Viruses and viral infection
A virus is composed of a nucleic acid molecule (RNA or DNA) and a protein shell. Some viruses also contain a lipid envelope outside the protein shell. Some of the most important and prominent aspects of viruses are cell tropism and host range (Ref. Reference Minton1).
The viral life cycle is a dynamic process that generally includes the interaction of viruses with cell surface receptors, endocytosis, intracellular transport, viral genome release, gene expression, assembly and progeny viral particle release (Ref. Reference Sun, He and Zhuang2). Viral evolution is affected by genetic modification and the acquisition of exogenous DNA or RNA (Ref. Reference Ibrahim3). After infecting host cells, some viruses coexist peacefully with hosts, while a few viruses cause acute or chronic infection of hosts (Ref. Reference Nomaguchi and Adachi4). For instance, the pandemic of severe acute respiratory syndrome coronavirus (SARS-CoV) that occurred in China in November 2002 attracted global attention. By July 2003, SARS-CoV had caused more than 8000 cases in 26 countries/regions. In December 2019, a virus named SARS-CoV-2 caused coronavirus disease 2019 (COVID-19). Shortly after the outbreak of COVID-19, it spread rapidly all over the world, causing a global pandemic and becoming a public health emergency (Refs Reference Wilder-Smith, Chiew and Lee5, Reference Li6). The environment around us can be altered by viruses, and we can be directly and/or indirectly influenced by their biological activities (Ref. Reference Adachi7). These viruses causing serious human and/or animal diseases will continue to pose a threat to global health and initiate terrible pandemics in the future.
In recent decades, a variety of antiviral drugs and treatment methods have been developed. Antiviral drugs can inhibit viral replication by blocking the key stage of the viral replication cycle, such as the anti-human immunodeficiency syndrome virus drug sifuvirtide. They can also inhibit the replication of viruses by interfering with the host immune response, such as interferon. An increasing number of natural compounds exert good antiviral effects in this way (Ref. Reference Gonçalves8). In addition, new directions have emerged in the treatment of antiviral diseases. Nanotechnology has been applied to the research and development of antiviral drugs. For example, nanoparticle tannins can be used to treat diseases caused by herpes simplex virus (Ref. Reference Galdiero9). Monoclonal antibody technology and CRISPR-Cas technology for antiviral infection are becoming increasingly mature (Refs Reference Ison10, Reference Read11). Viruses can be recognised by cell pattern recognition receptors, such as Toll-like receptors and RIG-I-like receptors, which further activate the innate immune system. These pattern recognition receptors share many downstream signalling molecules, which further cause the expression of inflammatory factors, innate immune factors and transcription factors (Ref. Reference Read11). In many downstream intermediates, p38 mitogen-activated protein kinase (MAPK) plays an important role in the signalling cascade induced by various extracellular stimuli, including viral infections. The infection of many viruses can activate the p38 signalling pathway, which makes the p38 pathway a promising target for the treatment of viral diseases.
p38 and p38 activation
MAPKs are Ser/Thr protein kinases that can phosphorylate many downstream targets to achieve highly specific cellular responses (Ref. Reference Martinez-Limon12). The p38 signalling pathway responds to a variety of external stimuli, including viral infections. It can be activated by hepatitis C virus (HCV), influenza A virus (IAV), herpes simplex virus (HSV) and other viruses. In mammals, a total of 14 MAPK members have been identified, which are divided into seven subgroups: p38 MAPK, extracellular-regulated kinase (ERK1/2), ERK5 and c-Jun-N-terminal kinase (JNK), as well as three atypical MAPKs ERK3/4, ERK7/8 and Nemo-like kinase (NLK).
The p38 MAPK family includes four subtypes: p38α (MAPK14), p38β (MAPK11), p38γ (MAPK12) and p38δ (MAPK13), which are encoded by four different genes (Ref. Reference Corre, Paris and Huot13). The four subtypes of p38 can be detected in human tissues, but their cellular expression profiles are different. p38α is ubiquitous in various cell types, especially monocytes, while the other three subtypes are expressed in certain tissues. p38β is mainly distributed in the brain, and p38γ is mainly distributed in the bones, while p38δ is mainly expressed in the pancreas, testis, small intestine and kidney.
The structure of p38α was determined by X-ray crystallography. p38α can be cocrystallised with an inhibitor, such as SB203580, or its substrate. p38β has a three-dimensional structure similar to that of p38α, but the relative orientations of its N- and C-terminal domains are different from those of p38α, resulting in a reduction in the p38 ATP binding pocket, which is possibly related to the selectivity of ATP-competitive inhibitor compounds. The activation loop conformation of p38γ is almost the same as the conformation of activated ERK2 (Ref. Reference Cuadrado and Nebreda14).
There are three pathways to activate p38 MAPK. The typical p38 MAPK activation pathway is the activation of the loop sequence Thr-Gly-Tyr, causing the double phosphorylation of p38 MAPK. The main protein kinases activating p38 are MAP kinase kinase 3 (MKK3) and MAP kinase kinase 6 (MKK6). All four subtypes of the p38 MAPK family can be phosphorylated by MKK6. Except for p38β, the remaining three subtypes can be phosphorylated by MKK3 (Ref. Reference Enslen, Raingeaud and Davis15). Moreover, MKK4, the main activator of JNK, can also activate p38. In addition to the typical p38 MAPK activation pathway, there is also an atypical p38 activation pathway involved in the direct binding of transforming growth factor activated kinase-1 binding protein-1 (TAB1) to p38α. TAB1 induces the self-phosphorylation of p38α, bypassing the need for upstream MAP2Ks. Another pathway activating p38 occurs in T cells, where p38 activation is mediated by ZAP-70 and is involved in the phosphorylation of p38α (possibly p38β) at tyrosine 323, which leads to autophosphorylation of p38α, followed by the phosphorylation of threonine 180 and tyrosine 182 (Ref. Reference Salvador16).
