Introduction
The availability of plant genome sequences is growing exponentially, which opens new dimensions for seed science research. One of the flagship themes of the 13th International Society for Seed Science (ISSS) conference (Kew Gardens, UK) was to connect seed traits and phylogenomics. This article summarizes the opening plenary lecture ‘Seed traits and phylogenomics’ given at the ISSS conference to highlight the utility of phylogenomics for future seed studies. Rather than discussing general aspects of phylogenomics, the focus of this article will be placed on the recent case study of the DELAY OF GERMINATION1 (DOG1) family genes, which illustrates interesting research developments during a phylogenomic study, including the generation of new hypotheses and theories for future functional genomics. While the case study highlights specific information about the gene family, it also raises interesting questions about the origins of seed programmes, such as reserve accumulation, dormancy and desiccation tolerance. The discussion will be extended through the possible roots of the molecular mechanisms of seed maturation programmes in non-seed plants. Potential applications of the knowledge obtained from evolutionary studies to future research in both fundamental and applied seed science will also be discussed.
Diversification of the DOG1 family genes in flowering plants
The DOG1 family genes (termed DFGs hereafter) of Arabidopsis include the six genes DOG1, DOG1-LIKE1 (DOGL1), DOGL2, DOGL3, DOGL4 and DOGL5 (Bentsink et al., Reference Bentsink, Jowett, Hanhart and Koornneef2006; Nishimura et al., Reference Nishimura, Tsuchiya, Moresco, Hayashi, Satoh, Kaiwa, Irisa, Kinoshita, Schroeder, Yates, Hirayama and Yamazaki2018). A genomic survey of DFGs in other angiosperm species has identified DOGL6, another DOGL found in multiple species (Nishiyama et al., Reference Nishiyama, Nonogaki, Yamazaki, Nonogaki and Ohshima2021). Extensive phylogenetic analysis of DFGs suggests that they have evolved in the four stem lineages such as DOG1, DOGL4, DOGL5 and DOGL6 in angiosperms, while DOGL1, DOGL2 and DOGL3 occurred within the DOG1 lineage (Nishiyama et al., Reference Nishiyama, Nonogaki, Yamazaki, Nonogaki and Ohshima2021) (Fig. 1). In terms of gene function, DOG1 has intensively been characterized for its involvement in seed dormancy (Bentsink et al., Reference Bentsink, Jowett, Hanhart and Koornneef2006; Cyrek et al., Reference Cyrek, Fedak, Ciesielski, Guo, Sliwa, Brzezniak, Krzyczmonik, Pietras, Kaczanowski, Liu and Swiezewski2016; Fedak et al., Reference Fedak, Palusinska, Krzyczmonik, Brzezniak, Yatusevich, Pietras, Kaczanowski and Swiezewski2016; Née et al., Reference Née, Kramer, Nakabayashi, Yuan, Xiang, Miatton, Finkemeier and Soppe2017; Nishimura et al., Reference Nishimura, Tsuchiya, Moresco, Hayashi, Satoh, Kaiwa, Irisa, Kinoshita, Schroeder, Yates, Hirayama and Yamazaki2018), while DOGL4 was found to play a role in seed reserve accumulation (Sall et al., Reference Sall, Dekkers, Nonogaki, Katsuragawa, Koyari, Hendrix, Willems, Bentsink and Nonogaki2019). The DOG1 involvement in seed dormancy has also been reported for Lepidium, another genus in Brassicaceae (Graeber et al., Reference Graeber, Voegele, Büttner-Mainik, Sperber, Mummenhoff and Leubner-Metzger2013, Reference Graeber, Linkies, Steinbrecher, Mummenhoff, Tarkowská, Turečková, Ignatz, Sperber, Voegele, de Jong, Urbanova, Strnad and Leubner-Metzger2014). The DOG1 function in dormancy or the DOGL4 function in reserve accumulation seems to be specific to each gene, although they also have an overlapping role in desiccation tolerance (Dekkers et al., Reference Dekkers, He, Hanson, Willems, Jamar, Cueff, Rajjou, Hilhorst and Bentsink2016; González-Morales et al., Reference González-Morales, Chávez-Montes, Hayano-Kanashiro, Alejo-Jacuinde, Rico-Cambron, de Folter and Herrera-Estrella2016; Sall et al., Reference Sall, Dekkers, Nonogaki, Katsuragawa, Koyari, Hendrix, Willems, Bentsink and Nonogaki2019), suggesting their common origin and functional diversification (Nonogaki et al., Reference Nonogaki, Nishiyama, Ohshima and Nonogaki2020).
