Introduction
During the decade of 2002–2011, the global mean surface temperature was 0.77 to 0.80°C warmer than the pre-industrial average; in Europe, the increase reached 1.3°C in terrestrial areas (EEA, 2012). Climatic models predict that global warming will continue in the coming decades. The mean global temperature is projected to increase by 1.1–6.4°C by 2100, while mean temperatures in Europe are expected to rise by 2.5–4.0°C for the same period (EEA, 2012). Models also predict increased weather variability and increased severity and frequency of extreme weather events such as heat waves, droughts and extreme precipitation (EEA, 2012; IPCC, Reference Pachauri and Meyer2014). These climatic events can have major effects on many organisms. In particular, increasing temperatures are likely to affect the behaviour, development, reproduction, survival and geographical distribution of ectothermic organisms, as their physiological processes are highly dependent on ambient temperatures (Bale et al., Reference Bale, Masters, Hodkinson, Awmack, Bezemer, Brown, Butterfield, Buse, Coulson, Farrar, Good, Harrington, Hartley, Jones, Lindroth, Press, Symrnioudis, Watt and Whittaker2002; Menéndez, Reference Menéndez2007). While an increase in temperature within a favourable range will tend to speed up their metabolism and development, an increase above the optimal threshold will reduce survival or fitness, due to the disruption of metabolic functions (Bale et al., Reference Bale, Masters, Hodkinson, Awmack, Bezemer, Brown, Butterfield, Buse, Coulson, Farrar, Good, Harrington, Hartley, Jones, Lindroth, Press, Symrnioudis, Watt and Whittaker2002; Chiu et al., Reference Chiu, Kuo and Kuo2015). Further, the response of insects to climate depends on the developmental stage and phenology (i.e. the timing of life cycle events) (Bale et al., Reference Bale, Masters, Hodkinson, Awmack, Bezemer, Brown, Butterfield, Buse, Coulson, Farrar, Good, Harrington, Hartley, Jones, Lindroth, Press, Symrnioudis, Watt and Whittaker2002; Walther et al., Reference Walther, Post, Convey, Menzel, Parmesan, Beebee, Fromentin, Hoegh-Guldberg and Bairlein2002; Deutsch et al., Reference Deutsch, Tewksbury, Huey, Sheldon, Ghalambor, Haak and Martin2008). For example, the larval growth can be accelerated by higher temperature whereas the duration of diapause may be extended (Battisti & Jactel, Reference Battisti and Jactel2010).
Most studies on the effects of climate change on species and populations, e.g. species distribution modelling (SDM) approaches, usually consider mean monthly, seasonal or annual conditions. Similarly, most experimental studies test the consequences of constant temperatures (CT) under controlled laboratory conditions. However, organisms do not simply experience mean conditions but are exposed to daily fluctuations in temperatures, and a few hours above a lethal maximum can impede species survival or expansion. These daily dynamics have been generally ignored in the climate change literature and could have important effects on the fitness of organisms (Easterling et al., Reference Easterling, Meehl, Parmesan, Changnon, Karl and Mearns2000; Walther et al., Reference Walther, Post, Convey, Menzel, Parmesan, Beebee, Fromentin, Hoegh-Guldberg and Bairlein2002; Paaijmans et al., Reference Paaijmans, Heinig, Seliga, Blanford, Blanford, Murdock and Thomas2013).
A heat wave is defined by the World Meteorological Organization and according to the fourth and fifth IPCC assessment reports as a temperature regime in which the daily highest temperatures are 5°C above the mean maximum temperature for a few consecutive days. Such short-term temperature dynamics can affect life-history traits and fitness beyond the effects of mean temperatures alone (Martin & Huey, Reference Martin and Huey2008; Bozinovic et al., Reference Bozinovic, Bastias, Boher, Clavijo-Baquet, Estay and Angilletta2011; Clusella-Trullas et al., Reference Clusella-Trullas, Blackburn and Chown2011; Folguera et al., Reference Folguera, Bastias, Caers, Rojas, Piulachs, Belles and Bozinovic2011). The effects of heat waves are particularly severe once they exceed the thermal optima for development, fecundity and/or fitness (Chiu et al., Reference Chiu, Kuo and Kuo2015). The negative effects depend on the accumulated damage resulting from exposure to a given temperature. If temperatures are high relative to optimal conditions, even a short exposure can result in substantial mortality. Likewise, longer exposure to high, but not lethal, temperatures may not result in high mortality if individuals take advantage of the lower night temperatures to recover (Mironidis & Savopoulou-Soultani, Reference Mironidis and Savopoulou-Soultani2010).