p38 MAPK participates in multiple cell responses. Its substrates include nuclear proteins, protein kinases associated with different processes, and a group of heterogeneous cytoplasmic proteins. These substrates regulate various processes, such as protein degradation and localisation, cytoskeletal dynamics, endocytosis, mRNA stability, cell migration and apoptosis (Ref. Reference Cuadrado and Nebreda14). In this review, we summarise how different viruses activate the p38 signalling pathway and the effects of activation of the p38 signalling pathway on viral replication and explore the potential application of the p38 signalling pathway as an antiviral therapy target.
Induction of p38 activation by viral infection
To respond to extracellular stimuli, such as viral infection, cells have evolved many kinases and related signalling pathways, including the p38 signalling pathway. We summarise a large number of reports that detail how the regulation of the p38 MAPK signalling pathway plays important roles in viral replication. The activation pathways of p38 induced by different viruses are shown (Fig. 1). We describe the characteristics and relationship between different viruses and the p38 signalling pathway by dividing these viruses into RNA viruses and DNA viruses.
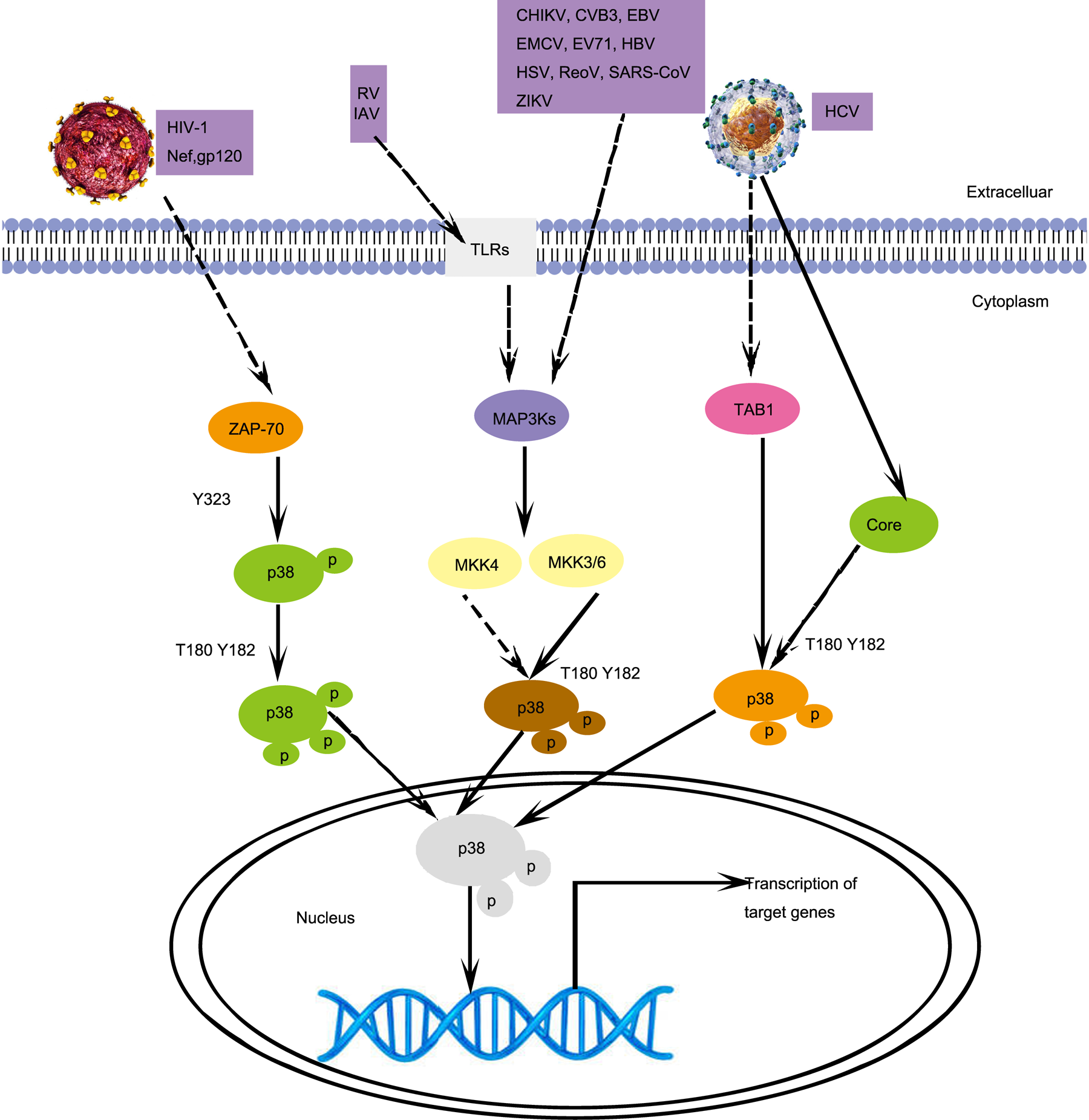
Fig. 1. Different p38 activation signalling pathways induced by viral infections. HIV-1 infection activates p38 in T cells, which is mediated by ZAP70. RV, IAV, CHIKV, CVB3, EBV, EMCV, EV71, HBV, HSV, RaoV, SAS-CoV, and ZIKV infections activate p38 by activating MKK4 or MKK6. HCV infection activates p38, which is mediated by TAB1. CoV, Coronavirus; CVB3, coxsackievirus B3; DENV, dengue virus; EBV, Epstein–Barr virus; EMCV, encephalomyocarditis virus; EV71, enterovirus 71; HIV-1, human immunodeficiency virus type 1; HSV, herpes simplex virus; ReoV, reovirus; ZIKV, Zika virus; MAP3Ks, mitogen-activated protein kinase kinase kinases; TAB1, TAK1-binding proteins 1; ZAP-70, Zeta chain of T-cell receptor-associated protein kinase 70. Solid arrows, direct interactions. Dashed arrows, indirect interactions.
