Introduction
Two non-exclusive mechanisms resulting from population exposure to environmental unpredictability are microevolutionary responses to selection and phenotypic plasticity (Charmantier et al., Reference Charmantier, McCleery, Cole, Perrins, Loeske, Kruuk and Sheldon2008). Phenotypic plasticity can be defined as the ability of a single genotype to produce different phenotypes according to the environment that it experiences (Ernande & Dieckmann, Reference Ernande and Dieckmann2004), as opposed to directional selection that favours the reproduction and survival of individuals possessing a particular trait over others. Hypothetically, when environmental variation occurs in predictable patterns, selection can favour the canalization of compromise phenotypes despite the accumulation of phenotypically cryptic genetic variance (Flatt, Reference Flatt2005; Fordyce, Reference Fordyce, Ananthakrishnan and Whitman2005). The role of phenotypic plasticity in evolution has been a contentious issue and has generated a debate over whether plasticity shields genotypes from selection or generates novel opportunities for selection to act (Ghalambor et al., Reference Ghalambor, McKay, Carroll and Reznick2007). Nevertheless, partial evidence has been gathered to support the notion that natural selection can favour plasticity by acting on different phenotypes in different environments (Van Buskirk & Relyea, Reference Van Buskirk and Relyea1998). In support of the above, it has been postulated that the accumulation of phenotypically neutral genetic variance and phenotypic accommodation of stress-induced effects, frequently experienced in heterogeneous environments, together with the inheritance of stress-induced modifications, ensures the evolutionary persistence of stress–response strategies and provides a link between individual adaptability and evolutionary adaptation (Badyaev, Reference Badyaev2005).
Anastrepha ludens (Loew) is a polyphagous tephritid fruit fly pest that exploits a wide range of hosts (Aluja, Reference Aluja1994; Norrbom, Reference Norrbom2003). It is distributed from northern Mexico to Costa Rica (Norrbom, Reference Norrbom2003), from sea level to 2000 m altitude (Birke et al., Reference Birke, Guillén, Midgarden, Aluja and Peña2013). Even though the fruits attacked by this fly can vary among regions, there appears to be little genetic variation among populations (Pecina-Quintero et al., Reference Pecina-Quintero, López-Arroyo, Loera Gallardo, Pons, Hernández, Mayek Pérez, Hernández Delgado, Rull and Aluja2009; Frey et al., Reference Frey, Guillén, Frey, Samietz, Rull and Aluja2013), and there is no evidence of reproductive isolation between populations stemming from different host species that are separated by over 1000 km in Mexico (Aluja et al., Reference Aluja, Rull, Pérez-Staples, Díaz-Fleischer and Sivinski2009).
One of the two putative ancestral hosts of A. ludens is yellow chapote, Casimiroa greggii Watson, a rutaceous plant native to northeastern Mexico that grows in riparian habitats along canyons in the Sierra Madre Oriental (the other one being Casimiroa edulis, also Rutaceae). The main fruiting season for this species spans from late March to August, with occasional precipitation-driven, off-season flowering, producing some fruit at other times such as October (Plummer et al., Reference Plummer, McPhail and Monk1941). Regionally, fruiting can be spatially variable with some areas producing no fruit and others large crops (Plummer et al., Reference Plummer, McPhail and Monk1941). In its native habitat, A. ludens is long lived and undergoes only two generations in a year (Thomas, Reference Thomas2003). In central Mexico, the ancestral host of A. ludens is thought to be white sapote, C. edulis Llave et Lex (Birke et al., Reference Birke, Guillén, Midgarden, Aluja and Peña2013). This plant occupies a much more stable environment than C. greggii in terms of precipitation and humidity, growing interspersed with several other native and introduced host plants. Anastrepha ludens has adopted citrus and mango as its preferred exotic agricultural hosts over its entire geographical range (Birke et al., Reference Birke, Guillén, Midgarden, Aluja and Peña2013). In sharp contrast to yellow chapote, commercial production of citrus follows very predictable fruiting patterns with a steady and large supply of fruit for egg laying over most of the year. Additionally, the mean weight of commercial citrus fruit, mainly orange and grapefruit, is considerably larger than that of yellow chapote [25 g in C. greggii vs 130 and 430 g in orange (cv. Valencia) and grapefruit (cv. Ruby Red or Marsh), respectively]. In agricultural settings of northeastern Mexico, A. ludens periodically migrates from C. greggii to citrus (Thomas & Loera-Gallardo, Reference Thomas and Loera-Gallardo1998). Females from such populations could therefore encounter large variability in fruit size for egg laying and little variability in host availability. Isolated A. ludens populations, arguably never having been in touch with agricultural settings, only remain in secluded, hard-to-access canyons in the northern Sierra Madre Oriental [states of Nuevo León and Tamaulipas (fig. 1a, b)]. Females from such populations exploit fruit of relatively small uniform size, yet experience great variability in spatial and temporal fruit availability.

Fig. 1. Images showing areas from which experimental populations were collected: satelite image (a) and digital image (b) of the isolated and undisturbed area ‘El Jarro’; satelite image (c) of the agricultural area ‘Troncones’ and digital image (d) of a citrus grove next to native vegetation including Casimiroa greggii bushes in ‘Troncones’, (e) Anastrepha ludens females ovipositing into yellow chapote (small, oblong fruit pictured on the upper right hand) and grapefruit and (f) size differences among the ancestral host C. greggii and the cutivated hosts Citrus sinensis (orange) and Citrus paradisi (grapefruit).
