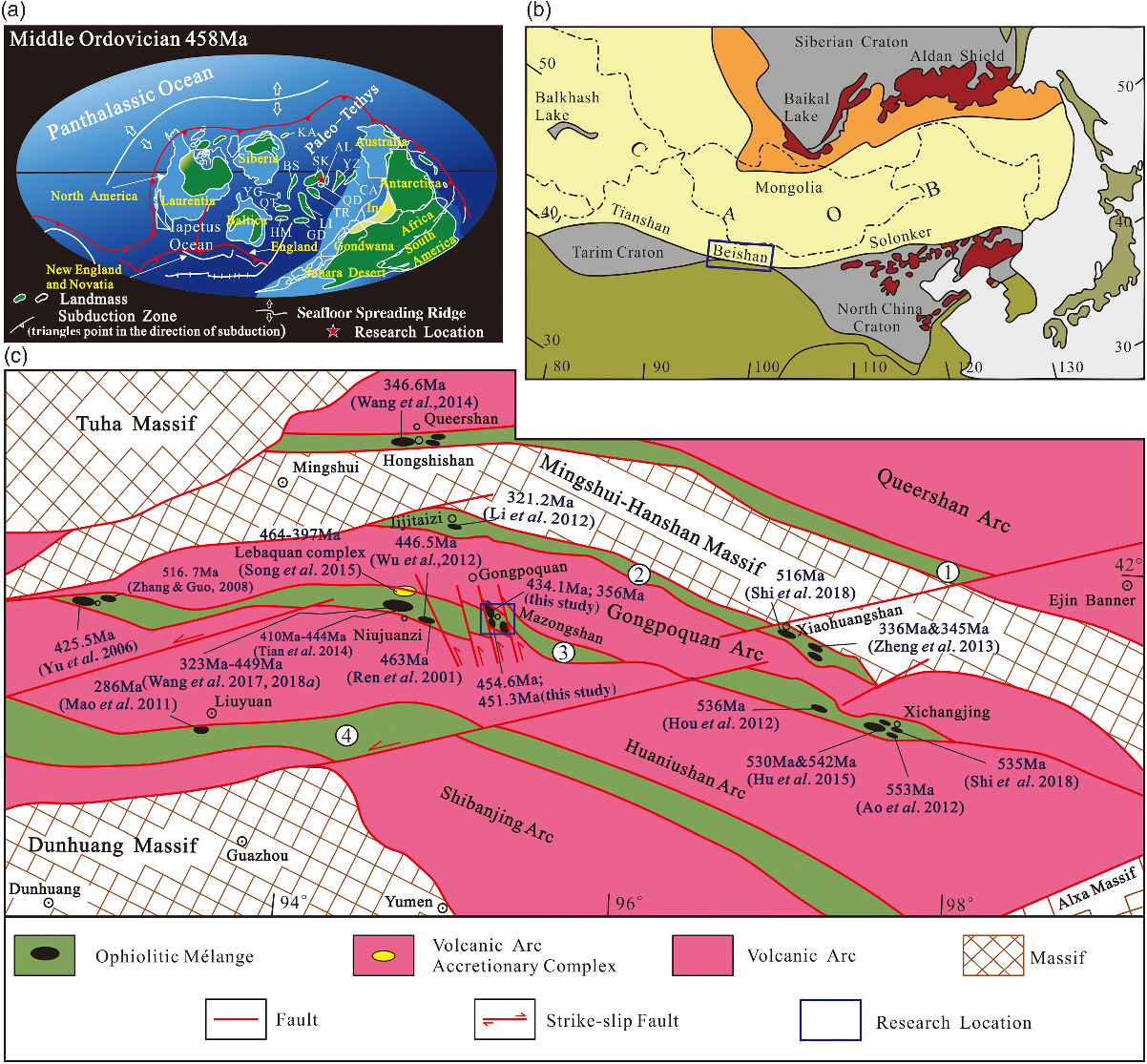
Fig. 1. (a) Palaeocontinental reconstruction of the globe during the Middle Ordovician Epoch (458 Ma), showing the distribution for the Middle Ordovician ocean and continent (after Wan & Zhu, Reference Wan and Zhu2011). AL – Alxa–Dunhuang; BS – Baoshan–Sibunasu; CA – Cathaysian; GD – Gangdise; HM – Himalayan; JG – Juggar; KD – East Kunln; KZ – Kazahkstan; LI – Lincang–Indochina; QD – Qaidam; QT – Qiangtang; SK – Sino-Korean; TR – Tarim; YG – Yagen; YZ – Yangtze. (b) Location of the study area in the southern Altaid orogen (after Xiao et al. Reference Xiao, Windley, Hao and Zhai2003; Guo et al. Reference Guo, Xiao, Hou, Windley, Han, Tian and Song2014). (c) Diagram showing the boundaries of tectonic units in the Beishan accretionary collage (modified by Wang et al. Reference Wang, Zhang, Song, Li, Li and Zhou2017): 1, Hongshishan Ophiolitic mélange; 2, Jijitai Ophiolitic mélange; 3, Honliuhe–Niujuanzi–Mazongshan–Xichangjing ophiolitic mélange; 4, Liuyuan ophiolitic mélange (Zhang & Guo, Reference Zhang and Guo2008; Ren et al. Reference Ren, He, Yao and Fu2001; Yu et al. Reference Yu, Li and Wang2006; Mao et al. Reference Mao, Xiao, Windley, Han, Qu, Ao, Zhang and Guo2011; Ao et al. Reference Ao, Xiao, Han, Li, Qu, Zhang, Guo and Tian2012; Hou et al. Reference Hou, Wang, Liu, Wang and Li2012; Li et al. Reference Li, Yu, Wang and Wu2012; Wu et al. Reference Wu, Wang, Li, Yu and Kang2012; Wang et al. Reference Wang, Li, Xu and Wu2014, Reference Wang, Zhang, Song, Li, Li and Zhou2017, Reference Wang, Zhang, Song, Li, Li, Yu and Bu2018 a; Zheng et al. Reference Zheng, Wu, Zhang, Xu and Meng2013; Tian et al. Reference Tian, Xiao, Windley, Lin, Han, Zhang, Wan, Ao, Song and Feng2014; Hu et al. Reference Hu, Zhao, Hu, Liao and Cheng2015; Song et al. Reference Song, Xiao, Windley, Han and Tian2015; Shi et al. Reference Shi, Zhang, Kröner, Li and Jian2018).