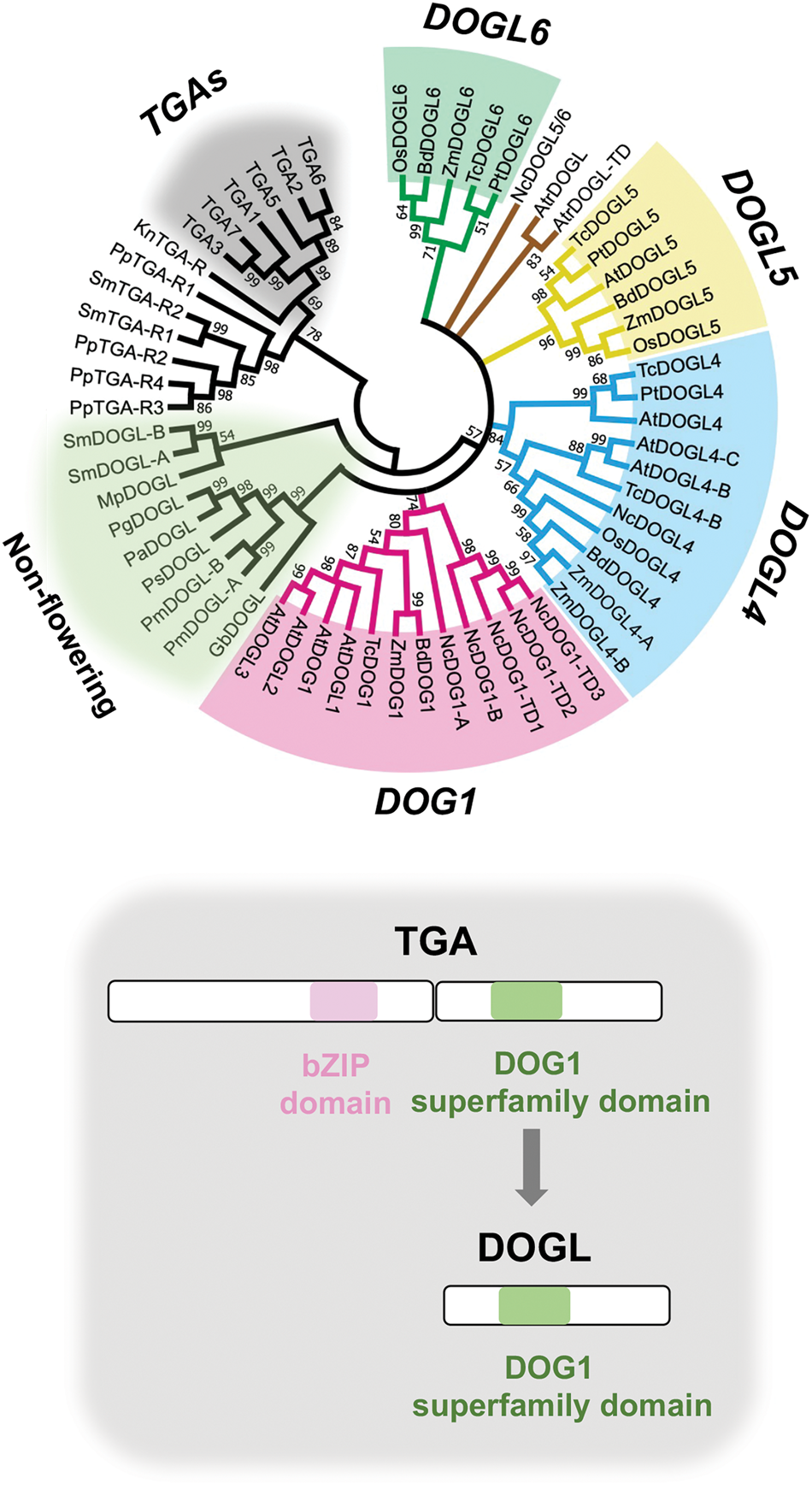
Fig. 1. Phylogeny of the DOG1 family genes. (Top) Phylogenetic tree of the DOG1 family genes (DFGs), including DOG1s and DOG1-LIKEs (DOGLs), which shows the diversification of DFGs in the four stem lineages (DOG1, DOGL4, DOGL5 and DOGL6) in angiosperms and the presence of DOGLs in non-flowering plants. Note that DOGL1, DOGL2 and DOGL3 are confined to the DOG1 lineage. The TGACG motif-binding transcription factor genes (TGAs) and TGA-related genes (TGA-Rs), which are associated with DOGLs, are used as an outgroup in the phylogeny (from Nishiyama et al. (Reference Nishiyama, Nonogaki, Yamazaki, Nonogaki and Ohshima2021)). (Bottom) Schematic representation of the possible evolution of DOGLs from TGAs. The basic leucine zipper (bZIP) domain and DOG1 superfamily domains are shown in the panel (based on Sall et al. (Reference Sall, Dekkers, Nonogaki, Katsuragawa, Koyari, Hendrix, Willems, Bentsink and Nonogaki2019)). At, Arabidopsis thaliana; Atr, Amborella trichopoda; Bd, Brachypodium distachyon; Gb, Ginkgo biloba; Mp, Marchantia polymorpha; Nc, Nymphaea colorata; Os, Oryza sativa; Pa, Picea abies; Pg, Picea glauca; Pm, Pseudotsuga menziesii; Pp, Physcomitrella patens; Ps, Pinus sylvestris; Pt, Populus trichocarpa; Sm, Selaginella moellendorffii; Tc, Theobroma cacao; Zm, Zea mays.