One approach to studying phenotypic plasticity is to compare ancestral and derived populations that occupy different environments (Carroll et al., Reference Carroll, Dingle and Klassen1997, Reference Carroll, Klassen and Dingle1998; Parsons & Robinson, Reference Parsons and Robinson2006). A particularly plastic trait among animal species is behaviour, which is generally more plastic than morphological or developmental features (Relyea, Reference Relyea2001). Clutch size regulation is a behavioural response that has been found to be phenotypically plastic among several species of birds (Eggers et al., Reference Eggers, Griesser, Nystrand and Ekman2006), reptiles (Jordan & Snell, Reference Jordan and Snell2002), arthropods (Fox & Czesak, Reference Fox and Czesak2000) and tephritid fruit flies (Díaz-Fleischer & Aluja, Reference Díaz-Fleischer and Aluja2003a; Xu et al., Reference Xu, Zhou, Xiao, Zhang, Tang and Xu2012). In the case of A. ludens, egg-laying behaviour varies according to host species, nutritional content, ripeness, size and host predictability (Aluja et al., Reference Aluja, Piñero, Jácome, Díaz-Fleischer, Sivinski, Aluja and Norrbom2000, Reference Aluja, Díaz-Fleischer, Papaj, Lagunes and Sivinski2001, Reference Aluja, Birke, Guillén, Díaz-Fleischer and Nestel2011a; Díaz-Fleischer & Aluja, Reference Díaz-Fleischer and Aluja2003a, Reference Díaz-Fleischer and Alujab). Anastrepha ludens females react to different host properties by adjusting the size (ranging from 5 to >50 eggs) and number of egg clutches they lay in each host fruit. Plasticity in egg-laying behaviour can be evaluated in A. ludens by examining clutch sizes when females are exposed to different qualities and/or quantities of host fruit.
Here, to gain insights into the phenotypic plasticity of clutch size and oviposition behaviour in A. ludens, a native species of quaratine importance, hosts of different sizes were offered to cohorts of A. ludens populations originating from environments that differed sharply in the quality (i.e., size, nutritional content), and spatiotemporal availability of oviposition resources. Our working hypothesis, assuming a microevolutionary response to selection, was that highly isolated heterogeneous environments (in terms of seasonal fruit availability, microclimate), where flies only oviposit in a small native host, should select for individuals laying small clutches in variable numbers according to available fruit, whereas environments that offer fruit of variable size, some ten times larger than the wild host, and where fruiting periods are more uniform/stable, should select for individuals better able to adjust clutch size according to host size (i.e., insects that are able able to lay large clutches when the opportunity arises). In contrast, under a scenario fostering phenotypic plasticity, we postulated that wild populations from heterogeneous environments should be better able to regulate clutch size and number than populations under relaxed selection during artificial rearing where plasticity in clutch size regulation could be lost, resulting in females laying clutches of a more uniform size regardless of host-size variability.
Materials and methods
Biological material
Infested fruit were collected from branches and under the canopy of several C. greggii (yellow sapote) trees along the roads in the localities of Troncones, Ejido Libertad, Municipality of Ciudad Victoria, Tamaulipas, Mexico (12°47′4.72″N, 99°11′42.98″W) and in wild patches in El Jarro, Ejido Cuevas, Municipality of Iturbide, Nuevo Léon, Mexico (24°32′55.47″N, 99°45′30.7″W). Laboratory flies were reared by exposing one generation of flies stemming from a domesticated strain (125 generations) of A. ludens to grapefruit cv. Ruby Red. The colony flies had habitually been reared using artificial oviposition devices consisting of 12 cm Ø Petri-type plastic dishes covered with green linen cloth and filled with transparent silicon (Devcon, Junta Flex, ITW Poly Mex, Mexico). The plastic dish was placed upside down on top of the fly-holding cage so that females could oviposit through the cloth and lay eggs in the silicon. That is, before they were allowed to lay eggs into grapefruit, they had been reared for hundreds of generations (>20 years) using a large, stable artificial host of uniform size, in which the usual clutch size was >20 eggs per clutch. These flies were named the laboratory strain.
‘El Jarro’ is a highly isolated patch of native vegetation within a canyon in the Sierra Madre Oriental. The lower reaches of the canyon comprised mesophilic vegetation running along a small river that drained rainfall from the slopes of the Sierra, and was neighboured by a semi-arid zone in which scrubland vegetation prevailed (fig. 1a, b). The population from ‘El Jarro’ was named the wild strain.
‘Troncones’ is located next to an agriculturally developed area with numerous citrus orchards in the northern Mexican state of Nuevo Léon (fig. 1c–f). These flies were named the orchard.
Infested fruit collected in the highly isolated canyon and the agricultural setting (orchard) was taken to the laboratory in Xalapa, Veracruz and processed according to the methods described in Jácome et al. (Reference Jácome, Aluja and Liedo1999) to recover pupae. We acknowledge the fact that only one population was sampled of each contrasted origin. However, the fact that populations were not orthodoxically replicated, in favour of large representative samples from each area, is an issue recently addressed by Davies & Gray (Reference Davies and Gray2015) that guided our sampling approach.