The Beishan accretionary collage, which connects the Tianshan suture to the west with the Solonker suture to the east (Fig. 1b; Xiao et al. Reference Xiao, Windley, Hao and Zhai2003), is an important component of the CAOB (Xiao et al. Reference Xiao, Mao, Windley, Han, Qu, Zhang, Ao, Guo, Cleven, Lin, Shan and Li2010 c; Zhang et al. Reference Zhang, Pan, He, Xiao, Xu, Zhang, Lu, Deng, Feng, Li, Zhao, Xing, Wang, Yin, Hao, Zhang, Zhang and Gong2015, Reference Zhang, He, Xu, Luo, Song, Kou, Zhang, Xiao and Pan2016, Reference Zhang, He, Xu, Luo, Song, Kou and Xing2018). It underwent a complex geotectonic evolution with multiple stages of subduction and accretion, but there is still much debate about its style and type of evolution: in particular, the timing of the conversions of oceanic and continental components, and of terminal collision. For example, did the CAOB accretionary orogen terminate during Devonian or Carboniferous time, and was it followed by continental rifting in during Carboniferous or Permian time (Wang et al. Reference Wang, Zhang, Song, Li, Li and Zhou2017, Reference Wang, Zhang, Song, Li, Li, Yu and Bu2018 a) or Permian time (Xiao et al. Reference Xiao, Windley, Hao and Zhai2003, Reference Xiao, Windley, Badarch, Sun, Li, Qin and Wang2004, Reference Xiao, Mao, Windley, Han, Qu, Zhang, Ao, Guo, Cleven, Lin, Shan and Li2010 c; Windley et al. Reference Windley, Alexeiev, Xiao, Kröner and Badarch2007)? In particular, a lack of adequate research has led to uncertainties about the structure, tectonic setting and subduction polarity of the main components in central Beishan, and this has prevented a full understanding of the entire Beishan collage (Ao et al. Reference Ao, Xiao, Han, Li, Qu, Zhang, Guo and Tian2012, Reference Ao, Xiao, Windley, Mao, Han, Zhang, Yang and Geng2016; Zheng et al. Reference Zheng, Wu, Zhang, Xu and Meng2013; Guo et al. Reference Guo, Xiao, Hou, Windley, Han, Tian and Song2014; Song et al. Reference Song, Xiao, Han, Li, Qu, Guo, Lin and Wang2013 a, b, c, Reference Song, Xiao, Han and Tian2014, Reference Song, Xiao, Windley, Han and Tian2015; Wang et al. Reference Wang, Zhang, Song, Li, Li and Zhou2017, 2018; Shi et al. Reference Shi, Zhang, Kröner, Li and Jian2018).
The centre of the Beishan accretionary collage is occupied by the Mazongshan tectonic mélange and subduction–accretion complex (Fig. 2), which is sandwiched between the Liuyuan passive continental margin or fore-arc and the Gongpoquan magmatic arc (Fig. 1c). Our aim in this paper is to present new detailed information about the structural relations and geochemical–isotopic data of the main components of the Mazongshan complex in order to constrain the tectonic setting, time of formation and accretionary history of the Beishan collage.

Fig. 2. Geological map of the research location (modified from the Gangu Survey Institute, 2008).
2. Geological background
The Beishan collage is a composite accretionary complex that is composed of fragments of island arcs, oceanic crustal debris, trench slope turbidites, and pelagic and fore-arc sediments (Xiao et al. Reference Xiao, Mao, Windley, Han, Qu, Zhang, Ao, Guo, Cleven, Lin, Shan and Li2010 c; Mao et al. Reference Mao, Xiao, Windley, Han, Qu, Ao, Zhang and Guo2011; He et al. Reference He, Zhang, Zong, Xiang, Chen and Xia2014 a, b; Song et al. Reference Song, Xiao, Windley, Han and Tian2015; Tian et al. Reference Tian, Xiao, Windley, Zhang, Zhang and Song2016; Wang et al. Reference Wang, Zhang, Song, Li, Li and Zhou2017). From south to north the Beishan collage is made up of the Dunhuang Massif, the Shibanjing arc, the Liuyuan ophiolitic mélange, the Huaniushan arc, the Hongliuhe-Niujuanzi-Mazongshan-Xichangjing ophiolitic mélange, the Gongpoquan magmatic arc, the Jijitaizi-Xiaohuangshan ophiolitic mélange, the Mingshui-Hanshan continental massif, the Hongshishan ophiolitic mélange, and the Queershan arc (Fig. 1c) (Xiao et al. Reference Xiao, Mao, Windley, Han, Qu, Zhang, Ao, Guo, Cleven, Lin, Shan and Li2010 c; Mao et al. Reference Mao, Xiao, Windley, Han, Qu, Ao, Zhang and Guo2011; Zhang et al. Reference Zhang, Feng, Song, Zhang, Wang, Pan, Lu, Zhao and Xing2014 a, b, Reference Zhang, He, Xu, Luo, Song, Kou, Zhang, Xiao and Pan2016, Reference Zheng, Xiao, Li, Wu and Zhang2018; Wang et al. Reference Wang, Zhang, Song, Li, Li and Zhou2017).
Stratas in the Beishan collage include Proterozoic, Palaeozoic, Mesozoic and Cenozoi. In southern Mazongshan glaciers deposited a moraine conglomerate seen in the Sinian Xichangjing Group, which contains limestone dropstones up to c. 3–8 cm across. Associated moraine gravels are mainly composed of limestone and siliceous dolomite, which suggests that the glacier travelled across a passive continental margin (Chen et al. Reference Chen, Xiu, Pan, Zhang, Zhang, Li and Zhuan2017). The deposition was likely in a continental glacial environment, indicating that an ancient continent existed in the Beishan area during Neoproterozoic time. The lower Cambrian Shuangyingshan Formation contains bioclastic limestones and thin-bedded marbles indicative of a shallow-marine or coastal-sea environment. The Middle–Upper Cambrian Xishuangyingshan Formation is mainly composed of blue-grey pelagic cherts with thin interbeds of limestone, which may have been related to a contemporary mid-ocean-ridge basalt (MORB-) type of ophiolite in central Beishan (Shi et al. Reference Shi, Zhang, Kröner, Li and Jian2018).