DFGs are found also in non-flowering plants where a single gene is a prevalent form (Nonogaki et al., Reference Nonogaki, Nishiyama, Ohshima and Nonogaki2020; Nishiyama et al., Reference Nishiyama, Nonogaki, Yamazaki, Nonogaki and Ohshima2021) (Fig. 1). Those results and the presence of multiple DOGLs in angiosperms suggest that DFG duplications occurred after the divergence of gymnosperms and angiosperms. DFG diversification probably occurred in early diverging angiosperms, which are also called basal angiosperms. In the genome of the basal angiosperm Nymphaea colorata (tropical water lily), the three genes DOG1, DOGL4 and DOGL5/6 (resembling DOGL5 and DOGL6) are detected. By contrast, a single form of DOGL is found in the genome of Amborella trichopoda that is supposedly the most ancient extant basal angiosperm, although another copy of this gene is found in its vicinity, probably due to tandem duplication (Nishiyama et al., Reference Nishiyama, Nonogaki, Yamazaki, Nonogaki and Ohshima2021). It is difficult to identify this A. trichopoda DOGL (AtrDOGL) as either of DOG1, DOGL4 or DOGL5/6 through sequence alignments. However, genome synteny analysis focusing on its neighbouring genes indicates that the same set of genes is conserved in the vicinity of N. colorata DOG1 (NcDOG1) and Arabidopsis thaliana DOG1 (AtDOG1) (Fig. 2), suggesting their evolutionary relationship. Interestingly, the Arabidopsis DOGL4 neighbourhood contains the genes similar to the DOG1 ‘signature’ genes (Nishiyama et al., Reference Nishiyama, Nonogaki, Yamazaki, Nonogaki and Ohshima2021), implying that DOG1 and DOGL4 originated from the same ancient locus possibly of a basal angiosperm species.