Pupae and adults were handled and reared as adults following Aluja et al. (Reference Aluja, Díaz-Fleischer, Papaj, Lagunes and Sivinski2001). Pupae were individually weighed using an analytical balance (Sartorius CP64), sorted by weight (15–30 mg), placed in 200 ml plastic containers lined with a 2 cm layer of moist vermiculite and kept in 30 × 30 × 30 cm Plexiglass cages with free access to water and food at 26°C and 60% relative humidity until adult eclosion. Flies from the laboratory colony reared on grapefruit for one generation were treated identically to the wild flies.
Oviposition behaviour
A single male and female pair were picked at random from each adult cage representing the various origins: wild, ‘El Jarro’, agricultural ‘Troncones’ and the Xalapa laboratory strain. Each pair was placed in a 20 × 20 × 20 cm Plexiglass cage with free access to water and food. At 15 days of age (sexual maturity), an artificial green coloured, parafilm-wrapped, agar sphere was hung from the cage ceiling to serve as an oviposition device (Jácome et al., Reference Jácome, Aluja and Liedo1999). There were five different agar sphere sizes, but only one was used per cage (i.e., no choice tests were performed). Couples from each of the three strains were exposed to 1.8, 2.8, 3.8, 5 or 8 cm diameter spheres (fig. 2). Spheres were left in cages for 24 h, removed for dissection under a magnifying glass, and replaced daily with a new sphere of the same diameter. The procedure was repeated for a total of 20 days. A total of 30 couples for the agricultural and laboratory and 13 couples from wild strain were assayed for each sphere size. Sample size was reduced in the wild fly strain due to high levels of larval mortality by hymenopterous parasitoids. During dissection, the number of clutches laid in spheres and the number of eggs per clutch were counted and recorded, immediately following exposure to flies on each day of the experiment.

Fig. 2. Image showing the artificial oviposition devices used as a comparative range of naturally ocurring hosts of different sizes ranging from 1.8 to 8 Ø (cm).
Statistical analyses
A generalized linear mixed-effects model (GLMM) with Poisson distribution was run to model the lack of temporal independence and autocorrelation (Baayen et al., Reference Baayen, Davidson and Bates2008). Response variables were (a) total number of clutches and (b) total number of eggs laid per sphere and per day. The fixed factors were (1) the population of origin with three levels (wild, agricultural and laboratory-reared flies), and (2) the host size with five levels (1.8, 2.8, 3.8, 5 and 8 cm Ø), while female identity was considered as a random factor. As total eggs and clutches were determined for each female over a 20 days period, they were likely to show temporal autocorrelation (i.e., total eggs/clutches laid one day may influence the number of eggs/clutches laid the following days). We fitted two models and determined the significance of the predictors using a likelihood ratio test comparing the fit of the null model with the fit of both models (with and without interactions), including the random-effect and autocorrelation term, and selected the model without interaction as the interaction term did not result in a significant change in model deviance. To test whether mean clutch size differed among the three populations and was regulated in response to host size, data were normalized by log transformation prior to fitting a linear mixed-effects model (LMM). Female identity was included as a random effect. Q–Q plots and scatterplots of residuals were plotted against predicted values to assess normality and homoscedasticity of the model.
Clutch size laid by females of each three populations was subjected to linear regression against sphere size to establish the significance, strength and direction of the relationship. Analyses were performed using R (3.0.3, R Core Team, 2014); GLMMs and LMM were calculated using the function of the R package lme4 (Bates et al., Reference Bates, Maechler and Bolker2011).
Results
Oviposition behaviour
The total number of clutches laid per sphere differed significantly between the laboratory and the wild population (GLMM, z = 3.6, P < 0.001), but not between the agricultural and wild populations (GLMM, z = −1.04, P = 0.29) (table 1). Sphere size was also significant (GLMM, z = −16.11, P < 0.001). Anastrepha ludens females laid significantly more clutches in small spheres than in larger ones (fig. 3a), with females from the laboratory population laying similar numbers of clutches in 8 and 5 cm spheres, while females from both wild and agricultural populations laid fewer clutches in the largest spheres (fig. 3b). Host size significantly influenced clutch size all over the 20 days period among the three populations (LMM, t = 6.89, df = 4244, P < 0.001). The total number of eggs laid differed significantly for the laboratory populations (LMM, t = 10.058, df = 68, P < 0.001) (table 2).