RNA viruses
Flaviviridae: hepatitis C virus (HCV), Zika virus (ZIKV) and dengue virus (DENV)
Millions of people around the world are infected with Flaviviridae each year. There are more than 100 members in the family Flaviviridae, and they are highly similar in terms of genome structure and life cycle. The viral genome is a 9.6–12.3 kb positive-strand RNA (Ref. Reference Zhang, Rong and Li17). HCV, ZIKV and DENV are important members of Flaviviridae in medicine.
HCV, ZIKV or DENV infection can activate the intracellular p38 signalling pathway. CD81 and LDLR are receptors on T lymphoma cells that have been found to interact with the HCV E2 protein to induce the upregulation of phosphorylated p38 (P-p38) expression (Ref. Reference Zhao18). In macrophages, the p38 signalling pathway is involved in HCV infection, and the interaction between the HCV core protein and gC1qR, instead of TLR2, TLR3 and TLR4, is detected, resulting in increased A20 expression (Refs Reference Song19, Reference Moorman20). T lymphocytes taking HCV non-enveloped particles can increase the phosphorylation level of p38 (Ref. Reference Serti21). The above studies suggest that there are manifold mechanisms involved in the activation of the p38 MAPK signalling pathway in HCV infection. After ZIKV infects Müller cells, a variety of inflammatory signalling pathways are activated. The inhibition of p38 activation effectively prevents the inflammation induced by ZIKV (Ref. Reference Zhu22). In contrast, the proinflammatory response induced by DENV is much lower. In DENV-infected endothelial cells, the non-structural protein NS1 can induce the activation of p38 MAPK through the classical MKK3/6 pathway (Ref. Reference Barbachano-Guerrero, Endy and King23).
Orthomyxoviridae: influenza A virus (IAV)
IAV is classified into the family Orthomyxoviridae. The overall viral particle is composed of hemagglutinin, neuraminidase, nuclear protein, RNA, matrix protein and envelope protein (Ref. Reference To and Torres24). There are an estimated 291 000–646 000 respiratory deaths attributable to seasonal influenza each year worldwide (Ref. Reference Chow, Doyle and Uyeki25). Emerging IAV can cause global pandemic and zoonotic infections, such as H5N1 and H7N9 (Refs Reference Meineke, Rimmelzwaan and Elbahesh26, Reference Choi27). Currently, drugs approved by the FDA for IAV treatment include cap-dependent endonuclease inhibitors, neuraminidase inhibitors and adamantanes.
IAV infection triggers the p38 MAPK signalling pathway, which causes p38 phosphorylation, further induces the activation of the antiapoptotic protein Bcl-2, and finally makes host cells susceptible to virus-induced apoptosis (Ref. Reference Nencioni28). The crucial event for the entry and replication of IAV is TLR4-mediated p38 activation (Ref. Reference Börgeling29). IAV interacts with TLR4 on the cell membrane to activate downstream MyD88 and to induce the activation of p38, which is the determinant of IAV infection (Ref. Reference Marchant30). IAV infection also activates p38 MAPK by promoting the production of cell reactive oxygen species (ROS). Moreover, during IAV infection in vitro, the activities of p38 and ERK are regulated by NADPH oxidase 4 (NOX4), resulting in an increase in ROS (Refs Reference Wu31, Reference Jaulmes32).
Coronaviridae: severe acute respiratory syndrome coronavirus (SARS-CoV) and SARS-CoV-2
Coronavirus (CoV) belongs to the family Coronaviridae. The genome of CoV is a positive-stranded RNA, which is the largest (27–32 kb) among the RNA viruses. SARS-CoV, which first appeared in 2002, caused a global outbreak. It is the causative agent of SARS, with 8096 cases and 774 deaths reported worldwide, corresponding to a 9.6% fatality rate (Ref. Reference Totura and Baric33). SARS-CoV-2 was first discovered in December 2019, causing the outbreak of COVID-19 around the world. Currently, SARS-CoV-2 is still an urgent public health event.
SARS-CoV infection promotes the phosphorylation of MKK3/6 in Vero E6 cells and further upregulates p38 expression. In addition, a papain-like protease (PLpro) encoded by the SARS-CoV genome can induce p38 activation. In SARS-CoV-infected cells, the upregulation of SARS-CoV 3a protein expression can activate MKK3/6 (Ref. Reference Padhan34). Another SARS-CoV protein, 7a, can also stimulate p38 activation, but the exact mechanism is still unclear. Angiotensin I-converting enzyme 2 (ACE2) is the receptor for both SARS-CoV and SARS-CoV-2 entry into host cells. The main function of ACE2 is to transform angiotensin II (ANGII) to angiotensin 1–7 (ANG1–7). ANGII binding to ACE2 is able to induce p38 phosphorylation, while ANG1–7 binding to the Mas receptor inhibits the activation of p38 MAPK. Therefore, SARS-CoV-2 infection causes excessive activation of p38 MAPK after viral entry (Ref. Reference Grimes and Grimes35). A recent report on SARS-CoV-2 showed that when p38δ, p38γ and MAP2K3 were knocked down by siRNA in A549 cells expressing ACE2, the replication of SARS-CoV-2 was significantly suppressed (Ref. Reference Grimes and Grimes35).