The Hongliuhe–Niujuanzi–Mazongshan–Xichangjing (HNMX) ophiolitic mélange, situated in central parts of the Beishan collage, is mainly composed of Cambrian–Silurian clastic and pyroclastic rocks, many tectonic slices of ophiolitic rocks, and Carboniferous–Permian conglomerates, graywackes, tuffaceous siltstones, slates, cherts and minor limestones (Zuo et al. Reference Zuo, Liu and Liu2003; Xiao et al. Reference Xiao, Han, Liu, Wan, Zhang, Ao, Zhang, Song, Tian and Luo2014; Wang et al. Reference Wang, Zhang, Song, Li, Li and Zhou2017, Reference Wang, Zhang, Song, Li, Li, Yu and Bu2018 a). All these rocks are mutually juxtaposed by thrusts. From west to east along this ophiolitic mélange, there are major fault-bounded bodies of ophiolite in the Hongliuhe, Niujuanzi and Xichangjing areas. There are much geochemical data and high-resolution geochronology of these ophiolitic mélanges (Fig. 1c; Ren et al. Reference Ren, He, Yao and Fu2001; Yu et al. Reference Yu, Li and Wang2006; Zhang & Guo, Reference Zhang and Guo2008; Ao et al. Reference Ao, Xiao, Han, Li, Qu, Zhang, Guo and Tian2012; Hou et al. Reference Hou, Wang, Liu, Wang and Li2012; Tian et al. Reference Tian, Xiao, Windley, Lin, Han, Zhang, Wan, Ao, Song and Feng2014; Hu et al. Reference Hu, Zhao, Hu, Liao and Cheng2015; Wang et al. Reference Wang, Zhang, Song, Li, Li and Zhou2017; Shi et al. Reference Shi, Zhang, Kröner, Li and Jian2018), which have improved our understanding of their tectonic settings. Recently, it was reported that the youngest age from the matrix of a greywacke turbidite in the HNMX ophiolitic mélange has a Carboniferous U–Pb zircon age of 323 Ma (Wang et al. Reference Wang, Zhang, Song, Li, Li, Yu and Bu2018 a). Tian et al. (Reference Tian, Xiao, Windley, Zhang, Zhang and Song2016) reported that intrusive granodiorite was dated as Carboniferous with an age of 308.1 ± 3.8 Ma from the Pochenshan area from a post-orogenic continental rift. These ages mean that the ocean that gave rise to the HNMX ophiolitic mélange was in existence from the early Cambrian to the late Carboniferous period.
The Gongpoquan Arc to the north of the HNMX ophiolitic mélange comprises Ordovician–Silurian rhyolite, rhyolitic volcanic breccia, altered andesite, feldspathic sandstone, quartzite, schist and marble, containing Silurian conodonts such as Amarphgnathrs sp., A. amorphognthiform, A. holodontiform, A. tetraprioniodiform, Parathlgnathusobesus and Spathognathodusprimus (Zuo et al. Reference Zuo, Jin and Feng1994). Recently, more and more published isotopic age data indicate that the northern Gongpoquan arc formed during Ordovician – Middle Devonian time (Song et al. Reference Song, Xiao, Han and Tian2013 b, Reference Song, Xiao, Han and Tian2014, Reference Song, Xiao, Windley, Han and Tian2015; Wang et al. Reference Wang, Li, Xu, Yu, Guo, Yan, Ji and Wu2016, Reference Wang, Yuan, Zhang, Long, Sun, Wang, Soldner and Lin2018 b), and this arc was the product of the northwards subduction of the Palaeo-ocean (Ao et al. Reference Ao, Xiao, Han, Li, Qu, Zhang, Guo and Tian2012; Song et al. Reference Song, Xiao, Han and Tian2014, Reference Song, Xiao, Windley, Han and Tian2015). Further south, the Shuangyingshan–Huaniushan Arc developed to the south of the HNMX ophiolitic mélange. The subduction-related plutons in the Huaniushan Arc are Silurian – Late Devonian in age (373.8 ± 1.6 to 424.0 ± 1.3 Ma; Mao et al. Reference Mao, Xiao, Fang, Wang, Han, Sun and Yuan2012; Ding et al. Reference Ding, Han, Xiao, Wang and Yang2015). Xiao et al. (Reference Xiao, Mao, Windley, Han, Qu, Zhang, Ao, Guo, Cleven, Lin, Shan and Li2010 c) proposed that the Shuangyingshan–Huaniushan Arc developed during Ordovician–Carboniferous time.
The Mazongshan complex was earlier thought to be Precambrian–Cambrian in age because of its pervasive metamorphic and deformational fabrics (Gangu Survey Institute, 2001), but it was then found to be Ordovician in age and connected to the Lebaquan arc–fore-arc complex (Gangu Survey Institute, 2008). Today, the field relations, geochemical characteristics and tectonic setting of the Mazongshan complex are controversial: alternatives are an arc, continental margin rift, an arc–fore-arc or an ophiolitic mélange (Fig. 1c) (Gangu Survey Institute, 2001; Song et al. Reference Song, Xiao, Han, Li, Qu, Guo, Lin and Wang2013 a, b, Reference Song, Xiao, Han and Tian2014, Reference Song, Xiao, Windley, Han and Tian2015; Xiao et al. Reference Xiao, Han, Liu, Wan, Zhang, Ao, Zhang, Song, Tian and Luo2014).
3. Structure, lithology and petrography of the Mazongshan complex
3.a. Field relations
We recently carried out detailed field-based structural mapping and sample collections in central Beishan, mainly in the Jiabuquankouzi area and the associated Lebaquan complex. The Mazongshan complex, which is well exposed from the south of Jiabuquankouzi to the north of Qigejing (Fig. 2), comprises diverse blocks up to c. 120 m across of seamount sediments, serpentinized peridotite, gabbro, basalt, andesite and chert in a turbiditic slaty matrix (Fig. 3a–c, e). These variably deformed rocks are thrust-imbricated and strongly sheared. The many blocks behaved competently within the ductile, tightly folded, slaty matrix. The lithologies and structures of the Mazongshan complex are characteristic of a subduction–accretion mélange.

Fig. 3. Field photographs from the Mazongshan complex, central Beishan: (a, b, c, e) characteristic blocks in the matrix of the mélange; and (d) tight folds in the turbidite matrix.
The thickest P3 cross-section (Fig. 4) contains (from bottom to top): greenish amygdaloidal and vesicular basalts; black-green, poorly exposed, serpentinized peridotites (see the eastern side of the P3 cross-section); and cumulate layered and graded, but noticeably inverted, gabbros (on the western side of the P3 cross-section). Another type of basalt that is uniquely associated with marbles mainly crops out on the western side of the P3–P5 cross-section. These successions are more than 20 m thick, comprising lower grey basalts and upper reddish marbles (Fig. 4a, b).

Fig. 4. Cross-sections P1, P2 and P3, showing their detailed lithologies, structural patterns and sample locations in the Jiabuquankouzi area. See Figure 2 for legend.
The matrix of the mélange is mainly composed of tuffaceous and silty slates, which contain innumerable tectonic lenses and blocks of gabbro, basalt and serpentinized peridotite c. 0.5–150 m long. All have been broken into fragments by reverse faults.
The Lebaquan arc–fore-arc, which is tens of kilometres from the Mazongshan complex (Song et al. Reference Song, Xiao, Han and Tian2014, Reference Song, Xiao, Windley, Han and Tian2015), is mainly composed of metasedimentary quartzite, phyllite, chlorite–quartz schist, sericite–quartz schist, chert, marble and mafic volcanic rocks, plus gneissic granite plutons and later leucogranite–pegmatites and mafic dykes. Clearly, the Mazongshan complex is very different from the Lebaquan complex in terms of lithologies and structures.