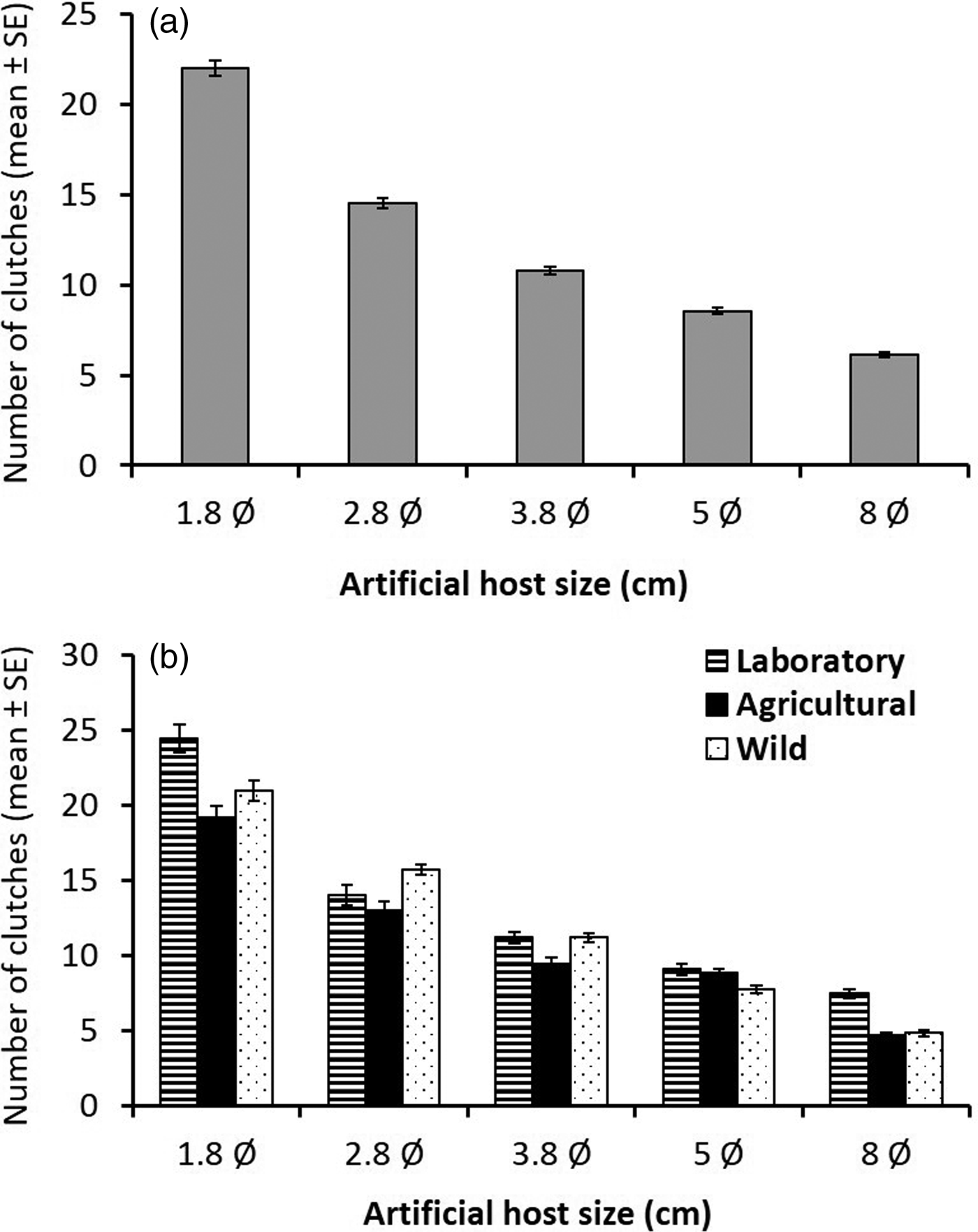
Fig. 3. Mean number of egg clutches (±SE) laid by: (a) individual sexually mature (15 days old) Anastrepha ludens females every 24 h over a 20 days period on artificial hosts of different diamaters (in cm) independent of fly origin (i.e., averaging over all origins) and (b) separating by fly origin [laboratory population (striped bars), an agricultural population (black bars) and wild population from an isolated habitat (dotted bars)].
Table 1. Analysis of the linear mixed-effects models using log-transformed data that examines the effect of population origin and host size on the mean clutch size over a 20 days period by Anastrepa ludens.

LMM, linear mixed-effects model.
Table 2. Results of GLMMs examining the effect of population origin and host size on the total number of clutches and eggs laid over a 20 days period by Anastrepha ludens females.

Overall, females from the laboratory population laid significantly larger clutches (6.23 mean ± 3.44 SD) than females from both the wild population, and females from the agricultural population. Wild strain females laid significantly larger clutches (5.24 ± 3.96) than females from the agricultural population (4.94 ± 3.88). When scatterplots of all treatments were compared, it was apparent that females gradually increased clutch size according to sphere size across all populations (fig. 4a).

Fig. 4. Mean (±SE) clutch size (number of eggs per clutch) for (a) A nastrepha ludens females laying in artificial spheres of different sizes independent of fly origin (i.e., averaging over all origins) and (b) separating by fly origin [laboratory population (striped bars), an agricultural population (black bars), and wild population from an isolated habitat (dotted bars)].
Importantly, the laboratory flies were less prone to adjust their clutch size to changing conditions (sphere sizes) than flies from the wild population, which accurately adjusted clutch size in line with sphere size until they encountered the very large fruit size (fig. 4b). But, when females of the wild and agricultural population encountered the largest sphere (8 cm Ø), clutch size appeared to be highly variable.
With respect to total number of eggs laid per sphere, significant differences were detected between the laboratory and the wild populations (GLMM, z = 8.67, P < 0.001), but this variable did not differ significantly between the agricultural and the wild strain (GLMM, z = −1.49, P = 0.14). Overall, sphere size had no significant effect on the total number of eggs laid (GLMM, z = 1.3, P = 0.193) (table 1). In general, flies from the laboratory population laid more eggs than females from the wild population, which in turn laid more eggs than flies from the agricultural setting (fig. 5). Differences in the cumulative number of eggs laid in hosts of different sizes over a 20 days period were not as sharp as those recorded for clutch number and clutch size; 1.8 cm spheres received the fewest eggs, whereas 5 cm spheres received more eggs than other sphere sizes (fig. 6a). Differences in cumulative number of eggs across different sphere sizes were less pronounced for females from the laboratory population indicating an inability to respond to environmental variability as efficiently as wild flies (fig. 6b).