Retroviridae: human immunodeficiency virus (HIV)
The genome of HIV is two identical single-stranded RNA molecules, each of which is approximately 9.2–9.8 kb in length. The particle diameter of HIV is 100 nm, and the outermost layer is the envelope protein (Ref. Reference Fanales-Belasio36). There are two HIV subtypes, HIV-type 1 (HIV-1) and HIV-type 2 (HIV-2). HIV-1 has a higher replication capacity and a higher transmission rate than HIV-2. HIV-1 is currently the dominant epidemic type of AIDS in the world, while symptoms caused by HIV-2 infection are relatively mild. HIV-2 is mainly distributed in some areas of West Africa and Central Africa (Ref. Reference Eberle and Gürtler37).
The HIV-1 accessory protein Nef activates p38 in T lymphocytes. The envelope protein Gp120 of HIV-1 induces the activation of p38 by binding to CXCR4, where CD4 is necessary. p38 may be activated through a non-classical activation pathway, which requires the participation of ZAP-70, followed by the phosphorylation of tyrosine 323 at p38 and the autophosphorylation of threonine 180 and tyrosine 182 (Refs Reference Furler and Uittenbogaart38, Reference Yuan39).
Reoviridae: reovirus (ReoV) and rotavirus (RV)
Reoviridae virus particles have icosahedral structure, spherical appearance and no envelope. The viral genome is linear double-stranded RNA. Although viral infection usually does not cause fatal diseases and is not discovered clinically, up to 50–60% of adults are infected with ReoV (Ref. Reference Selb and Weber40). Importantly, ReoV has an oncolytic effect and is being developed as an anticancer agent (Ref. Reference Errington41). RV is the leading cause of gastroenteritis in infants and young children. RV infection causes approximately 900 000 infant deaths worldwide each year, most of which occur in developing countries. Approximately 2.7 million cases of RV infections occur in the United States every year (Ref. Reference Offit42). Currently, the live-attenuated oral rotavirus vaccines approved globally are Rotarix and RotaTeq (Ref. Reference Bruijning-Verhagen and Groome43).
AICAR, a selective activator of AMP-activated protein kinase (AMPK), can stimulate the phosphorylation of p38 MAPK in Vero cells infected by avian reovirus (ARV). However, mTORC1, an important mediator of AMPK affecting cell function, is not a key factor in p38 activation. The inhibition of AMPK decreases the phosphorylation of MKK3/6 and p38 and restricts ARV replication. These reports show that the activation of the p38 classical pathway by AMPK-related mechanisms may be significant for ARV replication in Vero and DF-1 cells (Ref. Reference Ji44). Activation of the Ras signalling pathway can make recombinant ReoV grow more effectively in transformed cells. ReoV is related to Ras/RalGEF/p38 signalling pathways. Most RV strain infections, such as SA11, CRW-8, Wa and UK, can activate p38 to different degrees (Ref. Reference Holloway and Coulson45). RV protein NSP4 binding to TLR2 located on the cell membrane activates p38 MAPK in PMA-treated THP-1 cells within 15 min (Ref. Reference Ge46). However, the innate immune response of RV-infected macrophages depends on mitochondrial antiviral signalling protein (MAVS) rather than p38 MAPK (Ref. Reference Di Fiore, Holloway and Coulson47).
Togaviridae: Chikungunya virus (CHIKV)
CHIKV is classified into the family of Togaviridae. The whole genome of CHIKV is 11 805 bp in length and encodes seven proteins. CHIKV is the pathogen of Chikungunya fever, an acute infectious disease transmitted by Aedes mosquitoes (Refs Reference Weaver and Lecuit48, Reference Khan49). The main symptoms of Chikungunya fever are rash, myalgia, severe joint pain, fever and headache. Chikungunya fever re-emerged in tropical regions, such as Asia and Africa, 10 years ago (Ref. Reference Silva and Dermody50). Currently, there are still no targeted drugs or specific vaccines.
Although there have been few reports investigating the relationship between CHIKV and p38 MAPK, some studies show that p38 is a key protein during CHIKV infection. CHIKV infects macrophages and stimulates the activation of p38 in a time-dependent manner. The interaction between CHIKV NSP2 and phosphorylated p38 was detected in macrophages, which may have important implications for TNF-mediated inflammatory responses (Ref. Reference Nayak51). In recent years, the alkaloid berberine has been found to effectively suppress CHIKV replication and reduce CHIKV-induced p38 MAPK signal transduction (Ref. Reference Varghese52).
Picornaviridae: coxsackievirus B3 (CVB3), enterovirus 71 (EV71) and encephalomyocarditis virus (EMCV)
Picornaviridae is a non-enveloped, positive-stranded icosahedral structure with a genome length of 6.7–10.1 kb. Picornaviruses are ubiquitous and distributed worldwide, posing a threat to the health of humans and domestic animals (Ref. Reference Zell53). CVB3 infection in humans leads to various diseases, such as aseptic meningitis and myocarditis (Ref. Reference Sin54). Millions of people worldwide are infected with EV71, which causes outbreaks of hand, foot and mouth disease (HFMD) (Ref. Reference Xia55). EMCV is a zoonotic pathogen distributed all over the world, not only causing acute infectious diseases characterised by myocarditis and encephalitis but also leading to diabetes, neuropathy and reproductive diseases in many mammals (Ref. Reference Wei56).