3.b. Petrography
Ophiolitic rocks in the Mazongshan complex include serpentinized peridotite, gabbro and basalts, which are weakly altered. Serpentinized peridotite is generally composed of altered olivine (80% of rock volume), clinoenstatite (10%), tremolite, magnetite and spinel (Fig. 5a, b). Most basalts contain about 10% phenocrysts of plagioclase and ferromagnesian minerals within a 90% matrix of plagioclase, chlorite, calcite, magnetite and titanite (Fig. 5c, d).

Fig. 5. Photomicrographs of petrographic features of the Mazongshan complex, Beishan: (a, b) serpentinized peridotite; (c, d) metabasalt; (e, f) andesite; and (g, h) tuffaceous slate. Ol – olivine; Pl – plagioclase; Opx – clinoenstatite.
Gabbros are hypidiomorphic and consist of medium- to fine-grained plagioclase and pyroxene; some pyroxenes are partly to completely replaced by epidote, chlorite, hornblende and actinolite. Amygdaloidal andesite blocks (Fig. 5e, f) contain irregular or circular amygdales, consisting of plagioclase, chlorite, epidote, calcite, apatite, titanite and pyrite. The ‘matrix’ of turbidites is mostly tuffaceous slate and silty slate composed of quartz phenocrysts, some of which occurs in a foliated matrix. Tuffaceous slate matrix (Fig. 5g, h) shows a directional arrangement to some extent, containing irregular or circular quartz, feldspar and mica.
4. Sampling and analytical methods
Our representative samples from the mélange of the Mazongshan complex were collected from the least-altered and least-sheared parts of most of the tectonic blocks. The samples were crushed and pulverized to 200 mesh using an agate mortar in the State Key Laboratory of Geological Processes and Mineral Resources (GPMR), China University of Geosciences, Wuhan. A total of 20 samples were analysed for major and trace elements, including two gabbros (one from cross-section 1 and one from cross-section 2), 14 samples from cross-section 3 (that include 1 serpentinized peridotite, 3 andesites and 8 basalts), and a further 6 basalts from cross-sections 4 and 5 and line 3200. Four samples of the gabbros, andesites and tuffaceous slates were analysed for zircon U–Pb isotope analysis.
4.a. Major- and trace-element analyses
To constrain the tectonic setting of the Mazongshan complex, 1 serpentinized peridotite, 11 basalts, 1 gabbro and 7 andesite samples were collected from the blocks for whole-rock major-, trace- and rare-earth-element analyses. All the sample locations are highlighted in Figure 5.
Whole-rock major-, trace- and rare-earth-element analyses were performed at the Hubei Province Geological Experimental Testing Center. Major elements were measured by X-ray fluorescence spectrometry and plasma spectrometry with a Magix-pro2440 spectrometer, for which the analytical uncertainty was better than 5%. The trace elements were analysed with a Thermoelemental X7 inductively coupled plasma – mass spectrometer (ICP-MS) with analytical uncertainties of 5–10%. Methods of sample preparation, analysis and processing followed the procedures of Liu et al. (Reference Liu, Hu, Gao, Günther, Xu, Gao and Chen2008). Representative major- and trace-element analyses are listed in Table 1.
Table 1. Major and trace element composition for the Mazongshan complex

4.b. Zircon U–Pb isotope analysis
We attempted to collect fresh samples for dating and geochemical analyses from the Jiabuquankouzi area. Four samples (P1-3, P2-6, P3-117 and P3-27) of intermediate–basic rocks and slate were collected from the P1, P2 and P3 cross-sections (petrographic characteristics of sample P1-3, P3-117 and P3-27 are shown in Fig. 5a, b, e–h). Sample P1-3 and sample P2-6 are dark-green gabbros collected from the western side of the Jiabuquankouzi area. Sample P3-117 is yellowish-green andesite collected from the eastern side of the Jiabuquankouzi area. Sample P3-27 is light-grey tuffaceous slate collected from the central part of the Jiabuquankouzi area. These samples, which represent the typical lithology of the Mazongshan complex, constrain the evolution stages of the Mazongshan complex. All sample locations are highlighted in Figures 2 and 4.
Zircons were separated following the standard procedures of crushing and heavy liquid and magnetic techniques, and handpicked under a binocular microscope at the Langfang Credit Geological Service Co., Hebei Province. A total of 30 grains from each sample were picked randomly, enclosed in epoxy resin and polished to approximately half their thickness. The zircons were photographed under reflected and transmitted light, and their cathodoluminescence (CL) images were prepared at the State Key Laboratory of Geological Processes and Mineral Resources, China University of Geosciences, Wuhan, China. Zircon U–Pb dating was undertaken with an Agilent7500a ICP-MS instrument. Zircon91500 was used as an external standard for the U–Pb dating, and U, Th and Pb concentrations were calibrated using 29Si as an internal standard and NIST 610 as a reference material. The typical instrument operating conditions followed those reported in Liu et al. (Reference Liu, Hu, Gao, Günther, Xu, Gao and Chen2008). All data were acquired from the zircons in single spot ablation mode with a spot size of 32 μm. Every analysis from each sample incorporated one background acquisition lasting 20–30 s followed by 50 s of data acquisition. Offline selection and integration of background and analytical signals, time-drift corrections and quantitative calibrations for trace-element analyses and U–Pb dating were made with an ICP-MS Data Cal ver. 9. (Liu et al. Reference Liu, Hu, Gao, Günther, Xu, Gao and Chen2008), and concordia diagrams were prepared and weighted mean calculations performed using Isoplot3 software (Ludwig, Reference Ludwig2000).
5. Analytical results
5.a. Major and trace elements
The major elements were normalized based on the values in Table 1 to 100% excluding loss on ignition (LOI). Some samples from the Mazongshan complex show high LOI (up to 7.99 wt% for gabbro), indicating different degrees of alteration. Alteration may lead to the migration of some active elements, such as most of the major elements and low-ion lithophile elements (LILEs), but trace elements Zr, Nb, Y and rare earth elements (REEs) Th and Ta are relatively stable. In this study we only used the relatively stable elements mentioned above to determine the lithology, genesis and tectonic settings. On the basis of their geochemical and field characteristics, samples mainly comprised basalts and andesites.
Basalt samples have large variations in contents of SiO2 (51.21–53.80 wt%), MgO (4.02–7.42 wt%), TiO2 (0.87–1.86 wt%), Na2O (2.09–4.87 wt%), Mg no.(42.02–62.92) (where Mg no. is defined as 100 × Mg2+/(Mg2++Fe2++Fe3+)). These samples are high in Al2O3 (16.14–21.42 wt%), FeOT (7.82–10.23 wt%) and CaO (1.08–10.43 wt%), and K2O/Na2O ratios range from 0.01 to 0.42. On a Nb/Y versus (v.) Zr/TiO2 diagram (Fig. 6), they plot in the basalt and andesitic basalt fields. The samples are relatively enriched in light REEs (LREEs) (La/Ybcn = 1.28–6.38, La/Smcn = 0.86–2.6) and differentiated in heavy REEs (HREEs) (Gd/Ybcn = 1.25–1.71). Europium (Eu/Eu* = 0.65–1.04) anomalies are weakly negative to positive.