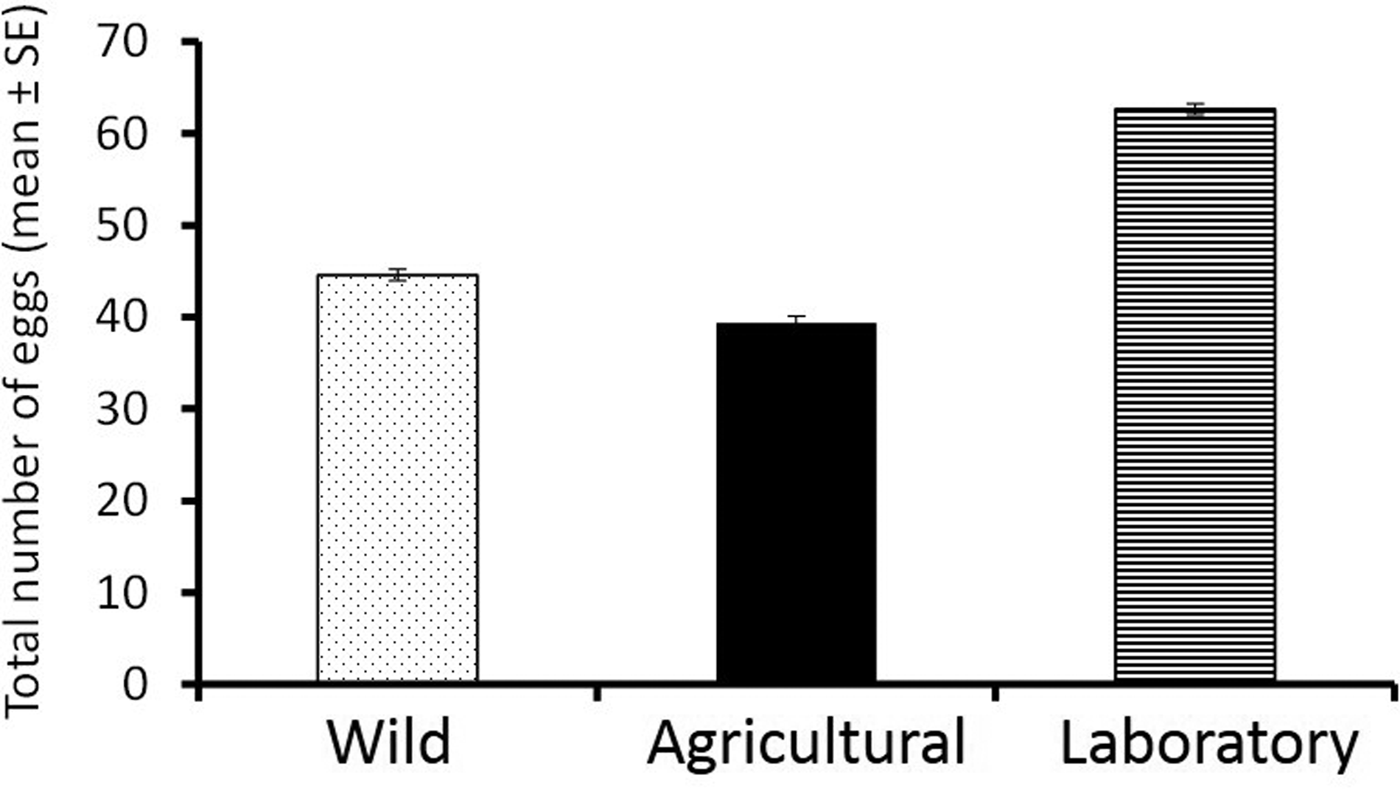
Fig. 5. Cumulative number (±SE) of eggs laid by A. ludens females from a laboratory population (striped bars), an agricultural population (black bars), and a wild population from an isolated habitat (dotted bars) independent of host size (i.e., averaging over all host sizes).
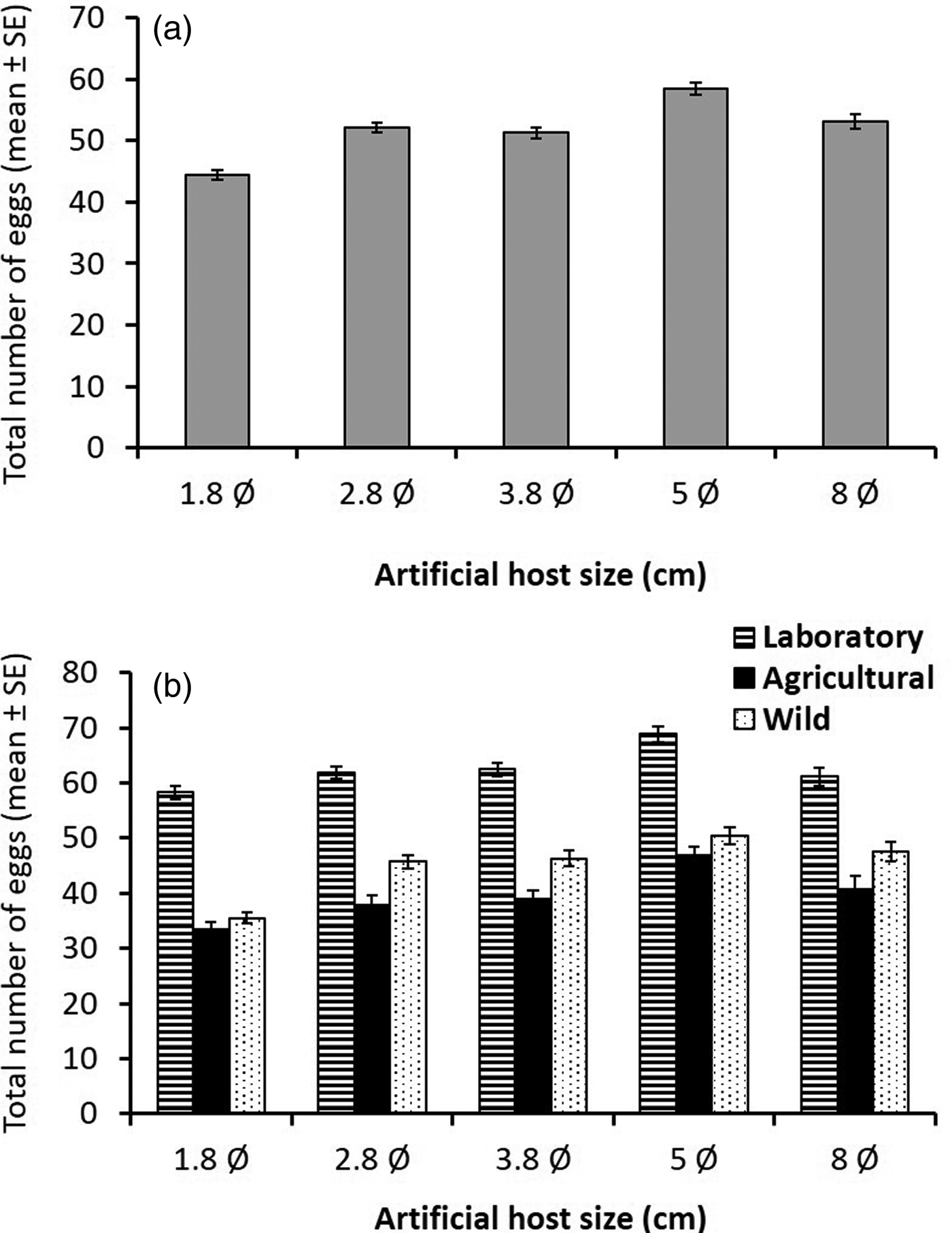
Fig. 6. Cumulative number of eggs laid over 20 days (±SE) for (a) A nastrepha ludens females laying in artificial spheres of different sizes independent of fly origin (i.e., averaging over all origins) and (b) separating by fly origin [laboratory population (striped bars), an agricultural population (black bars) and wild population from an isolated habitat (dotted bars)].
When regressing the mean clutch size against host size, both flies from the wild strain (clutch size = −1.056111 +− 1.4824963 × host size; R 2 = 0.55) and the agricultural strain (clutch size = −0.419167 + 1.2250874 × host size; R 2 = 0.44) exhibited a stronger positive linear relationship between clutch size and host size in comparison to that observed for the laboratory population (clutch size = 0.941464 + 1.1262361 × host size; R 2 = 0.37) (fig. 7). Overall, A. ludens females laid larger numbers of eggs in larger spheres and achieved this by laying fewer clutches of larger size.
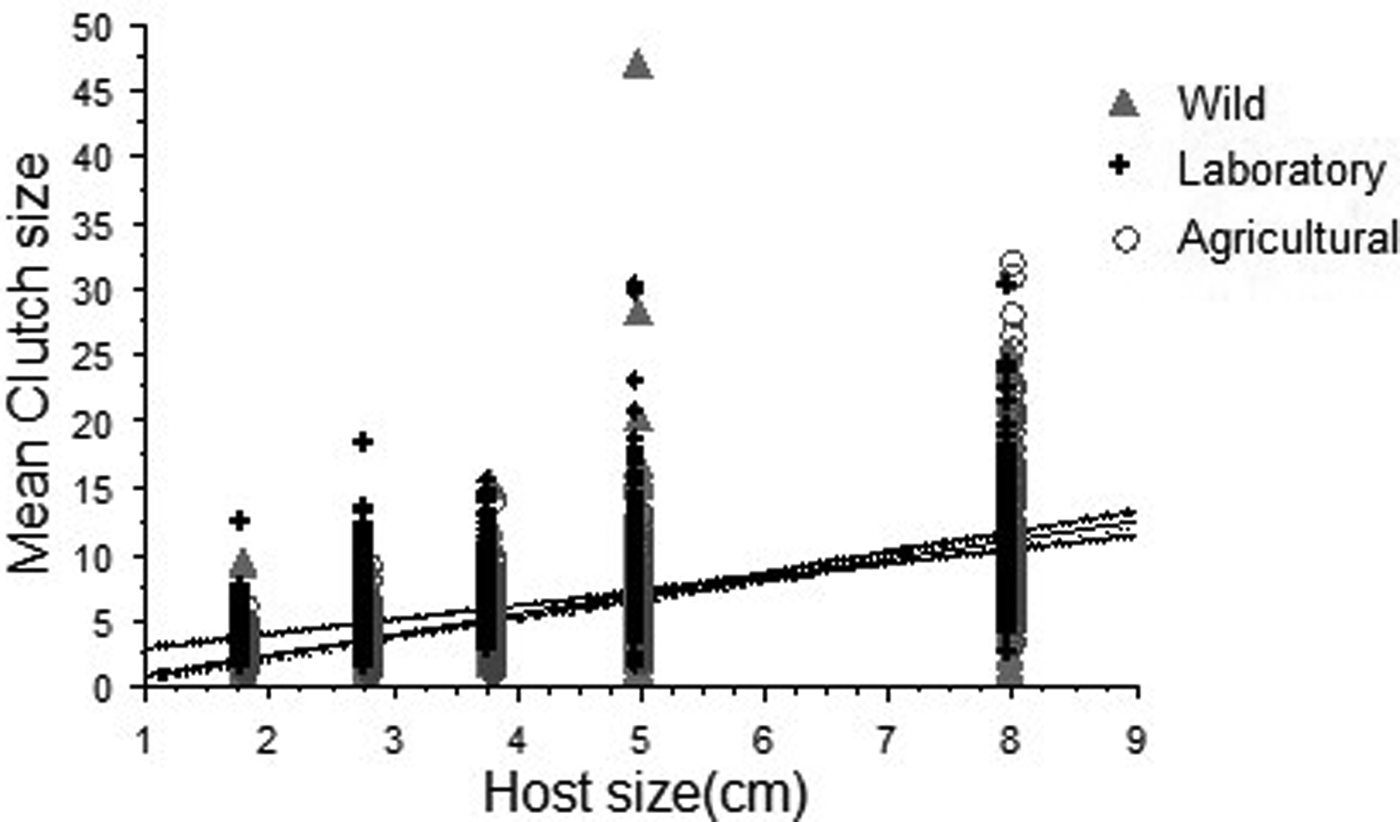
Fig. 7. Relationship between clutch size and host size for A nastrepha ludens females from laboratory, agricultural and wild environments. Significant regression equations were found for the three populations: wild (F 1,1633 = 2384.2, P < 0.001, clutch size = −1.837 + 1.564 × host size; R 2 = 0.59), agricultural (F 1,742 = 804.6, P < 0.001, clutch size = −0.824 + 1.489 × host size; R 2 = 0.52) and laboratory (F 1,1910 = 1248.3, P < 0.001, clutch size = 1.829 + 1.083 × host size; R 2 = 0.39).
Discussion