The pine processionary moth Thaumetopoea pityocampa (Denis & Schiffermüller) (Lepidoptera: Notodontidae) is the most damaging and widespread defoliator of conifers in the Mediterranean region. Adult emergence, immediately followed by mating and egg laying, occurs in the summer. Eggs take about one month to hatch. The larvae feed on pine needles throughout the fall and winter. In spring, they burrow in the soil to pupate and undergo pupal diapause until the following summer (Démolin, Reference Démolin1969). This phenology, characterized by the development of larvae in winter, is observed in most T. pityocampa populations, hereafter called winter populations (WPs). An anomalous population displaying a shifted life cycle was discovered in Leiria (Portugal) about 20 years ago. In this spatially localized population, hereafter called SP (summer population), the larvae develop in the summer, pupate in September and the adults reproduce April–May. SP is genetically differentiated from the local sympatric WP from which it is hypothesized to originate (Santos et al., Reference Santos, Rousselet, Magnoux, Paiva, Branco and Kerdelhué2007, Reference Santos, Burban, Rousselet, Rossi, Branco and Kerdelhué2011a ; Burban et al., Reference Burban, Gautier, Leblois, Landes, Santos, Paiva, Branco and Kerdelhué2016). Experimental studies showed that this phenology is heritable and possibly originated from a mutation in one or more genes (Branco et al., Reference Branco, Paiva, Santos, Burban and Kerdelhué2017). As a consequence of this shift in phenology, the different stages of the SP are facing different climatic environments as compared with the WP, with possible implications on climate adaptations as observed on the larval stage (Santos et al., Reference Santos, Paiva, Tavares, Kerdelhué and Branco2011b ). We hypothesized that differences in temperature tolerance may also occur in the egg stage.
The relationship between T. pityocampa and climate is important as warmer temperatures have led to recent range expansions towards higher latitudes and altitudes in some regions (Hódar & Zamora, Reference Hódar and Zamora2004; Battisti et al., Reference Battisti, Stastny, Netherer, Robinet, Schopf, Roques and Larsson2005). This expansion is clearly associated with warming that may improve larval performance and winter survival at the leading, cold edge of the distribution (Hódar et al., Reference Hódar, Castro and Zamora2003; Battisti et al., Reference Battisti, Stastny, Netherer, Robinet, Schopf, Roques and Larsson2005; Robinet et al., Reference Robinet, Baier, Pennerstorfer, Schopf and Roques2007; Hoch et al., Reference Hoch, Petrucco Toffolo, Netherer, Battisti and Schopf2009). However, increased temperatures can also produce negative feedbacks, as the heat waves that are predicted to become more frequent may affect eggs and early larval stages during summer and autumn (Robinet et al., Reference Robinet, Baier, Pennerstorfer, Schopf and Roques2007). This is particularly relevant in the southern parts of the distribution range, where temperatures are high and might possibly reach lethal limits. T. pityocampa eggs and young larvae are sensitive to high temperatures and an excess of heat or exposure to intense solar radiation may induce high mortality (Démolin, Reference Démolin1969). Indeed, a threshold of 32°C was proposed as a lethal temperature limit for eggs and young larvae by Huchon & Démolin (Reference Huchon and Démolin1970). Consistent with this, the exceptional heat that occurred in Europe during the summer of 2003, with maximum temperatures exceeding 40°C, caused a huge decrease in T. pityocampa populations in northern France (Robinet et al., Reference Robinet, Rousselet, Pineau, Miard and Roques2013).
Regarding the tolerance of insects to extreme temperatures, few studies investigate the egg stage; most of them focus on the larval stage. Still, while larvae are able to thermoregulate in nature (Kührt et al., Reference Kührt, Samietz, Höhn and Dorn2006) or seek for refugia such as suitable micro-habitats, eggs cannot move or escape, and thus are a highly sensitive stage (Potter et al., Reference Potter, Davidowitz and Woods2011). Therefore, gaining knowledge about thermal tolerance of eggs is most important to understand responses to the ongoing climate warming. Robinet et al. (Reference Robinet, Rousselet, Pineau, Miard and Roques2013) simulated the potential effects of the heat wave of 2003 on T. pityocampa egg masses and concluded that a maximum temperature of 40°C for 5 h during 1, 3, 5 and 12 consecutive days had no effects on egg survival. The authors assumed that the possible effect of the 2003 heat wave on T. pityocampa populations was due to higher mortality in the young larval stages, which are sensitive to temperatures above 36°C as demonstrated by Santos et al. (Reference Santos, Paiva, Tavares, Kerdelhué and Branco2011b ). However, no studies to date have analysed the effects of temperatures above 40°C on the T. pityocampa embryo stage, so the upper temperature threshold for egg survival is still unknown in this species.