Fig. 2. The synteny of the DOG1 family genes suggesting their diversification in the early diverging angiosperms. It is difficult to identify AtrDOGL as either of DOG1, DOGL4, DOGL5 or DOGL6. However, the examination of its neighbouring genes, such as MEE62, CIPK19, CIPK20 and MDIS1, suggests its evolutionary relationship with the NcDOG1 (water lily) and AtDOG1 (compare the top three panels). The Arabidopsis DOGL4 neighbours also include similar genes like CIPK12 and MDIS2 (not shown in the figure), suggesting that DOG1 and DOGL4 originated from the same ancient locus. To support this idea, DOG1 and DOGL4 are found next to each other in the genome of T. cacao (bottom panel), with the mixture of the DOG1 signature neighbouring genes (green) and the DOGL4 signature neighbouring genes (light green). This type of genomic locus probably represents the ancient genomic arrangement of DFGs (from Nishiyama et al. (Reference Nishiyama, Nonogaki, Yamazaki, Nonogaki and Ohshima2021)). CIK4, CLAVATA3 INSENSITIVE RECEPTOR KINASE 4; CIPK, CBL-INTERACTING PROTEIN KINASE; DOT4, DEFECTIVELY ORGANIZED TRIBUTARIES 4; LSH9, LIGHT-DEPENDENT SHORT HYPOCOTYLS 9; MBF, multiprotein-binding factor; MDIS, MALEDISCOVERER; MEE62, MATERNAL EFFECT EMBRYO ARREST 62; PP, protein phosphatase; Rho, Rho termination factor; SCARL, SCAR-LIKE; SHAGGY, SHAGGY-LIKE KINASE; TD, tandem duplication; TULP, Tubby-like F-box protein.
To support this notion, DOG1 and DOGL4 are found next to each other in the genome of Theobroma cacao, where a mixture of the DOG1 and DOGL4 signature genes is found in their vicinity (Fig. 2). Likewise, colocalization of DOGL4 and DOGL5 is found in the Nelumbo nucifera (lotus) genome (Nishiyama et al., Reference Nishiyama, Nonogaki, Yamazaki, Nonogaki and Ohshima2021). While no gene is annotated next to the NnDOGL4–NnDOGL5 region in the lotus genome, a pseudo DOG1 that is comprised of two parts of collapsed open reading frames of a copy of DOG1 has been identified next to NnDOGL4. In addition, a pseudo NnDOGL4 has been found next to NnDOG1, which is annotated on a separate chromosome (Nishiyama et al., Reference Nishiyama, Nonogaki, Yamazaki, Nonogaki and Ohshima2021). Taken together, it is likely that DOG1, DOGL4 and DOGL5/6 originated from tandem duplications at an ancient locus of the most recent common ancestor of angiosperms.
Possible origins of seed maturation programmes in non-seed plants
DFG diversification probably occurred in angiosperms, which must have contributed to functional diversification of the gene family as well. It is possible that DOGLs were already playing some biological roles in non-flowering plants. The Amborella-type of intron-less DOGLs are found in gymnosperms, lycophytes and bryophytes (Nishiyama et al., Reference Nishiyama, Nonogaki, Yamazaki, Nonogaki and Ohshima2021). These findings raise interesting questions about the origins of the molecular mechanisms associated with seed maturation programmes, such as seed dormancy. In the liverwort Marchantia polymorpha, there is gemma dormancy where the germination of rhizoid from gemmae is blocked by abscisic acid (ABA) (Eklund et al., Reference Eklund, Kanei, Flores-Sandoval, Ishizaki, Nishihama, Kohchi, Lagercrantz, Bhalerao, Sakata and Bowman2018), as is the case of seed dormancy. It would be interesting to examine in future research whether M. polymorpha DOGL (MpDOGL) functions as a repressor of protein phosphatase 2C (PP2C) to enhance ABA sensitivity of gemmae, just like Arabidopsis DOG1 in seeds (Née et al., Reference Née, Kramer, Nakabayashi, Yuan, Xiang, Miatton, Finkemeier and Soppe2017; Nishimura et al., Reference Nishimura, Tsuchiya, Moresco, Hayashi, Satoh, Kaiwa, Irisa, Kinoshita, Schroeder, Yates, Hirayama and Yamazaki2018) (see below for more discussion about PP2C repression).