Fig. 6. Nb/Y v. Zr/Ti diagram of volcanic rocks from the Mazongshan complex (after Winchester & Floyd, Reference Winchester and Floyd1977). Triangles, gabbro; squares, basalt; diamonds, andesite.
All of the seven andesite samples contain SiO2 (54.47–59.75 wt%), Na2O (3.61–5.78 wt%), TiO2 (1.02–3.25 wt%) and FeOT (6.55–12.53 wt%). On chondrite-normalized diagrams (Fig. 7c), they are clearly enriched in LREE (La/Ybcn = 3.81–11.26) and depleted in HREE (Gd/Ybcn = 1.43–1.81). Europium (Eu/Eu* = 0.57–0.87) anomalies are markedly negative.

Fig. 7. (a, b) Chondrite-normalized rare earth element patterns and primitive mantle-normalized spider diagram for basalts, gabbro and serpentinized peridotite from the Mazongshan complex. (c, d) Chondrite-normalized REE patterns and primitive mantle-normalized spider diagram for andesite; standardization values are from Sun & McDonough (Reference Sun, McDonough, Saunders and Norry1989). CAA – continental-arc andesite; OAB – oceanic-arc basalt; MORB – mid-ocean-ridge basalt.
The serpentinized peridotite (P3-100) sample has a relatively high MgO content (23.29 wt%) and low Al2O3 (10.76 wt%) and Na2O (0.87 wt%) contents. The geochemical characteristics of the gabbro sample are similar to those of the basalts in being characterized by 53.61 wt% SiO2, 1.68 wt% K2O, 3.70 wt% Na2O and 4.38 wt% MgO. The gabbro sample displays enriched LREE (La/Ybcn = 3.05) and less differentiated HREE (Gd/Ybcn = 1.18).
5.b. Geochronology
The size of zircon grains in the cumulate gabbro (sample P1-3) ranges from 80 to 200 μm. Most zircon grains have columnar or irregular shapes, and a few have cracks. The CL images (Fig. 8a) display a complex structure. Some grains have clear oscillatory zones of magmatic origin, while others have bright–dark bands or no obvious oscillatory zones. Of the 30 analysed spots, 26 yielded concordant ages; their Th contents ranged from 124.8 to 1378.6 ppm, their U contents from 268.8 to 1148.8 ppm, and their Th/U ratios from 0.4 to 1.2. The Th/U ratio of magmatic zircons is greater than 0.3; the zircons of sample P1-3 are therefore typically magmatic. The ages ranged from 453.8 to 455.6 Ma (Fig. 9a) and yield a weighted mean 206Pb/238U age of 454.6 ± 2.5 Ma (mean square weighted deviation (MSWD) = 0.007), which we interpret to be the time of crystallization of the cumulate gabbro block (Fig. 9b; see Table 2 for more details on data sources).

Fig. 8. Cathodoluminescence images and U–Pb ages of zircons from the Mazongshan complex: (a) cumulate gabbro sample P1-3; (b) gabbro sample P2-6; (c) andesite sample P3-117; (d) tuffaceous slate sample P3-27.

Fig. 9. The Mazongshan complex. (a) U–Pb concordia diagrams for zircons from cumulate gabbro sample P1-3; (b) weighted average 206Pb/238U ages from cumulate gabbro sample P1-3; (c) U–Pb concordia diagrams for zircons from gabbro sample P2-6; and (d) weighted average 206Pb/238U ages from gabbro sample P2-6.
Table 2. U–Pb data for the zircons from samples of the Mazongshan complex

The zircon grains of gabbro sample P2-6 are relatively small, of the order of 50–150 μm. Most grains are euhedral and prismatic and their length/width ratios range from 1.5 to 3. Sample P2-6 contains two zircon types, illustrated by their CL images (Fig. 8b). Most grains have magmatic oscillatory zones of different lengths and widths, but a few have cores with obvious magmatic oscillatory zones. A total of 18 concordant ages were obtained from sample P2-6, but the 206Pb/238U age of point 4 is 652 ± 7 Ma, which is inconsistent with the overall age; this grain was likely inherited from a crustal component of the gabbro source or was captured during magmatic emplacement. The Th contents of the 18 zircons range from 77.9 to 1470.4 ppm, the U contents from 113.5 to 608.6 ppm, and the Th/U ratios from 0.2 to 2.4; these values suggest that the zircons from this gabbro had a magmatic origin. The ages range from 433.5 to 435.3 Ma (Fig. 9c) and yield a weighted mean 206Pb/238U age of 434.1 ± 3.6 Ma (MSWD = 0.0077), which we interpret as the time of crystallization of the gabbro block (Fig. 9d; see Table 2 for more details of data sources).
A total of 12 zircon grains, which were analysed from meta-basaltic andesite sample P3-117, yielded concordant ages. The grains have small and complex morphologies, and their ages range from 445 ± 8 (point 7) to 2800 ± 31 Ma (point 17), which implies that many are detrital zircons. According to their zircon CL images (Fig. 8c), the meta-andesite zircons are divisible into two types. One type is euhedral and prismatic, and characterized by magmatic oscillatory zones of different lengths and widths, and has no inherited zircons. The Th contents of sample P3-117 range from 86.3 to 372.9 ppm, the U contents from 131.6 to 691.5 ppm, and the Th/U ratios from 0.3 to 1.0; these values indicate that the zircons in this basaltic andesite are typically magmatic. The ages have a small range from 445.5 to 459.9 Ma, and 10 concordant ages yield a weighted mean 206Pb/238U age of 451.3 ± 3.5 Ma (MSWD = 0.93) (Fig. 10a). Other zircons are subcircular or round, and their CL images display no rhythmic bands or oscillatory zones (points 4 and 17) (Fig. 8c). The Th/U ratios of points 4 and 17 are 0.5 and 0.6, respectively. These ancient zircons may be inherited from a crustal component of the meta-andesite source. The weighted mean 206Pb/238U age of most magmatic zircons is therefore 451.3 ± 3.5 Ma (MSWD = 0.93), which we interpret as the time of crystallization of the meta-andesite block (Fig. 10b; see Table 2 for more details on data sources).