Our main goal was here to evaluate the effects of heat wave episodes on egg survival in T. pityocampa by testing a large range of maximum temperatures from 36 to 48°C; thus, encompassing the potential range of extreme temperatures that egg masses may experience under current and future climate warming scenarios. We also aimed to test for differences in tolerance of eggs to high temperatures between the unique SP and two WP populations from Portugal and Tunisia. The Tunisian population was sampled from a region where extreme summer maximum temperatures frequently exceed 40°C, while the eggs are developing; we therefore expected that the corresponding embryos would be more tolerant to high temperatures. Additionally, considering that variation in temperature can influence insect survival (Paaijmans et al., Reference Paaijmans, Heinig, Seliga, Blanford, Blanford, Murdock and Thomas2013), we tested whether egg survival differed between CT regimes and daily temperature regimes (DT) with equivalent mean temperature. Finally, we wanted to analyse the effect of a pulse of high temperatures on the embryo developmental time.
Material and methods
Sampling of egg masses
In the field, egg laying occurs from the end of April to mid-June for SP, from the beginning of August to September for the Portuguese WP (hereafter PWP) and from mid-September to October for the Tunisian population (hereafter TWP). SP egg masses were thus collected in Nazaré, Leiria (N39°36′50.70″; W9°04′25.80″) between the end of May and middle of June; for PWP, egg masses were collected in Pinhal Freiras, Setúbal (N38°34′42″; W9°07′35″) in the beginning of September; Tunisian egg masses were collected in mid-September in Djebel Mansour (N36°16′0.0″, W9°42′0.0″). Portuguese populations were collected in maritime pine, Pinus pinaster Aiton stands whereas Tunisian egg masses were collected in Aleppo pine, Pinus halepensis Miller and subsequently transported by plane to Portugal. Egg masses were individually placed in glass test tubes and kept at room temperature (22–28°C) until trials. Experiments were conducted from 2009 to 2014 for the Portuguese populations and in 2012 and 2013 for the Tunisian population.
The average maximum temperatures for the three sites and periods indicated above are respectively 21.6°C for Leiria (period May–June), 27.9°C for Setúbal (period August–September) and 28.5°C for Djebel Mansour (period September–October), as estimated from weather stations data from the period 1982–2012 (WorldClim by Hijmans et al., Reference Hijmans, Cameron, Parra, Jones and Jarvis2005).
Heat treatments
The tolerance of embryos to high temperatures was tested by exposing egg masses to 3-days of hot temperatures simulated in laboratory-controlled conditions, mimicking heat waves. According to the definition of the IPCC (Reference Pachauri and Meyer2014), a heat wave occurs when temperatures exceed the mean maximum temperature by 5°C during a few consecutive days. A 3-days period was chosen for comparison with previous results on the larval stage for the same species (Santos et al., Reference Santos, Paiva, Tavares, Kerdelhué and Branco2011b ). Two temperature regimes were developed, namely (i) DT cycles and (ii) constant heat treatments (CT), using a range of daily average temperatures from 30 to 42°C in both cases. Both CT and DT regimes were implemented in climate chambers Fitoclima S600PL (ARALAB, Portugal), with relative humidity fixed at 60% and photoperiod of 14:10 h (L:D). Data loggers OPUS10 were placed inside the chambers to confirm temperature and relative humidity values.
For DT regime, night temperature was fixed 10°C below the maximum temperature (T max) reached during the day. In the morning, temperature gradually increased from the night temperature to T max in 6 h, and was then kept constant for the following 4 h, corresponding to the expected length of the warmest daily period in the field. Finally, it progressively decreased during the remaining 4 h until reaching the night temperature (T max −10°C) (fig. 1). For clarity, we will hereafter refer to each DT treatment by its daily average temperature, using the codes DT30 (T max = 36°C), DT32 (T max = 38°C), DT34 (T max = 40°C), DT36 (T max = 42°C), DT38 (T max = 44°C), DT40 (T max = 46°C) and DT42 (T max = 48°C). Due to limited sampling sizes, Tunisian populations were tested only for DT32, DT34, DT36 and DT38.