Bryophytes are not the oldest group containing DOGLs. They are found even in the algae Zygnematophyceae (Fig. 3), which is an algal sister lineage of land plants (Wodniok et al., Reference Wodniok, Brinkmann, Glöckner, Heidel, Philippe, Melkonian and Becker2011; Wickett et al., Reference Wickett, Mirarab, Nguyen, Warnow, Carpenter, Matasci, Ayyampalayam, Barker, Burleigh, Gitzendanner, Ruhfel, Wafula, Der, Graham, Mathews, Melkonian, Soltis, Soltis, Miles, Rothfels, Pokorny, Shaw, DeGironimo, Stevenson, Surek, Villarreal, Roure, Philippe, dePamphilis, Chen, Deyholos, Baucom, Kutchan, Augustin, Wang, Zhang, Tian, Yan, ei Wu, Sun, Wong and Leebens-Mack2014; Gitzendanner et al., Reference Gitzendanner, Soltis, Wong, Ruhfel and Soltis2018; Cheng et al., Reference Cheng, Xian, Fu, Marin, Keller, Wu, Sun, Li, Xu, Zhang, Wittek, Reder, Günther, Gontcharov, Wang, Li, Liu, Wang, Yang, Xu, Delaux, Melkonian, Wong and Melkonian2019). This timing coincides with the emergence of the ancestral form of ABA receptor pre-PYR1-LIKE (pre-PYL) (Sun et al., Reference Sun, Harpazi, Wijerathna-Yapa, Merilo, de Vries, Michaeli, Gal, Cuming, Kollist and Mosquna2019). Pre-PYL has been found in Zygnema circumcarinatum, while DOGLs can be found in Mesotaenium endlicherianum (MeDOGLs) of the same Zygnematophyceae (Fig. 3) (ME000109S10872 and ME000088S10108 in PhycoCosm; https://phycocosm.jgi.doe.gov/phycocosm/home). In Arabidopsis seeds, PYLs repress PP2C upon ABA perception and enhance ABA sensitivity, which results in dormancy (Cutler et al., Reference Cutler, Rodriguez, Finkelstein and Abrams2010), while pre-PYL in Zygnema represses PP2C without requiring ABA (Sun et al., Reference Sun, Harpazi, Wijerathna-Yapa, Merilo, de Vries, Michaeli, Gal, Cuming, Kollist and Mosquna2019) (Fig. 4). This is intriguing because it is exactly what DOG1 is doing in seeds; DOG1 is a PP2C repressor independent of ABA (Nishimura et al., Reference Nishimura, Tsuchiya, Moresco, Hayashi, Satoh, Kaiwa, Irisa, Kinoshita, Schroeder, Yates, Hirayama and Yamazaki2018).

Fig. 3. Ancestral DOGL and ABA receptor found in algae. DOGLs can be found in as early as MeDOGL in Zygnematophyceae, which is an algal sister lineage of land plants. Interestingly, this timing coincides with the timing of the emergence of the ancestral ABA receptor pre-PYRABACTIN RESISTANCE 1-LIKE (pre-PYL) found in Z. circumcarinatum (ZcPYL) in the same class (Sun et al., Reference Sun, Harpazi, Wijerathna-Yapa, Merilo, de Vries, Michaeli, Gal, Cuming, Kollist and Mosquna2019). Phylogeny is based on Cheng et al. (Reference Cheng, Xian, Fu, Marin, Keller, Wu, Sun, Li, Xu, Zhang, Wittek, Reder, Günther, Gontcharov, Wang, Li, Liu, Wang, Yang, Xu, Delaux, Melkonian, Wong and Melkonian2019). Photos: Mesotaenium and Zygnema, T. Friedl CC BY-SA 4.0 (sagdb.uni-goettingen.de) and Feng et al. (Reference Feng, Holzinger, Permann, Anderson and Yin2021), CC BY, respectively.

Fig. 4. ABA-independent PP2C repression by the algal pre-PYL and seed DOG1. In Arabidopsis, the ABA receptor PYRABACTIN RESISTANCE 1-LIKE (PYL) represses protein phosphatase 2C (PP2C) upon ABA perception (right), which in turn activates SNF1-related protein kinase 2 (SnRK2) to phosphorylate (P) transcription factors downstream, such as basic leucine zipper protein (bZIP), leading to stress responses, including seed dormancy (Cutler et al., Reference Cutler, Rodriguez, Finkelstein and Abrams2010). The ancestral ABA receptor pre-PYL (middle), which represses PP2C without requiring ABA, has been found in Z. circumcarinatum (Sun et al., Reference Sun, Harpazi, Wijerathna-Yapa, Merilo, de Vries, Michaeli, Gal, Cuming, Kollist and Mosquna2019). This is interesting because it is exactly how DOG1 functions in seeds (left): DOG1 is a PP2C repressor independent of ABA (Nishimura et al., Reference Nishimura, Tsuchiya, Moresco, Hayashi, Satoh, Kaiwa, Irisa, Kinoshita, Schroeder, Yates, Hirayama and Yamazaki2018). Thus, pre-PYL and DOG1 play similar roles in terms of enhancing stress responses without binding ABA. *Note that alternate mechanisms downstream of the DOG1 function have been predicted (Nonogaki, Reference Nonogaki2020). Photo (Zygnema): Feng et al. (Reference Feng, Holzinger, Permann, Anderson and Yin2021), CC BY.