Fig. 10. The Mazongshan complex. (a) U–Pb concordia diagrams for zircons from andesite sample P3-117; (b) weighted average 206Pb/238U ages from andesite sample P3-117; (c) U–Pb concordia diagrams for zircons from tuffaceous slate sample P3-27; and (d) probability histograms 206Pb/238U ages from tuffaceous slate sample P3-27, Y.A.-the youngest age.
The zircon grains in the tuffaceous slate sample P3-27 range from 60 to 150 μm in size. Most grains have columnar or irregular shapes, and a few have metamorphic edges. The CL images (Fig. 8d) clearly show complex structures. Some grains have obvious oscillating zones of magmatic type, but others have bright–dark bands or no oscillatory zones. A total of 98 spots were analysed, 54 of which yielded concordant ages. The Th contents of these 54 zircons range from 38.0 to 485.6 ppm, the U contents from 111.2 to 576.7 ppm, and the Th/U ratios from 0.3 to 0.8. The Th/U ratio of magmatic zircons is greater than 0.3; the zircons of sample P1-3 are therefore typically magmatic. The ages range from 355.1 to 374.4 Ma (Fig. 10c), and the youngest 206Pb/238U age is 353.6 ± 5.1 Ma. We interpret this youngest age to be the time of deposition of the tuffaceous pelite, which is not older than early Carboniferous (Fig. 10d; see Table 2 for more details of data sources).
6. Discussion
6.a. Age and spatial distribution of the Mazongshan complex
The times of formation of the blocks and matrix of the mélange in the Mazongshan complex in the central Beishan accretionary collage have, so far, remained undetermined, meaning that their evolution is not well understood. Our two gabbros have U–Pb zircon ages of 454.6 and 434.1 Ma, and the basaltic andesite has a U–Pb zircon age of 451.3 Ma, that is, the mélange formed between the Late Ordovician and early Silurian periods.
In turbidites, the youngest detrital zircon U–Pb ages can be used to determine their order depositional age limit (Brown & Gehrels, Reference Brown and Gehrels2007; Dickinson & Gehrels, Reference Dickinson and Gehrels2009). The metasedimentary rocks in this study underwent strong deformation, but the Carboniferous zircons retain clear oscillatory zones and high Th/U ratios (> 0.4); their magmatic ages are therefore considered reliable. The age of the youngest detrital zircons in the tuffaceous slate sample is 353.6 ± 5.1 Ma, which constrains the time of deposition as not older than early Carboniferous. A significant feature of the sample is that it has unimodal detrital zircon style with peak ages of 356 Ma, close to their youngest detrital zircon age; the youngest age suggests that early Palaeozoic oceanic subduction in the Central Beishan accretionary collage was ongoing until early Carboniferous time. These new data are inconsistent with previous theories that the early Palaeozoic Palaeo-Asian Ocean closed during the Devonian Period.
Most accretionary orogenic belts worldwide undergo complex tectonic processes, such as subduction, accretion and collision (Windley, Reference Windley and Condie1992; Isozaki, Reference Isozaki1996; Cawood et al. Reference Cawood, Kröner, Collins, Kusky, Mooney, Windley, Cawood and Kröner2009; Xiao et al. Reference Xiao, Mao, Windley, Han, Qu, Zhang, Ao, Guo, Cleven, Lin, Shan and Li2010 c; Wakabayashi, Reference Wakabayashi, Wakabayashi and Dilek2011; Safonova & Santosh, Reference Safonova and Santosh2014). It is particularly difficult to unravel the differential ages of the tectonic fragments in mélanges, regardless of whether they formed in oceanic-island arcs, continental-margin arcs or fore-arc basins. The Beishan subduction–accretion collage occupies a key position within the CAOB and contains the Mazongshan complex, which contains critical data that can help to constrain the evolution of the central Beishan collage, particularly of the complicated HNMX ophiolitic mélange.
The Mazongshan complex is situated in the southern Gongpoquan arc, and is close to the HNMX ophiolitic mélange. Using new geological maps and geochemical data, Song et al. (Reference Song, Xiao, Han, Li, Qu, Guo, Lin and Wang2013 a, b, Reference Song, Xiao, Han and Tian2014, Reference Song, Xiao, Windley, Han and Tian2015) recently suggested that the Lebaquan arc–fore-arc complex belongs to the Gongpoquan arc rather than the Mazongshan complex settings. Notably, Kazakhstan and North Tien Shan are composed of several arc–basin systems, which show a complicated tectonic massif during early Palaeozoic time (Degtyarev, Reference Degtyarev2011; Safonova et al. Reference Safonova, Perfilova, Obut, Savinsky, Chyornyi, Petrenko, Gurova, Kotler, Khromykh, Krivonogov and Maruyama2019). Some recently acquired data suggest that accretion events did not cease during the Silurian Period (Shu et al. Reference Shu, Wang and Zhu2007). We propose that the accretion events to the north of the HNMX ophiolitic mélange are similar to the eastern segment of Kazakhstan in terms of structure, geochronology and subduction polarity.
6.b. Petrogenesis and tectonic setting of the Mazongshan complex
6.b.1. Gabbro and basalts
The gabbro and basalts from the Mazongshan mélange have similar geochemical compositions (Fig. 7; Table 1) in that they all have slightly enriched REE patterns on chondrite-normalized diagrams. However, on normal- (N-) MORB-normalized diagrams, the gabbro and basalts have different high-field-strength element (HFSE) trace-element patterns (Fig. 7b).
It is well known that the geochemical signatures of island-arc basalts are indicated by high LILE/HFSE and LREE/HREE ratios and low Nb/U and Ce/Pb ratios. In contrast, N-MORB-like geochemical signatures are indicated by low LILE/HFSE and LREE/HREE ratios. As a result of hydrothermal alteration by seawater, igneous oceanic crust acquires variably elevated abundances of LILE and Pb relative to N-MORB, so seafloor sediments show significant enrichments in LILE, Pb, LREE, Nb and Ta compared with MORB, despite the Nb–Ta trough in the diagram (Fig. 7b; Zheng, Reference Zheng2019). The gabbro and basalts exhibit variable contents of Zr (54.91–194.30 ppm), Nb (2.04–6.85 ppm), Ta (0.13–2.03 ppm) and Hf (1.58–4.93 ppm), which are close to those of typical modern oceanic arcs (Sun & McDonough, Reference Sun, McDonough, Saunders and Norry1989; Stolz et al. Reference Stolz, Jochum, Spettel and Hofmann1996; Fliedner & Klemperer, Reference Fliedner and Klemperer2000). The Zr/Nb ratios of the gabbro and basalts range from 9.52 to 64.56, which is close to the range of MORB. On chondrite-normalized diagrams (Fig. 7a), the Mazongshan gabbro and group 1 basalts are similar to those of enriched (E-) MORB. Moreover, on primitive mantle-normalized diagrams (Fig. 7b), the geochemical characteristics of the gabbro and group 1 basalts range from altered oceanic basalts to oceanic-arc basalts (OAB). The difference in HFSEs suggests that the sedimentary input to the subduction zone was highly variable. Continental margins adjacent to oceanic subduction zones generally undergo chemical weathering during subduction, transporting a variety of material into the trench including water-soluble elements and water-insoluble detritus.