Fig. 1. Temperature daily cycle regimes (DT) simulating heat waves. Each line represents one treatment of fluctuating temperatures with a daily heat peak at maximum temperature T max.. Each cycle was repeated for three consecutive days.
Egg masses from SP and PWP were also exposed to CT regimes, which simply consisted of three consecutive days at a given temperature, using the same photoperiod and relative humidity as above. The temperatures selected corresponded to the average daily temperatures used in DT experiments, and were noted CT30, CT32, CT34, CT36, CT38, CT40 and CT42. Number of replicates for each regime and condition are shown in table 1. We maximized replicates for controls and for the conditions that proved to be close to the mortality thresholds (see ‘Results’ section).
Table 1. Number of egg masses and average number of eggs per egg mass ± SE used in the temperature experiments for each tested population: Portuguese SP, Portuguese PWP and Tunisian TWP.

The experiments were all conducted in Portugal, at the University of Lisbon. They took place in spring for SP, in late summer for PWP and in fall for TWP egg masses, i.e. following their respective sampling periods. For both CT and DT regimes, control groups were held at room temperature (25 ± 2°C) rather than outdoor conditions to ensure that the control conditions were similar between populations in spite of differences in sampling seasons. Note that 25°C falls within the range of optimal temperature for egg development (Démolin, Reference Démolin1969). In each experimental temperature regime and condition, from 5 to 35 egg masses were tested depending on the number of available egg masses; we used a higher number of replicates for the control (25°C) because we had to perform several repetitions over several years (table 1). To allow adaptation from room to higher temperatures, the DT cycle regimes always started from the lower level (night temperature), whereas for the constant regimes CT temperatures increased gradually during 1 h until reaching the target temperature. In all cases, after the 3-days treatment, egg masses were placed back at room temperature (~25°C) and 60% relative humidity until hatching. Hatch date was recorded. Newly hatched larvae were counted, carefully removed, and kept alive in Petri dishes with fresh pine needles for 2–3 days to test their ability to survive after heat treatment. At the end of the experiments, and after a period of at least 50 days in case no eggs had hatched, egg masses were inspected under a binocular microscope (Olympus, SZX12). The number of hatched/unhatched eggs and of parasitized eggs (eggs with a parasitoid exit hole or still containing a parasitoid) were counted. Parasitized eggs were then discounted from the total number of eggs used in any given experiment.
Effect of temperature on the embryo development time
We used egg masses obtained in laboratory conditions, for which we had the exact date of egg laying, to test the effect of different temperature treatments on duration of embryonic development. These eggs were obtained from females resulting from PWP pupae collected in the field that emerged and mated in the laboratory (rearing protocols as described in Branco et al., Reference Branco, Paiva, Santos, Burban and Kerdelhué2017). Egg masses are difficult to obtain in laboratory conditions, because of a set of constraints such as an obligate univoltine life cycle, a high natural mortality during the larval and pupal stages, the lack of artificial diet, the highly urticating hairs of the larvae and the short lifespan of the adults and the consequent difficulty of synchronizing emergence to obtain successful mating. Therefore, the numbers of replicates for this experiment were limited. The following treatments were tested: CT36, CT38, CT40 as well DT30, DT32, DT34 and DT36 (N = 5 or 4). Control groups were placed at 25°C (N = 9 and 13, respectively). Age of egg masses varied between 3 and 12 days for this experiment, i.e. they were at 10–40% of their embryonic development when tested.
Data analysis
To compare egg survival among the three populations (SP, PWP and TWP) and among temperatures for the DT30, DT32, DT34, DT36 treatments, the total numbers of non-parasitized eggs exposed to heat treatment was analysed using a binomial distribution with a logit link function through Generalized Linear Models (GLM). Pairwise comparisons of estimated marginal means based on the events/trials proportion were obtained using least significant differences (α = 0.05).
The same procedure was used to compare egg survival between the DT and CT regimes for each population and each tested daily average temperature. First we considered a model with two factors, temperature and regime, but since interaction term was significant, we then used separate models for each temperature. Development time was tested by one way ANOVA after the verification of Levene's test for homogeneity of variances considering the factor temperature. Pairwise comparisons were obtained using least significant differences. All data were analysed with the software program SPSS 22 (SPSS Inc) and using overlap of 95% confidence intervals.