Individuals from a population under selection in a highly heterogeneous and isolated natural environment, and those experiencing seasonal variability in host size (an agricultural setting that offered flies both wild and cultivated hosts), exhibited significantly greater levels of behavioural plasticity than individuals extracted from populations stemming from stable and predictable environments (relaxed host-related selection). Nevertheless, and contrary to the notion that phenotypic plasticity shields genotypes from selection, populations occupying the ancestral heterogeneous range of distribution were more genetically diverse than those exploiting hosts with easy access to introduced stable agricultural hosts, and laboratory populations (Pecina-Quintero et al., Reference Pecina-Quintero, López-Arroyo, Loera Gallardo, Pons, Hernández, Mayek Pérez, Hernández Delgado, Rull and Aluja2009), suggesting either that plasticity itself can be selected for, or that, as is obvious, some genetic variability of populations exploiting ancestral hosts is lost during colonization. In sum, both spatial and temporal variation in host availability, produced individuals exhibiting greater plasticity in clutch size regulation than those stemming from populations where host-related selection was relaxed. As a result of the latter, our hypothesis postulating that large clutches were partly a result of contact with large commercial fruit was not supported. Rather, we found that individuals from a population inhabiting a highly isolated canyon, exploiting small ancestral hosts, exhibited a high degree of behavioural plasticity, enabling them to lay very small and very large clutches in response to variation in host size, independent of the fact that some of the hosts offered to them in the laboratory experiment were approximately ten times larger than the hosts they encounter in their natural habitat (fig. 1e, f). That is, environmental variability, expressed as variability in host availability, variable weather patterns guiding flowering and fruiting periods, and parasitism and predation pressures, apparently selected for behavioural plasticity, a valuable trait when flies are exposed to more stable, agricultural settings, as female flies can efficiently exploit large hosts laying large clutches of eggs into them.