It was reported that CVB3, EV71 and EMCV infection can induce the activation of the p38 signalling pathway. When miRNA-21 expression was upregulated in HeLa cells, a decrease in CVB3 release was observed, and apoptosis was reduced, proving that miRNA-21 regulated CVB3 replication through the p38 signalling pathway. SB203580 is an inhibitor of p38 MAPK and effectively inhibits p38 activity in cardiomyocytes, as anticipated, thereby blocking the phosphorylation of downstream MK2 in the early and late stages of CVB3 infection (Ref. Reference Jensen57). In addition, two studies on anti-CVB3 drugs in 2019 showed that the addition of luteolin and Scutellaria baicalensis Georgi extracts (SBE) can reduce the replication of CVB3. SBE reduced viral replication by inhibiting the expression of p38, and luteolin played a partial role by inhibiting the formation of P-p38 and the nuclear translocation of NF-κB, thereby resulting in the inhibition of inflammatory cytokines in CVB3-infected cells (Refs Reference Wu58, Reference Fu59).
EV71 infection promotes the expression of MKK3/MKK6 and induces the expression of P-p38 (Ref. Reference Peng60). Moreover, the replication of EV71 instead of viral particles can induce the phosphorylation of p38 (Ref. Reference Zhang61). Zhu et al. found that the expression levels of ERK, JNK and p38 in PBMCs from EV71-infected severe patients were obviously higher than those of mild patients, which was similar to the expression patterns of TLR3, TLR4, TLR7 and TLR8. These results imply that the high expression levels of TLR3, TLR4, TLR7 and TLR8 in severe EV71 infection increase the production of cytokines by the MAPK pathway (Ref. Reference Zhu62).
dsRNA is an intermediate substance of EMCV replication. Both EMCV infection and dsRNA stimulation induce the phosphorylation of MKK3/6 and further activate p38 in mouse fibroblasts (Ref. Reference Iordanov63). After infection with the recombinant EMCV virus vEC9 or transfection with EMCV leader protein in HeLa cells, the p38 signalling pathway is activated, which is not catalysed by typical MAPKKK or MAPKK (Ref. Reference Porter, Brown and Palmenberg64).
Filoviridae: Ebola virus (EBOV)
EBOV is a member of the Filoviridae family, and its genetic material is a non-segmented negative-stranded RNA with a size of approximately 19 kb. EBOV particles are long, filamentous structures with a diameter of approximately 80 nm and are enveloped (Ref. Reference Baseler65). EBOV infection leads to severe haemorrhagic fever with a mortality rate of up to 90%. From 2014 to 2016, the EBOV outbreak caused more than 11 000 deaths in West Africa (Ref. Reference Zawilińska and Kosz-Vnenchak66).
Recently, several reports studied the relationship of p38 MAPK and EBOV infection and indicated that EBOV infection can induce p38 phosphorylation (Refs Reference Johnson67, Reference Halfmann, Neumann and Kawaoka68). For example, the p38 signalling pathway was activated in both macrophages and dendritic cells infected with EBOV (Ref. Reference Johnson67).
DNA viruses
Herpesviridae: herpes simplex virus (HSV), Epstein–Barr virus (EBV) and varicella zoster virus (VZV)
Herpesviruses are widely distributed in nature and are divided into three subfamilies according to their biological characteristics. Representatives of the α-herpesvirus subfamily are HSV and VZV, and EBV is a member of the γ-herpesvirus subfamily. According to different antigens, HSV is divided into two serotypes, namely, HSV-1 and HSV-2. The genome homology of the two HSV subtypes is 50% (Ref. Reference Sharma, Mobeen and Prakash69). HSV infection can cause oral and genital diseases, most of which are latent infections. In severe cases, HSV can invade the central nervous system and damage the brain, causing herpetic encephalitis (Refs Reference Heineman70, Reference Song71). Over 90% of adults worldwide are infected with EBV. EBV is the first virus associated with epithelial and lymphoid malignancies (Ref. Reference Auerbach and Aguilera72), such as childhood lymphoma, Burkitt lymphoma, Hodgkin's lymphoma and nasopharyngeal carcinoma (Ref. Reference Phan73). VZV can easily infect children, causing chickenpox and herpes zoster (Ref. Reference Baird74).
Several reports have shown that p38 MAPK is involved in HSV, EBV and VZV infection. HSV-1 infection activates JNK/p38 MAPKs via the upstream activator mitogen-activated protein kinase kinase 4/stress-activated protein/ERK kinase 1 (MKK4/SEK1) to further stimulate AP-1 (Refs Reference Karaca75, Reference Zachos, Clements and Conner76). CXCR3 ligand expression was mediated by P-p38 in human cervical epithelial cells treated with HSV-2 ICP4. The expression of HSV-1 ICP27 activates the p38 pathway and subsequently causes apoptosis. HSV-1 infects mouse microglia to produce ROS by NADPH oxidase, and the redox signal generated by reactive oxygen species induces p38 phosphorylation (Ref. Reference Hu77).
PKCθ, a new member of protein kinase C (PKC), regulates MKK4 activation, thereby activating p38 MAPK and then promoting the EBV cleavage cycle by inducing autophagy (Ref. Reference Gonnella78). Treating EBV-transformed B cells with sorafenib, a novel multitarget drug for tumour treatment, can activate ROS in cells, and ROS can continuously phosphorylate JNK/p38-MAPK, ultimately inducing cell apoptosis. These results indicate that sorafenib can promote the apoptosis of EBV-transformed B cells through the JNK/p38-MAPK pathway (Ref. Reference Park79).