Results
Since egg masses were collected in the field, parasitoids were also recovered. PWP egg masses were the most parasitized, mainly by Baryscapus sp., with 21 ± 2% of parasitism, followed by TWP with 8 ± 1.1% of parasitized eggs (mean ± S.E.). SP egg masses were practically free of parasitoids (1.8 ± 0.4%) as observed in previous studies (Santos et al., Reference Santos, Paiva, Rocha, Kerdelhué and Branco2013).
Differences between T. pityocampa populations in DT
The three populations overall differed in their fecundity. Mean number (±SE) of eggs per egg mass was higher for PWP and TWP (147 ± 14.6 and 163 ± 9.2, respectively) than for SP (120 ± 9.6) (table 1).
There were overall significant differences in egg survival between populations (W = 286.9, df = 2, P < 0.001) and among temperatures (W = 1165.3, df = 4, P < 0.001). Moreover, the interaction term was also significant (W = 16.3, df = 7, P = 0.022). When comparing populations for each tested temperature, TWP had lower survival than both PWP and SP for all temperatures including control (table 2).
Table 2. Survival (average ± SE) of egg masses of Portuguese SP, Portuguese PWP and Tunisian TWP populations exposed to the daily cycle temperature (DT) regimes with maximum temperature (T max) of 36, 38, 40, 42 and 44°C and the control at 25°C constant temperature.

Values followed by the same lowercase letter within the same row and by the same capital case letter within the same column are not statistically different as determined by least significant differences (α = 0.05).
For each population there was a significant effect of temperature (W = 375.4, 389.4 and 431.2 for SP, PWP and TWP, respectively, P < 0.001 in all cases). Still, in all populations, egg survival did not differ significantly from the control (25°C) for all temperatures up to DT34 (table 2). A negative effect of temperature was observed at DT36 for all populations, with a higher decrease in survival when compared with the control for TWP (about 21%) than for the two Portuguese populations (13 and 9% for PWP and SP, respectively). At DT38, survival was 0% for all populations.
For all treatments and populations, the survival of the neonate larvae was 100% during the first 2–3 days, when larvae were fed and observed.
Cycle vs. CT effect
Differences between CT and DT conditions corresponding to the same daily average temperatures were tested in the two Portuguese populations. Up to an average temperature of 34°C (CT34 and DT34), survival was very high (>90%) and was not significantly different between the two regimes (fig. 2, table 3), nor different from the control. A negative effect of heat treatment started in the pair CT36/DT36 with a similar decrease in survival for both DT and CT regimes for each population (fig. 2). Although egg survival decreased for both populations in these trials, the mortality was higher for PWP (fig. 2). Still, for the pairs CT38/DT38 and CT40/DT40 significant differences were found between the two regimes (table 3). For both DT38 and DT40 survival was 0% for both populations, while for the equivalent CT38 and CT40 egg survival was observed to be 70 ± 0.9 and 38 ± 1.0% in the case of PWP, and 70 ± 0.7 and 1 ± 0.1% in the case of SP, respectively (fig. 2). For DT42 and CT42, mortality was 100% for both populations.
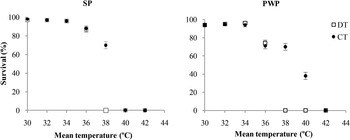
Fig. 2. Egg survival (average ± 2SE) in two experimental regimes: daily cycles (DT) vs. constant temperatures (CT) for two Portuguese populations, SP and PWP. Horizontal axis, mean temperature corresponding to maximum temperatures (T max) for daily cycles as follow: DT30 (T max = 36), DT32 (T max = 38), DT34 (T max = 40), DT36 (T max = 42), DT38 (T max = 44), DT40 (T max = 46), DT42 (T max = 48).
Table 3. Comparison of embryo survival of egg masses exposed to constant temperature (CT) and daily cycles (DT) regimes for Portuguese SP and Portuguese PWP populations.
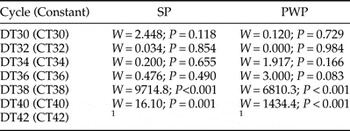
1 No embryo survived at this temperature regime.
While living adult parasitoids were found in all treatments, the egg masses subjected to the highest temperature also hosted dead adult parasitoids. The maximum temperature treatments for which parasitoids emerged were CT38 (nine Ooencyrtus sp. and 26 Baryscapus sp.) and DT36 (one Ooencyrtus sp. and 14 Baryscapus sp.). Since we could not control the number of parasitoids in each regime, it was not possible to statistically test the effects of temperature on the survival of the egg parasitoids.