Although molecular function of non-flowering plant DOGLs is not known yet, possible concurrent emergence of the ancestral ABA receptor and DOGL in the algal sister lineage of land plants is interesting. It is the time when plants were ‘landing’ from an aqueous environment, consequently exposing themselves to diverse stresses, such as drought and UV irradiance (de Vries and Archibald, Reference de Vries and Archibald2018; de Vries et al., Reference de Vries, Curtis, Gould and Archibald2018; Cheng et al., Reference Cheng, Xian, Fu, Marin, Keller, Wu, Sun, Li, Xu, Zhang, Wittek, Reder, Günther, Gontcharov, Wang, Li, Liu, Wang, Yang, Xu, Delaux, Melkonian, Wong and Melkonian2019). This window of terrestrial adaptation is where we can start to find the algal pre-PYL and DOGL. Pre-PYL later became an ABA receptor, which is an ABA-dependent PP2C repressor (Sun et al., Reference Sun, Harpazi, Wijerathna-Yapa, Merilo, de Vries, Michaeli, Gal, Cuming, Kollist and Mosquna2019), while DOGL evolved to DOG1, an ABA-independent PP2C repressor (Nishiyama et al., Reference Nishiyama, Nonogaki, Yamazaki, Nonogaki and Ohshima2021).
The timings of pre-PYL and DOGL emergence are similar; however, their evolutionary origins seem to be distinct. It has been proposed that pre-PYL originated from soil bacteria through horizontal gene transfer (Cheng et al., Reference Cheng, Xian, Fu, Marin, Keller, Wu, Sun, Li, Xu, Zhang, Wittek, Reder, Günther, Gontcharov, Wang, Li, Liu, Wang, Yang, Xu, Delaux, Melkonian, Wong and Melkonian2019) (Fig. 5). By contrast, DOGLs presumably evolved from the TGACG motif-binding transcription factors (TGAs) (Nishiyama et al., Reference Nishiyama, Nonogaki, Yamazaki, Nonogaki and Ohshima2021), which can be found in Klebsormidium nitens (GAQ81419.1 and GAQ90893.1) and Mesostigma viride (Mv17328-RA, PhycoCosm), more ancestral algae than Zygnematophyceae (Fig. 5). Interestingly, Klebsormidium already has PP2C and SNF1-related kinase 2 (SnRK2) (Hori et al., Reference Hori, Maruyama, Fujisawa, Togashi, Yamamoto, Seo, Sato, Yamada, Mori, Tajima, Moriyama, Ikeuchi, Watanabe, Wada, Kobayashi, Saito, Masuda, Sasaki-Sekimoto, Mashiguchi, Awai, Shimojima, Masuda, Iwai, Nobusawa, Narise, Kondo, Saito, Sato, Murakawa, Ihara, Oshima-Yamada, Ohtaka, Satoh, Sonobe, Ishii, Ohtani, Kanamori-Sato, Honoki, Miyazaki, Mochizuki, Umetsu, Higashi, Shibata, Kamiya, Sato, Nakamura, Tabata, Ida, Kurokawa and Ohta2014; Shinozawa et al., Reference Shinozawa, Otake, Takezawa, Umezawa, Komatsu, Tanaka, Amagai, Ishikawa, Hara, Kamisugi, Cuming, Hori, Ohta, Takahashi, Shinozaki, Hayashi, Taji and Sakata2019), and Mesostigma has SnRK2 (de Vries et al., Reference de Vries, Curtis, Gould and Archibald2018). ABA might have been mediated by another type of receptor (?) in those ancestral algae. Alternatively, PP2C–SnRK2 antagonism could have been modulated by another ligand rather than ABA at ancient times. In any event, outstanding questions about DOGLs are: When did they become PP2C repressors? Is it only in flowering plants, or it was from much more ancient time of non-seed plants? Those are the questions to be addressed by future research through international collaboration in the global seed research community.