On the V–Ti/1000 diagram (Fig. 11c), the gabbro and group 1 basalts have low V contents and moderate Ti/V ratios, mainly located in the MORB field. In the Th–Hf/3–Ta discrimination diagram (Fig. 11a), most of our basalts plot in the field of subduction-related, calc-alkaline basalts. This may suggest derivation in an active continental margin, suggesting that the MORB-like source material had been overprinted by subduction-derived components before the partial melting. This conclusion is further supported by the higher Th contents and Th/Yb ratios than those typical of MORB. In general, the metasomatizing agents in subduction zones are mainly hydrous melts and slab-derived fluids (Brenan et al. Reference Brenan, Shaw and Ryerson1995; Elliott et al. Reference Elliott, Plank, Zindler, White and Bourdon1997; Castillo et al. Reference Castillo, Solidum and Punongbayan2002; Zheng, Reference Zheng2012). Accordingly, these relevant chemical distinctions indicate that the gabbro and group 1 basalts most likely formed as juvenile oceanic rocks in a supra-subduction zone setting (Davies & Stevenson, Reference Davies and Stevenson1992; Stolz et al. Reference Stolz, Jochum, Spettel and Hofmann1996; Davidson & Arculus, Reference Davidson, Arculus, Brown and Rushmer2006; Kröner et al. Reference Kröner, Hegner, Lehmann, Heinhorst, Wingate, Liu and Ermelov2008; Dilek & Furnes, Reference Dilek and Furnes2011, Reference Dilek and Furnes2014).

Fig. 11. Tectonic discrimination diagrams for the gabbro, basalts and andesites of the Mazongshan complex. (a) Th–Hf/3–Ta discrimination diagram (after Wood, Reference Wood1980); A, N-MORB; B, E-MOR.; C, OIB; D, calc-alkaline. (b) Th/Yb v. Ta/Yb diagram (after Pearce, Reference Pearce2008, Reference Pearce2014); (c) V v. Ti/1000 diagram (after Shervais, Reference Shervais1982). Triangles, gabbro; squares, basalt; diamonds, andesite.
6.b.2. Andesites
The andesites are characterized by enrichments of LREE and variable enrichments of HREE (Fig. 7b, d). On Th/Yb v. Ta/Yb diagrams (Fig. 11b), the andesites plot in the active continental-arc field, but the TiO2 content is higher than that of continental arcs. Collectively, the geochemical signatures indicate that the andesites formed in an oceanic-arc setting (Sun & McDonough, Reference Sun, McDonough, Saunders and Norry1989; Stolz et al. Reference Stolz, Jochum, Spettel and Hofmann1996; Fliedner & Klemperer, Reference Fliedner and Klemperer2000).
The blocks in the Mazongshan ophiolitic mélange underwent extensive deformation and metamorphism during accretion and emplacement, resulting in fragmentation, shearing and intrusion of hydrothermal veins. Both basalts and andesites exhibit obvious depletions in Nb–Ta, suggesting that their magmas were generated in modern subduction zone settings and that they were affected by continental contamination (Davidson & Arculus, Reference Davidson, Arculus, Brown and Rushmer2006). However, the plots of MgO v. Zr, Th and Nb/La show no correlations, suggesting that crustal contamination played an insignificant role in their genesis (Fig. 12).

Fig. 12. Plots of Zr v. TiO2, La, Nb and Ta for the gabbro, basalts and andesites of the Mazongshan complex. Triangles, gabbro; squares, basalt; diamonds, andesite.
6.b.3. Tectonic setting
We used initial field characteristics to distinguish between the basalts and andesites. However, they both formed during a similar age range (Fig. 2). Our geochemical study reveals that the basic–intermediate rocks in the mélanges consist of subduction-modified E-MORB-like rocks that likely formed in a back-arc basin and arc-related andesites.
The process of accretion incorporates the concept of ocean–continent transition as follows: oceanic crust grows at a mid-oceanic ridge from a plume generated in the mantle transition zone at a depth of c. 440–660 km. An immature arc grows in an ocean by subduction; considering that such arcs can reach some 50 km in thickness, their formation in a supra-subduction zone setting is not far below that depth. Mature continental crust grows at an active continental margin that starts from partial melting of a shallow-dipping oceanic slab as in the Andes, and progresses via emplacement of the magma through the continental crust. An ocean–continent transition is therefore an important tectonic zone within the plate tectonic paradigm, because it records the change from a deep mantle oceanic source to a shallower continental or shallow mantle condition. The co-existence of MORB and IAT rocks has rarely been documented in the Mazongshan complex, where an immature arc crust has grown into a mature arc-continental crust, as in the Coast Range complex of California (e.g. Shervais, Reference Shervais2001; Reagan et al. Reference Reagan, Ishizuka, Stern, Kelley, Ohara, Blichert-Toft, Bloomer, Cash, Fryer, Hanan, Hickey-Vargas, Ishii, Kimura, Peate, Rowe and Woods2010; Xiao et al. Reference Xiao, Li, Pan, Lu, Ding, Deng, Feng, Liu, Kou and Yang2016). Considering the lithological, structural, geochronological and geochemical characteristics of the Mazongshan complex, we propose that it formed in a single back-arc basin that formed above a NW-dipping intra-oceanic subduction zone during Late Ordovician to earliest middle Silurian time (Fig. 13b). As subduction continued, these rocks evolved into the HNMX ophiolitic mélange until the Carboniferous Period.

Fig. 13. Tectonic evolution of the central Beishan accretionary collage, emphasizing the sequential spatial relationships of the Mazongshan complex (modified by Ao et al. Reference Ao, Xiao, Han, Li, Qu, Zhang, Guo and Tian2012; Wang et al. Reference Wang, Zhang, Song, Li, Li and Zhou2017). (a) Cambrian – Early Ordovician: the Niujuanzi Ocean was expanding, as indicated by Sinian moraine gravels situated in the two flanks of the HNMX ophiolitic mélange; age of ophiolites by Hou et al. (Reference Hou, Wang, Liu, Wang and Li2012), Tian et al. (Reference Tian, Xiao, Windley, Lin, Han, Zhang, Wan, Ao, Song and Feng2014) and Shi et al. (Reference Shi, Zhang, Kröner, Li and Jian2018). (b) Middle–Late Ordovician: Niujuanzi oceanic crust subducted N-wards beneath the Mingshui–Hanshan massif at least since Middle Ordovician time, forming the Mazongshan intra-arc-basin system during Late Ordovician time (after Ao et al. Reference Ao, Xiao, Han, Li, Qu, Zhang, Guo and Tian2012; Song et al. Reference Song, Xiao, Windley, Han and Tian2015). (c) Late Ordovician – late Silurian: magmatic activity peaked on both sides of the HNMX, which suggests bidirectional subduction and shrinkage of the Niujuanzi Ocean (Mao et al. Reference Mao, Xiao, Fang, Wang, Han, Sun and Yuan2012; Zheng et al. Reference Zheng, Xiao, Li, Wu and Zhang2018, Reference Zheng2019). (d) Early Devonian – early Carboniferous: the youngest age of the turbidites indicates that accretionary growth continued to the north until early Carboniferous time (Wang et al. Reference Wang, Zhang, Song, Li, Li, Yu and Bu2018 a).