Effect of temperature on the embryo development time
In the constant regime, the CT36, CT38 and CT40 conditions all had a significant effect on the egg developmental time causing an increased delay in hatching with increased heat treatment in comparison with the control (table 4). A similar increase in development time was observed when eggs were submitted to DT32, DT34 and DT36 (table 4). The longest embryo development time was observed for DT36 with a mean of 35 days (table 4), i.e. 5 days longer than the mean development time at 25°C.
Table 4. Development time (average ± SE) (days) of Portuguese PWP egg masses exposed to constant temperature (CT) and daily cycle temperature (DT) regimes, at different maximum temperatures (T max).
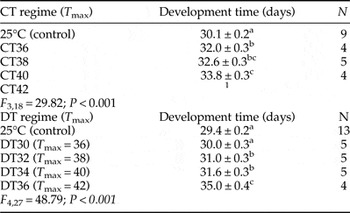
1No embryo survived in this temperature regime.
Values followed by the same lowercase letter within the same column are not statistically different as determined by least significant differences pairwise comparison (α = 0.05).
Discussion
Identification of upper lethal temperatures for T. pityocampa embryos
Many studies have demonstrated the detrimental effects of high temperatures on insect survival. Yet, the threshold limits are species and stage specific (Chiu et al., Reference Chiu, Kuo and Kuo2015), and can only be identified through stage-specific experimental studies for each species. Most of the studies conducted thus far concern the larval, nymphal or adult stages and only a few of them have explored the egg stage. These studies demonstrate large differences among species in their sensitivity to temperature. For example, in a study of the effect of high temperatures on mortality of different life stages of the almond moth Ephestia cautella (Lepidoptera), Darwish et al. (Reference Darwish, Ali, Mohamed and Khalil2015) showed that the egg stage was the most sensitive to high temperatures and that an exposure to 45°C for 59 min or more caused 95% egg mortality. In a study conducted with Parahypopta caestrum (Lepidoptera), Salpiggidis et al. (Reference Salpiggidis, Navrozidis and Copland2004) found that eggs were already negatively affected at 35°C.
For T. pityocampa, we observed a severe decline of embryo survival when T max reached 42°C, and 100% mortality above this threshold. On the other hand, up to T max = 40°C, the survival of the heat-treated groups did not differ from the controls kept at 25°C. In the study conducted by Robinet et al. (Reference Robinet, Rousselet, Pineau, Miard and Roques2013), the authors concluded that a heat wave corresponding to a maximum of 40°C (T max) for 5 h a day over 12 days had no effect on T. pityocampa egg survival, which is consistent with our findings. Using higher temperatures, we could identify the upper lethal temperature for T. pityocampa egg stage. This limit is situated near 42°C, and affects egg survival after only 3 days heat wave. Interestingly, this temperature is well above of the 32°C that Huchon & Démolin (Reference Huchon and Démolin1970) reported as the lethal temperature of eggs and young larvae. Differences between this past study, in which no clear protocol was described, and recent ones could be attributed to different methodologies, to differences among populations or possibly to adaptive evolution that could have occurred in T. pityocampa populations during the past 40 years as a consequence of climate warming, as already suggested in Santos et al. (Reference Santos, Paiva, Tavares, Kerdelhué and Branco2011b ).
We showed that the lethal temperature threshold for T. pityocampa embryos is relatively high, which is consistent with the Mediterranean origin of the species (Simonato et al., Reference Simonato, Battisti, Kerdelhué, Burban, Lopez-Vaamonde, Pivotto, Salvato and Negrisolo2013). Temperatures above 42°C are not frequent but can be sometimes observed in the studied area in very hot summer years (Santos et al., Reference Santos, Paiva, Tavares, Kerdelhué and Branco2011b ). Further, T. pityocampa females tend to lay their eggs exposed to the sun, and since temperature below the scales of the egg masses may be higher than the air temperature (Robinet et al., Reference Robinet, Rousselet, Pineau, Miard and Roques2013), an exposure to temperatures above 42°C can occur even when air temperatures under shelter remain below that threshold. Lastly, as we could not precisely control the age of the egg masses sampled in the field, we cannot rule out the hypothesis that embryos at the very beginning or end of development would be sensitive to lower temperatures than the threshold obtained from our results, and that we tend to underestimate the negative effect of heat waves. Thus, egg mortality due to heat waves may occur in the studied region and become even more frequent in the future according to climate change scenarios (EEA, 2012).