VZV infection activates p38 and ERK1/2, but its tegument proteins associated with viral entry and release are not sufficient to activate MAPK. This activation process seems to require viral gene expression or VZV-mediated cell gene expression (Ref. Reference Rahaus, Desloges and Wolff80). ORF12, a tegument protein of VZV, is not necessary for the growth of VZV, but it can activate a variety of intracellular signalling pathways. ORF12 and ORF61 can activate the p38 MAPK signalling pathway (Refs Reference Rahaus, Desloges and Wolff81, Reference Liu82).
Hepadnaviridae: hepatitis B virus (HBV)
HBV is classified into the Hepadnaviridae family, and the complete HBV particle diameter is approximately 42 nm (Ref. Reference Kang83). HBV infection has caused global public health problems. There are 257 million chronic HBV patients and more than 880 thousand deaths from HBV-related diseases every year (Refs Reference Horng84, Reference Karayiannis85).
HBV infection was reported to activate the p38 signalling pathway. Among all HBV genome-encoding proteins, only the HBx protein can upregulate the expression of special AT-rich binding protein-1 (SATB1) by activating the p38 and ERK pathways (Ref. Reference Tu86). The expression of HBcAg and HBx in HBV activates p38, ERK1/2 and NF-κB to promote the secretion of IL-6 in hepatocytes (Refs Reference Chen87, Reference Xiang88).
Interfering with p38 activation and its effect on viral replication
p38 activation and the p38 signalling pathway can affect and regulate many biological functions. p38 was revealed to be associated with the stress response, inflammation, and tumour and cell development. Disturbing the p38 signalling cascade has serious pathological consequences. A large number of studies have shown that regulating p38 activation and signalling pathways has important significance for virus replication. We summarise the pathways by which different viruses induce the activation of the p38 signalling pathway in the form of a table (Table 1). In addition, we summarise the effects of the activation of the p38 signalling pathway on virus replication (Table 2).
Table 1. Summary on signal pathways and effects of viral infection-induced p38 activation

Table 2. Summary of the role of the p38 signalling pathway in viral infection

At present, p38 kinase inhibitors are the most widely used inhibitors in research on the p38 signalling pathway, and the field is becoming increasingly mature. Common inhibitors include SB203580, BIRB796 and PD169316. More than 20 drug candidates among p38 kinase inhibitors have entered the stage of clinical trials and are actively being developed for the treatment of rheumatoid arthritis, chronic obstructive pulmonary disease and other diseases (Ref. Reference Haller89). In addition, the p38 signalling pathway has also become a target for cancer treatment (Ref. Reference Yong, Koh and Moon90). Other pathway inhibitors, such as ferrostain-1 (Fer-1), a specific inhibitor of iron death, have recently been found to inhibit the p38 signalling pathway to reduce cognitive impairment in epileptic rats (Ref. Reference Ye91). Next, this review focuses on the inhibition of various viruses after the p38 signalling pathway is blocked.
RNA viruses
Flaviviridae: HCV, ZIKV and DENV
Research on the relationship between HCV and the p38 signalling pathway is more in-depth. The p38 signalling pathway plays an important role in HCV-induced liver cancer. Studies have shown that the HCV core protein can be catalysed by phosphorylated p38α, which promotes the oligomerisation of the HCV core protein and viral assembly. The treatment of the p38 kinase inhibitor SB203580 or knocking down p38α is found to reduce the expression of the HCV core protein and significantly inhibit the replication of HCV (Ref. Reference Cheng92). In ZIKV-infected Müller cells, p38 MAPK is a key mediator of ZIKV-induced inflammation, which provides a new perspective for understanding ZIKV infection-induced retinopathy and the treatment of ZIKV infection. Blocking p38 MAPK is discovered to effectively reduce the inflammatory phenotype of Müller cells after ZIKV infection, such as the decrease of IL-6 mRNA expression (Ref. Reference Zhu22). DENV infection induces the phosphorylation of p38α in Huh 7 cells. The phosphorylation of eIF4E is further induced by the MNK1 protein, which may be related to the translation of specific mRNAs regulated by DENV (Ref. Reference Roth93). In a mouse model of DENV infection, the treatment of SB203580 improves the two haematological parameters of leukopenia and thrombocytopenia. In addition, tumour necrosis factor α, caspase 9, caspase 8 and caspase 3 proteins are significantly lower than those in the control group (Ref. Reference Sreekanth94).
Orthomyxoviridae: IAV
The expression of the metalloproteinase MMP9 is increased in the lung tissue of mice infected with IAV, suggesting that IAV infection could cause destruction of the lung epithelial barrier. Moreover, p38 MAPK has been found to be possibly indispensable for the elevation of MMP9 (Ref. Reference Shen95). The p38 inhibitor SB203580 reduces IAV replication, viral ribonucleoprotein (vRNP) output and apoptosis (Ref. Reference Nencioni28). Another study showed that the inhibition of p38 in macrophages infected with H5N1 and H7N7 can result in a decrease in the content of cytokines such as IFN-β and IFN-λ1 (Ref. Reference Hui96). More than 90% of the viruses partially or completely rely on p38 signalling to induce gene expression. p38 regulates the expression of interferon-stimulated genes by phosphorylating STAT1 at serine 727. Blocking p38 MAPK in vivo will greatly reduce the expression of virus-induced cytokines and simultaneously decrease the virus titre, thereby protecting mice from fatal infection (Ref. Reference Börgeling29).