Variability in host fruit size could select for plasticity in clutch size regulation. Tephritid species that lay eggs in clutches tend to lay larger clutches in large fruit (Díaz-Fleischer & Aluja, Reference Díaz-Fleischer and Aluja2003b). Individuals from populations exploiting hosts of variable size should be more successful if they are able to adjust clutch size according to host size thereby maximizing the probability of offspring survival and development while minimizing foraging time and associated predation risk (Díaz-Fleischer et al., Reference Díaz-Fleischer, Papaj, Prokopy, Norrbom, Aluja, Aluja and Norrbom2000). Under such a scenario, populations switching from the ancestral C. greggii to citrus in areas with large-scale commercial orchards should be better able to adjust clutch size than those exploiting only small-sized C. greggii in environments far removed from agricultural settings. Nevertheless, populations exploiting wild hosts part of the season, and commercial hosts the remainder of the time, were also very plastic in response to our experimental host size variability. Such a pattern might be explained by host fruit variability at another dimension. Large variation in crop size due to irregular precipitation patterns in arid environments could produce scenarios of scarce fruit, abundant competition and high natural enemy pressure (parasitoids) where females lay large clutches of eggs, of which only a few offspring survive to reproduce. In fact, A. ludens tends to lay larger clutches of eggs in unripe than in ripe fruit, which has been suggested as a bet-hedging mechanism to overcome greater mortality risks associated with offspring exposure to high levels of secondary compounds during development (Díaz-Fleischer & Aluja, Reference Díaz-Fleischer and Aluja2003a). Additionally, crop size could vary spatially from patch to patch depending on the availability of soil moisture, with plants near streams producing substantially more fruit than those in the upper reaches of the canyon. Under such conditions, females able to regulate clutch size could distribute their eggs more efficiently and produce more offspring.