Daily cycling vs. CT regimes
Most studies on the effects of climate change consider mean temperatures or mean maximum values, and do not consider that a short exposure (few days or few hours) above a certain temperature can cause high mortality. Experimental protocols using CTs are often chosen because they are easy to handle in the laboratory. Yet, they are likely to result in a number of biases (Robinet & Roques, Reference Robinet and Roques2010). Moreover, changes in mean temperatures do not reflect those occurring in maximum and minimum temperatures, which can affect survival and development and could hinder any advantages offered by a warmer mean temperature in temperate environments (Vasseur et al., Reference Vasseur, DeLong, Gilbert, Greig, Harley, McCann, Savage, Tunney and O'Connor2014). Our study showed that both CT and DT regimes induce low mortality until a certain threshold is reached. On the other hand, the two regimes differ near the upper limit. For example, for a similar daily mean temperature of 38°C, no embryo survived at DT38 for both populations while survival was still high (70%) for both populations in the constant CT38 experimental condition. Thus extreme values of both mean and maximum DTs and their probability of occurrence in a given environment should be taken into account in probabilistic models of species distribution, as both can affect the probability of local extinction.
Temperature thresholds differ among populations
Three populations differed in egg survival. For all temperatures tested, including the control, embryo survival was highest in SP and lowest in TWP, while differences between SP and PWP were minor. Since the differences among populations were also significant for the control (25°C), they are possibly explained by factors besides the experimental treatment. The larger egg size of SP, and thus the greater amount of available resources (Santos et al., Reference Santos, Paiva, Rocha, Kerdelhué and Branco2013), could explain why embryo survival was highest for this population. In the case of the Tunisian population we assume that their transport conditions, including airplane travel, might have partially affected egg viability. Embryo tolerance to temperature could also be affected by egg age, which might have influenced the results but cannot explain differences observed for the control group. On the other hand, host trees differed between Tunisia and Portugal; we may assume that host food quality of the mother female affect embryo survival. Studies using similar host tree species for all populations would be needed to test this hypothesis. However, other factors than pine species could play a role to possibly change egg mass characteristics in the two populations.
In the CT experiments, we found that PWP embryos were more tolerant to high temperatures than those of SP, as 38% of PWP eggs could survive to a constant 3-day temperature of 40°C whereas all SP embryos died under these same conditions (fig. 2). This may be explained by the fact that PWP eggs are laid during summer and exposed to daily maximum temperatures that may exceed 40°C. On the contrary, SP eggs are laid during the spring and are typically not exposed to such high temperatures. On the other hand, results were similar for both populations under DT regimes, suggesting that both populations can tolerate similar maximum temperatures as long as those occur only few hours a day.
T. pityocampa exhibits variable phenology across its distribution range with moths emerging later (after the warmest temperatures) in the southern and lower-altitude areas, and earlier (before the warmest temperatures) in the northern and high-altitude regions (Démolin, Reference Démolin1969; Huchon & Démolin, Reference Huchon and Démolin1970). Our results suggest that high summer temperatures can affect embryo survival, and probably impose strong natural selection. High mortality due to hot summer temperatures of both eggs (this study) and neonate larvae (Santos et al., Reference Santos, Paiva, Tavares, Kerdelhué and Branco2011b ) would then favour the natural selection of late-emerging individuals in regions where summer temperatures are high, possibly through the evolution of longer pupal diapause. Such selection pressure could be especially strong because each individual only lives for 1–3 days and thus reproduces immediately after adult emergence (Branco et al., Reference Branco, Paiva, Santos, Burban and Kerdelhué2017). Plastic or adaptive changes in phenology could thus be a way to overcome seasonal unfavourable conditions, and could be an efficient response to climate change for many organisms (Bale et al., Reference Bale, Masters, Hodkinson, Awmack, Bezemer, Brown, Butterfield, Buse, Coulson, Farrar, Good, Harrington, Hartley, Jones, Lindroth, Press, Symrnioudis, Watt and Whittaker2002; Root et al., Reference Root, Price, Hall, Schneider, Rosenzweig and Pounds2003; Musolin et al., Reference Musolin, Tougou and Fujisaki2010; Clusella-Trullas et al., Reference Clusella-Trullas, Boardman, Faulkner, Peck and Chown2014; Robinet et al., Reference Robinet, Laparie and Rousselet2015).