Coronaviridae: SARS-CoV and SARS-CoV-2
The increased level of P-p38 in the CoV-infected endothelium has been found to be associated with endothelial cell apoptosis, arterial thrombosis and platelet aggregation (Refs Reference Zhang97, Reference Chen98). The 3a protein of SARS-CoV can induce p38 phosphorylation, which further upregulates the expression of p53, a downstream substrate of p38. Activated p53 further promotes the nuclear transcription of the bax gene. Bax forms a polymer and then inserts into the outer mitochondrial membrane, resulting in mitochondrial potential loss and delivery of cytochrome c, which further induces the activation of protease caspase-9 and ultimately affects cell apoptosis (Ref. Reference Padhan34). Meanwhile, SB203580 can slightly inhibit the formation of CPEs in SARS-CoV-infected Vero E6 cells (Ref. Reference Mizutani99). It has been reported that severe COVID-19 patients have obvious thrombosis, which is related to the direct involvement of the vascular endothelium in SARS-CoV-2 infection. In addition, the activation of p38 MAPK induces receptor-mediated endocytosis, thereby facilitating SARS-CoV-2 entry (Ref. Reference Zhou100).
Retroviridae: HIV
Three studies analysed the mechanism of HIV-1 infection and found that p38 MAPK inhibition can reduce the replication of virus in HIV-1-infected T lymphocytes and monocytes/macrophages, which may be due to blocking the long terminal repeat sequence of HIV-1, impairing reverse transcription and destroying precursor integration (Refs Reference Cohen101, Reference Muthumani102). When T cells are infected with HIV-1, the accessory protein Nef of HIV-1 can induce the phosphorylation of p38, and its proline-rich motif (PXXP) promotes programmed death-1 (PD-1) transcription upregulation. Nef-induced p38 activation is necessary for PD-1 upregulation. As a result, the upregulation of PD-1 means that not only may the virus not be cleared, but it may also cause the virus to exist for a long time. In addition, HIV-1 infection can lead to T lymphocyte apoptosis. Moreover, p38 activation is required for p53 phosphorylation induced by the HIV-1 envelope glycoprotein complex (Env). Perfettini et al. found that p38 phosphorylated at T180/Y182 colocalised with p53 phosphorylated at S46, and p53 is necessary for HIV-1 envelope-induced apoptosis. p38-mediated p53 phosphorylation is the key to HIV-1 Env-induced apoptosis (Ref. Reference Perfettini103).
Reoviridae: ReoV and RV
In Ras-transformed cells, SB203580 inhibits p38 activity and effectively intervenes in reovirus protein synthesis but does not influence host protein synthesis. Holloway et al. reported that blocking the JNK or p38 pathway can greatly reduce RV-induced c-Jun expression, resulting in a subsequent reduction in AP-1 transcription and viral titre. However, after RV infects Caco-2 and MA104 cells, p38 did not affect viral entry or the production of viral structural proteins (Ref. Reference Holloway and Coulson45). In addition, Jafri et al. also indicated that the addition of p38 and ERK1/2 MAPK inhibitors to RV-infected cholangiocytes led to reduced replication of the virus (Ref. Reference Jafri104).
Togaviridae: CHIKV
Nayak et al. found that the use of the p38 inhibitor SB203580 can reduce CHIKV infection in macrophages. Treatment with P-p38- and P-JNK-specific inhibitors decreased the protein level of TNF in CHIKV-infected macrophages. Moreover, CHIKV induced the production of P-IRF-3, a key transcription factor of macrophages, which possibly depended on p38 and JNK MAPK (Ref. Reference Nayak51).
Picornaviridae: CVB3, EV71 and EMCV
CVB3, EV71 and EMCV infection can induce the activation of the p38 signalling pathway. When the p38 signalling pathway was blocked, the replication of the three viruses was inhibited, and the release of virus particles was reduced (Refs Reference Peng60, Reference Si105). Relatively, the increase in P-p38 promoted virus replication (Ref. Reference Si105). The p38 signalling pathway is widely recognised, mainly due to its inflammatory response. CVB3, EMCV and EV71 infection can induce an inflammatory response and IL-6 expression through the p38 signalling pathway (Refs Reference Iordanov63, Reference Cao106). Jensen et al. found that p38 signalling did not directly control apoptosis in CVB3-infected cardiomyocytes, but this process was intervened by the ERK5 and ERK1/2 pathways. p38 is a key factor in the necrosis pathway, and its phosphorylation induces cardiomyocyte necrosis (Ref. Reference Jensen57). Another study showed that when RD cells were infected with EV71, p38 phosphorylation was observed and enhanced the relocation of hnRNP A1. Eps15 is the target protein of p38, which is very important for virus replication and can regulate intracellular transport. When p38 inhibitors were used, Eps15 phosphorylation was inhibited (Refs Reference Chen107, Reference Leong, Ong and Chu108). In mouse macrophages infected by EMCV, the expression levels of cyclooxygenase-2 (COX-2) and PGE2 were attenuated due to the inhibition of p38 and JNK, but the expression of inducible NO synthase (iNOS) was not suppressed (Ref. Reference Steer109). Another interesting study showed that the leader protein of EMCV affected the phosphorylation of nucleoporins or Nups, including hyperphosphorylation of Nup62, Nup153 and Nup214, by activating p38 and ERK. Nup phosphorylation levels were significantly inhibited when p38 and ERK inhibitors were used together, suggesting that the p38 and ERK pathways combined to affect EMCV leader protein-induced hyperphosphorylation of Nups affected nuclear transport (Ref. Reference Porter, Brown and Palmenberg64).