6.c. Implications and tectonic evolution
The evolution of the Mazongshan subduction–accretion complex took place over the following four stages (Fig. 13).
-
(1) An ocean opened along an accreting tectonic boundary of the HNMX ophiolitic mélange during early Cambrian time, as revealed by high-resolution geochronology and field characteristics (Gangu Survey Institute, 2001; Chen et al. Reference Chen, Xiu, Pan, Zhang, Zhang, Li and Zhuan2017; Shi et al. Reference Shi, Zhang, Kröner, Li and Jian2018).
-
(2) During a second stage, the passive continental margin was transformed into an active continental margin. The EW-trending Gongpoquan arc developed on the southern margin of the Mingshui–Hanshan Massif, and the HNMX ophiolitic mélange, which is made of MORB-type basalts and gabbros, formed during N-wards oceanic subduction beneath the Mingshui–Hanshan Massif (Ao et al. Reference Ao, Xiao, Han, Li, Qu, Zhang, Guo and Tian2012; Song et al. Reference Song, Xiao, Windley, Han and Tian2015; Wang et al. Reference Wang, Zhang, Song, Li, Li and Zhou2017). The emplacement of the northern Lebaquan complex, the product of N-dipping subduction and other events within the Mazongshan complex during Ordovician – late Silurian time (Song et al. Reference Song, Xiao, Windley, Han and Tian2015), suggests that intra-ocean subduction continued until at least Middle Ordovician time (Ao et al. Reference Ao, Xiao, Han, Li, Qu, Zhang, Guo and Tian2012).
-
(3) Magmatic activity peaked during Late Ordovician – late Silurian time (Zuo et al. Reference Zuo, Jin and Feng1994; Xiao et al. Reference Xiao, Mao, Windley, Han, Qu, Zhang, Ao, Guo, Cleven, Lin, Shan and Li2010 c; Mao et al. Reference Mao, Xiao, Fang, Wang, Han, Sun and Yuan2012; Song et al. Reference Song, Xiao, Han and Tian2014; Ding et al. Reference Ding, Han, Xiao, Wang and Yang2015; Wang et al. Reference Wang, Yuan, Zhang, Long, Sun, Wang, Soldner and Lin2018 b; Zheng et al. Reference Zheng2019). With continuous N-wards subduction of the Palaeo-Asian Ocean, andesites and basalt–andesite–rhyolite–quartz–trachyte–trachy basalts were extruded in the Gongpoquan subduction-generated arc (Wang et al. Reference Wang, Li, Xu, Yu, Guo, Yan, Ji and Wu2016, Reference Wang, Yuan, Zhang, Long, Sun, Wang, Soldner and Lin2018 b; Zheng et al. Reference Zheng2019). Our chemical and age data suggest that the Mazongshan complex formed in a back-arc basin that began to shrink as a result of the N-wards subduction of the Niujuanzi Ocean or Palaeo-Asian Ocean during Late Ordovician – early Carboniferous time. By considering the timing (Xiao et al. Reference Xiao, Mao, Windley, Han, Qu, Zhang, Ao, Guo, Cleven, Lin, Shan and Li2010 c; Mao et al. Reference Mao, Xiao, Fang, Wang, Han, Sun and Yuan2012; Ding et al. Reference Ding, Han, Xiao, Wang and Yang2015), we can conclude that the southern magmatic arc in the central Beishan accretionary collage resulted from two-way subduction of the Niujuanzi Ocean after Late Ordovician Epoch.
-
(4) The final stage of evolution was the migration of the Mazongshan subduction–accretion complex towards the ocean during late Silurian – Carboniferous time. During early Silurian – Early Devonian time, the Niujuanzi Ocean may have been subducting beneath the accretionary wedge. During this process, extensive Silurian–Devonian magmatism resulted from the subduction of a spreading ridge and the opening of a slab window (Zheng et al. Reference Zheng, Xiao, Li, Wu and Zhang2018, Reference Zheng2019). The central Beishan accretionary collage is a complicated region, in which subduction apparently began from the Late Ordovician Epoch (454.6 Ma). Integration with other published data suggests that the younging direction of accretion was from north to south, and that accretion continued until early Carboniferous time (353.6 Ma).
7. Conclusions
-
(1) The Mazongshan subduction–accretion mélange is characterized by a ‘block-in-matrix’ structure, the ‘blocks’ mainly consisting of serpentinized peridotite, basalt, gabbro, andesite, chert and seamount sediments in a matrix of turbidite and shale.
-
(2) The Mazongshan complex evolved from a variety of sources and tectonic environments. The ophiolitic rocks that have an E-MORB geochemical signature formed with tholeiitic and alkaline basalts and diorites during initial intra-ocean subduction. The lithologies and geochemical characteristics of continental-arc lavas indicate formation in a relatively mature arc during the change from an initial immature oceanic arc to a mature continental arc.
-
(3) The Mazongshan mélange contains two gabbro blocks that have U–Pb zircon ages of 454.6 ± 2.5 and 434.1 ± 3.6 Ma, and an andesite block with an age of 451.3 ± 3.5 Ma; these isotopic ages indicate that the Mazongshan complex formed during Late Ordovician – early Silurian time. The age of the oldest rocks (454.6 ± 2.5 Ma) provides the time of initial accretion and tectonic emplacement after the Late Ordovician Epoch.
-
(4) The age of the tuffaceous slate (353.6 ± 5.1 Ma) and of the youngest rocks (353.6 ± 5.1 Ma) indicates that the accretion continued until early Carboniferous time. The Mazongshan back-arc basin began to shrink as a consequence of N-wards subduction of the Palaeo-Asian Ocean during Late Ordovician – early Carboniferous time. Subsequently, it was accreted as a relict ophiolite into the accretionary orogen that we see today.
Acknowledgements
This study was funded by the National Natural Science Foundation of China (grant no. 41772107), the Geological Survey of China (grant no. DD20190370), the National Key Research and Development Program of China (grant no. 2016YFC0601005) and the National Nonprofit Institute Research Grant of IGGE (grant no. AS2019Y01).