Fig. 5. Proposed distinct evolutionary origins of the two PP2C repressors DOG1 and pre-PYL. The ancestral ABA receptor pre-PYL and ancestral DOGL seem to have emerged at the similar timings. This is the time when plants were ‘landing’ from water, exposing themselves to diverse stresses (de Vries and Archibald, Reference de Vries and Archibald2018; Cheng et al., Reference Cheng, Xian, Fu, Marin, Keller, Wu, Sun, Li, Xu, Zhang, Wittek, Reder, Günther, Gontcharov, Wang, Li, Liu, Wang, Yang, Xu, Delaux, Melkonian, Wong and Melkonian2019). The window of terrestrial adaptation of plants is where we can start to find pre-PYL and DOGL. pre-PYL later became an ABA receptor (Sun et al., Reference Sun, Harpazi, Wijerathna-Yapa, Merilo, de Vries, Michaeli, Gal, Cuming, Kollist and Mosquna2019), which is an ABA-dependent PP2C repressor, while DOGL evolved to DOG1, an ABA-independent PP2C repressor. Pre-PYL and DOGL emerged at similar timings; however, their evolutionary origins seem to be distinct. It has been proposed that pre-PYL originated in soil bacteria and were brought into plants through horizontal gene transfer (Cheng et al., Reference Cheng, Xian, Fu, Marin, Keller, Wu, Sun, Li, Xu, Zhang, Wittek, Reder, Günther, Gontcharov, Wang, Li, Liu, Wang, Yang, Xu, Delaux, Melkonian, Wong and Melkonian2019). By contrast, our analysis suggests that DOGLs have evolved from TGAs (Sall et al., Reference Sall, Dekkers, Nonogaki, Katsuragawa, Koyari, Hendrix, Willems, Bentsink and Nonogaki2019; Nishiyama et al., Reference Nishiyama, Nonogaki, Yamazaki, Nonogaki and Ohshima2021; and this study), which we can find in Klebsormidium and Mesostigma, more ancestral algae than Zygnema. Klebsormidium has PP2C and SnRK2 already (Hori et al., Reference Hori, Maruyama, Fujisawa, Togashi, Yamamoto, Seo, Sato, Yamada, Mori, Tajima, Moriyama, Ikeuchi, Watanabe, Wada, Kobayashi, Saito, Masuda, Sasaki-Sekimoto, Mashiguchi, Awai, Shimojima, Masuda, Iwai, Nobusawa, Narise, Kondo, Saito, Sato, Murakawa, Ihara, Oshima-Yamada, Ohtaka, Satoh, Sonobe, Ishii, Ohtani, Kanamori-Sato, Honoki, Miyazaki, Mochizuki, Umetsu, Higashi, Shibata, Kamiya, Sato, Nakamura, Tabata, Ida, Kurokawa and Ohta2014; Shinozawa et al., Reference Shinozawa, Otake, Takezawa, Umezawa, Komatsu, Tanaka, Amagai, Ishikawa, Hara, Kamisugi, Cuming, Hori, Ohta, Takahashi, Shinozaki, Hayashi, Taji and Sakata2019), and Mesostigma has SnRK2 (de Vries et al., Reference de Vries, Curtis, Gould and Archibald2018). An outstanding question is: When did DOGL become a PP2C repressor? KCM: Klebsormidium, Chlorokybus and Mesostigma; PP2C, protein phosphatase 2C; pre-PYL, pre-PYRABACTIN RESISTANCE 1-LIKE; SnRK, SNF1-related protein kinase; TGA, TGACG motif-binding transcription factor; ZCC, Zygnematophyceae, Coleochaete and Chara. Photo (Zygnema): Feng et al. (Reference Feng, Holzinger, Permann, Anderson and Yin2021), CC BY.