Filoviridae: EBOV
It was reported that when p38 MAPK was inhibited by SB202190, the entry of EBOV GP into MDDCs was blocked, which meant that the replication of EBOV may be blocked in MDDCs due to the restriction of EBOV entry. In addition, the secretion of cytokines, including MIP-1A, MIP-1B and RANTES, was also inhibited (Ref. Reference Johnson67). The VP24 protein of Zaire ebolavirus (ZEBOV) can block the IFN-β-mediated phosphorylation of p38α in HEK293 cells to interfere with the p38 MAPK pathway, which was not observed in HeLa cells, suggesting that the inhibition of ZEBOV on the p38 MAPK pathway was cell type-dependent (Ref. Reference Halfmann, Neumann and Kawaoka68).
DNA viruses
Herpesviridae: HSV, EBV and VZV
Activation of the p38 signalling pathway is very important in the viral life cycle. In virus-infected cells, p38-mediated expression of inflammatory cytokines, such as IL-6, IL-8 or related transcription factors, was triggered (Refs Reference Rahaus, Desloges and Wolff80, Reference Eliopoulos110, Reference Desloges111). When the p38 signalling pathway was blocked, viral replication was inhibited, and the expression levels of some viral proteins related to p38 phosphorylation also decreased. For example, when the p38 signalling pathway was blocked, the expression levels of the EBV-encoded proteins BZLF1 and BGLF2 decreased (Refs Reference Adamson112, Reference Liu and Cohen113). In contrast, when the p38 signalling pathway was activated, the expression levels of some viral proteins increased. For example, after HSV-2 activates p38, P-p38 further phosphorylates C/EBP-β, and then C/EBP-β combines with the CXCL9 promoter to increase the protein content of CXCL9 in human cervical epithelial cells. p38 was involved in the expression of CXCL9. After the secretion of CXCL9, activated CD4+ T cells can migrate and may be recruited to the viral infection site to play essential functions (Ref. Reference Huang114). In addition, some studies have shown that EBV infection promotes apoptosis and autophagy by activating p38 (Ref. Reference Gonnella78).
Hepadnaviridae: HBV
IL-6 is one of the most significant cytokines and is found to participate in the formation of hepatitis and hepatocellular carcinoma (Ref. Reference Xia55). According to previous reports, HBcAg can induce the upregulation of IL-6 expression in hepatocytes and hepatoma cells in many ways, such as NF-κB, ERK and p38 MAPK (Ref. Reference Chen87). Another HBV protein, middle s protein (MHBs), also leads to an increase in IL-6 expression levels by activating NF-κB and p38 MAPK. When p38-specific inhibitors are treated, HBV antigen secretion is reduced, and HBV replication is inhibited. HBV infection was observed to activate homeobox A10. In the later stage of HBV infection, homeobox A10 interacts with activated p38 and recruits SH2 tyrosine phosphatase 1 (SHP-1) to catalyse p38/STAT3 dephosphorylation, thereby inhibiting HBV replication (Ref. Reference Yang115).
Conclusion
There are many kinds of viral diseases, and many of them infect humans. The current treatments for virus infections are still limited, but the emergence of new virus and antiviral-resistant strains poses a serious threat to human health. Therefore, it is imperative to seek effective treatments and strategies against viral infections.
The survival of the virus completely relies on the host cell. Therefore, antiviral therapy targeting host cells can effectively restrict the virus. By analysing the key cellular processes utilised in most viral infections, kinases are revealed to play an important role in virus-infected host cells. Exploring the relationship between kinases and viral infections will be a new approach to discover ideal antiviral targets and related agents.
MAPKs, a very important family of kinases, mediate multiple cellular responses by multiple extracellular stimuli, including virus infection. p38 MAPK is one of the four main members of the MAPK family (Ref. Reference Zarubin and Han116). The p38 pathway has become an intermediate hub involved in antiviral innate immunity and inflammation pathways. Studying the relationship between p38 and virus infection is of great significance for understanding potential immune regulation and developing new intervention strategies. In recent decades, research on p38 has extended to many aspects in addition to inflammation-related responses, including the dual roles of p38 in tumorigenesis and the regulation of synaptic plasticity or glucose metabolism. However, there are still many problems to be further investigated, especially the specific molecular events in signal transduction. Which viral proteins can activate or inactivate p38 MAPK during viral infections? How do viral proteins affect p38 MAPK activation? How does phosphorylated p38 activate downstream effector proteins to produce related effects? All these questions still need to be further explored. A recent study on the global phosphorylation landscape of SARS-CoV-2 infection shows that pharmacological blockade of p38 activation could be a promising anti-SARS-CoV-2 strategy and that p38 MARK inhibitors might have great potential against SARS-CoV-2.
In conclusion, to better understand the relationship between p38 MAPK and viral infection, we summarise the viruses that can induce the activation of the p38 signalling pathway on the basis of previous studies. We seek to identify the pathways by which different viruses activate the p38 signalling pathway and elaborate on the impact of the activation of the p38 signalling pathway on viral replication to provide relevant information for the treatment of viral diseases.
Acknowledgements
This work was supported by grants from the joint research fund of the National Science Fund of China – Science and Technology Development Fund of Macau SAR (No. 32161160303 for NSFC and 0010/2021/AFJ for FDCT), the National Science Fund of China (Nos. 31872239 and 81630091), the Foundation for Innovative Research Groups of the Hubei Natural Science Foundation (No. 2020CFA015), and the Fundamental Research Fund for the Central Universities of China (No. 2042021kf0219).
Conflict of interest
None.