It has been argued that phenotypic plasticity can shield genotypes from selection. Despite the latter, r-strategist polyphagous tephritid species such as Ceratitis capitata (Wiedemann) and Ceratitis rosa (Karsch) have been found to be more genetically heterogeneous than monophagous Bactrocera olea (Rossi) and Capparimyia savastanoi (Martelli) (Malacrida et al., Reference Malacrida, Guglielmino, D’ Adamo, Torti, Marinoni and Gasperi1996). Anastrepha ludens populations from different geographical origins and different host plant affiliations appear to possess little genetic variability (Pecina-Quintero et al., Reference Pecina-Quintero, López-Arroyo, Loera Gallardo, Pons, Hernández, Mayek Pérez, Hernández Delgado, Rull and Aluja2009; Frey et al., Reference Frey, Guillén, Frey, Samietz, Rull and Aluja2013). The apparent loss of diversity in populations exploiting introduced hosts in agricultural settings, as well as in the laboratory population we used in our oviposition experiment, could be explained by the fact that populations undergo genetic bottlenecks when colonizing novel hosts (Gilchrist et al., Reference Gilchrist, Cameron, Sved and Meats2012; Oroño et al., Reference Oroño, Paulin, Alberti, Hilal, Ovruski, Vilardi, Rull and Aluja2013). But, in the case of orchard (‘agricultural’) populations, given that A. ludens does not necessarily mate on its host plant (Aluja et al., Reference Aluja, Piñero, Jácome, Díaz-Fleischer, Sivinski, Aluja and Norrbom2000), populations exploiting different hosts may frequently hybridize after the citrus or alternate host growing season (Pecina-Quintero et al., Reference Pecina-Quintero, López-Arroyo, Loera Gallardo, Pons, Hernández, Mayek Pérez, Hernández Delgado, Rull and Aluja2009).
For invasive plants, it has been argued that the invasion success of many plant species might depend more heavily on their ability to respond to natural selection than on broad physiological tolerance or plasticity (Lee, Reference Lee2002). However, renewed scrutiny appears to indicate that successful invaders display greater phenotypic plasticity than native plants (Davidson et al., Reference Davidson, Jennions and Nicotra2011) and could be better able to maintain fitness in unfavourable environments or better able to increase fitness in favourable ones (Richards et al., Reference Richards, Bossdorf, Muth, Gurevitch and Pigliucci2006). For animals, tests of whether systematic differences in plasticity exist between indigenous and exotic species are relatively uncommon (Chown et al., Reference Chown, Slabber, McGeoch, Janion and Leinaas2007) and could shed some insight into invasion mechanisms. Among fruit flies, the most successful invaders are polyphagous, and may possess superior competitive abilities compared with displaced monophagous or stenophagous species (Duyck et al., Reference Duyck, David and Quilici2004). Nevertheless, in many cases, invasive tephritid species persist and thrive in exotic agricultural host plants and their success could also be due to a superior ability to occupy and exploit empty niches (Schliserman et al., Reference Schliserman, Aluja, Rull and Ovruski2014). Invasive tephritid species, such as Bactrocera dorsalis and C. capitata, exhibit an extraordinarily wide host range, yet limited population differentiation (Schutze et al., Reference Schutze, Krosch, Armstrong, Chapman, Englezou, Chomič and Clarke2012), and although it has been argued that this could be due to high levels of human-mediated gene flow (Karsten et al., Reference Karsten, van Vuuren, Barnaud and Terblanche2013), it could also be that invasive tephritid species are more plastic than many of the native species they encounter during an invasion.
Establishing unequivocally whether or not there is direct selection for plasticity on A. ludens populations in unpredictable environments would require determining whether plasticity is coded for by specific genes (Auld et al., Reference Auld, Agrawal and Relyea2010). Given the explosion and economic impact of biological invasions (Pimentel et al., Reference Pimentel, Zuniga and Morrison2005) and in particular recent geographic expansion of tephritid pests (Aluja et al., Reference Aluja, Guillén, Rull, Höhn, Frey, Graf and Samietz2011b, Reference Aluja, Birke, Ceymann, Guillén, Arrigoni, Baumgartner, Pascacio-Villafán and Samietz2014; Papadopoulos et al., Reference Papadopoulos, Plant and Carey2013), understanding how these organisms produce integrated, adaptive and environment-specific phenotypes may aid in predicting invasive abilities of particular species, a topic of increasing importance given the expected changes in the geographical distribution of these pests caused by global climate change (Aluja et al., Reference Aluja, Birke, Ceymann, Guillén, Arrigoni, Baumgartner, Pascacio-Villafán and Samietz2014).
Acknowledgements
The authors thank Trevor Williams for a critical review of an earlier version of this paper and thank Nicoletta Righini for statistical support and Rafael Villegas for providing digital images. They also appreciate comments on an earlier draft of this manuscript by an anonymous referee and the editor. The authors gratefully acknowledge the technical support of Zoraya Alvarado, Lizbeth González-Cobos, Darío García Medel and Flor Torres. This study was supported by the Mexican Consejo Nacional de Ciencia y Tecnología (CONACyT, Project 46846), the Mexican Campaña Nacional Contra Moscas de la Fruta (Convenio SAGARPA-IICA-INECOL) and the Instituto de Ecología, A.C. (INECOL).