Other mechanisms, such as physiological and biochemical adaptations, can allow organisms to overcome unfavourable temperatures (Denlinger & Yocum, Reference Denlinger, Yocum, Hallman and Denlinger1998; Angilletta et al., Reference Angilletta, Niewiarowski and Navas2002). Studies looking for differential expression of target genes in eggs and larvae exposed to high temperatures might increase understanding of the underlying metabolic mechanisms. Such a perspective is now feasible thanks to the recent development of transcriptomic and genomic resources (Gschloessl et al., Reference Gschloessl, Vogel, Burban, Heckel, Streiff and Kerdelhué2014).
Development time
Although higher temperatures usually speed up metabolism and development in poikilothermic organisms, short exposure to extreme high temperature might have an opposite outcome. Indeed, we observed that egg development time increased with heat treatment, independently of the temperature regime applied (daily cycle or constant), at least when using between 3 and 12-days old embryos. For DT36, the time of egg development was almost 5 days longer than the control (25°C), and for CT40 eggs took almost four more days to hatch than the control. Similarly, longer egg development times with increasing temperatures and exposure time were observed in other Lepidoptera, namely Helicoverpa armigera, H. punctigera (Qayyum & Zalucki, Reference Qayyum and Zalucki1987) and Manduca sexta (Potter et al., Reference Potter, Davidowitz and Woods2011). Such results suggest a further negative effect of high temperatures by indirectly increasing exposure to natural enemies during the egg stage. However, it might also be adaptive, as such a delay allows the young larvae to hatch further in time from the heat wave, which would increase the survival of this highly sensitive stage. In fact in a previous study, it was found that significant mortality of PWP young larvae occurs at T max = 38°C (Santos et al., Reference Santos, Paiva, Tavares, Kerdelhué and Branco2011b ), whereas the threshold found here for the embryo was T max = 42°C.
Parasitoids
According to Hance et al. (Reference Hance, Baaren, Vernon and Boivin2007), both hosts and parasitoids are affected by extreme temperatures, and any effect on the host has consequences for the parasitoids within it. Still, it is possible that parasitoids respond differently to extreme conditions and could have different temperature threshold for survival. This is a relevant issue since different effects of climate changes on the phenology or survival of the two trophic levels might have consequences for their population dynamics by disrupting the biotic interactions. In our study, it was not possible to follow the effects of high temperatures on egg parasitoids despite their ecological interest, because parasitoid survival rates could not be estimated and the parasitoid stage that experienced the heat conditions could not be controlled. However, we did observe that a few parasitoids survived in the CT38 and DT36 conditions. Although these results are only preliminary, it seems that parasitoids may cope, at least partially, with these high temperatures.
Conclusions
Our study tested the effect of a wide range of high temperatures and allowed setting the lethal egg temperature for T. pityocampa in the case of short-term exposures. Further, differences were registered between a population with spring egg phenology (SP) and the typical PWP population with summer egg laying. A significant effect of simulated heat waves on egg developmental time was also observed. Considering potential effects of future climate warming scenarios, present findings suggest that environmental conditions in the southern regions may become less favourable to T. pityocampa populations that have the typical winter phenology due to the expected increase of summer temperatures. Shifts in phenology towards even later emergence may be further expected. Concerning the evolution of the SP, even though its embryo survival is not expected to be affected by spring temperatures, as they are likely to stay below the detrimental threshold, its fate under climate change may be threatened due to increased mortality of neonate larvae in summer (Godefroid et al., Reference Godefroid, Rocha, Santos, Paiva, Burban, Kerdelhué, Branco, Rasplus and Rossi2016). It is similarly possible that the current expansion of T. pityocampa observed at the northern edge of its distribution could be impeded if the frequency of summer heat waves increases as expected. Taking into account the intensity and the probability of occurrence of heat waves will be necessary in the future to forecast the evolution of populations under climate change.
Acknowledgements
We thank Liliana Vilas Boas, Catarina Tavares and Helena Santos for help with the field work and laboratory trials. We are grateful to Ruth Hufbauer for editing the English usage throughout the manuscript. This study was partly financed by Fundação para a Ciência e Tecnologia, FCT-MCES, Portugal, projects Pest-OE/AGR/UI0239/2011 and UID/AGR/00239/2013. It was also supported by Susana Rocha PhD scholarship with the reference SFRH/BD/90188/2012 (FCT-MCES).