Algal genome analysis, which sounds somewhat immaterial to seed biology, could further advance our understanding about the history of the molecular mechanisms associated with seed maturation. Genome analysis of Chara (see Figs 3 and 5) indicates that the transcripts related to seed storage proteins, oleosins and LATE EMBRYOGENESIS ABANDUNT (LEA) proteins accumulate in its zygotes, which also germinate like seeds (after meiosis) (Nishiyama et al., Reference Nishiyama, Sakayama, de Vries, Buschmann, Saint-Marcoux, Ullrich, Haas, Vanderstraeten, Becker, Lang, Vosolsobě, Rombauts, Wilhelmsson, Janitza, Kern, Heyl, Rümpler, Villalobos, Clay, Skokan, Toyoda, Suzuki, Kagoshima, Schijlen, Tajeshwar, Catarino, Hetherington, Saltykova, Bonnot, Breuninger, Symeonidi, Radhakrishnan, Nieuwerburgh, Deforce, Chang, Karol, Hedrich, Ulvskov, Glöckner, Delwiche, Petrášek, Van de Peer, Friml, Beilby, Dolan, Kohara, Sugano, Fujiyama, Delaux, Quint, Theißen, Hagemann, Harholt, Dunand, Zachgo, Langdale, Maumus, Van Der Straeten, Gould and Rensing2018). Those proteins are induced by DOGL4 in Arabidopsis seeds (Sall et al., Reference Sall, Dekkers, Nonogaki, Katsuragawa, Koyari, Hendrix, Willems, Bentsink and Nonogaki2019). DOGLs are not found in Chara; however, these results imply that the very origin of the molecular mechanisms driving seed maturation programmes, such as reserve accumulation and desiccation tolerance, dates back through the ancient time of algae.
Concerning dormancy, the roots of its molecular mechanism could go back even further through red algae, one of the oldest groups of algae. In the unicellular red alga Cyanidioschyzon merolae, stress-induced ABA causes growth arrest of the cell (Kobayashi et al., Reference Kobayashi, Ando, Hanaoka and Tanaka2016) (Fig. 6), which is similar to dormancy in a sense. In this alga, which contains a single mitochondrion, a single chloroplast and the nucleus, ABA is likely produced in the chloroplast, while haeme, another signalling molecule, is probably produced in the mitochondrion (Watanabe et al., Reference Watanabe, Hanaoka, Ohba, Ono, Ohnuma, Yoshikawa, Taketani and Tanaka2013; Kobayashi et al., Reference Kobayashi, Ando, Hanaoka and Tanaka2016). These molecules are thought to play important roles for interorganelle communication and possibly retrograde signalling to the nucleus in the alga. A substantial volume of recent literature started to highlight the importance of intracellular signalling, including interorganelle communication and anterograde and retrograde signalling, in the seed cells for the regulation of dormancy and germination (summarized in Nonogaki, Reference Nonogaki2019). Therefore, it is crucial to go back through algae (and earlier organisms) and understand how mitochondrion and chloroplast started to live together in a cell and how they have established the well-orchestrated interaction with the nucleus. Thus, understanding even algal cell biology will be an integral part of seed biology in the future.

Fig. 6. Possible roots of dormancy-like ABA-induced growth arrest in the unicellular red alga. The ancient red alga C. merolae contains the nucleus (n), one mitochondrion (m) and one chloroplast (c). In this species, stress-induced ABA causes growth arrest of the cell by scavenging haeme, another signalling molecule, with tryptophan-rich sensory protein O (TSPO) (Kobayashi et al., Reference Kobayashi, Ando, Hanaoka and Tanaka2016). ABA and haeme, which are probably produced by chloroplast and mitochondrion, respectively, are thought to be involved in interorganelle communication (Kobayashi et al., Reference Kobayashi, Ando, Hanaoka and Tanaka2016) and possibly in retrograde signalling also. Intracellular signalling in the seed cells is also emerging as the key element of the germination and dormancy mechanisms (Nonogaki, Reference Nonogaki2019).
Integration of the outcomes of phylogenomic studies with experimental seed biology
While genomic information from non-flowering plants is useful to deduce the evolution of the molecular mechanism of seed maturation programmes, phylogenetic information of the actual seed genes, such as DOG1, DOGL4 and DOGL5/6 found in basal angiosperms (Nishiyama et al., Reference Nishiyama, Nonogaki, Yamazaki, Nonogaki and Ohshima2021), could address important biological questions about seeds in a more direct manner. For example, the basal angiosperms’ DOG1 and DOGL4 that were identified by phylogenomic studies can be used in genetic studies, including their heterologous expression in Arabidopsis. It is possible that Nymphaea DOG1 rescues the non-dormancy phenotype of the Arabidopsis dog1 mutants. Alternatively, chemically induced expression of Nymphaea DOGL4 in Arabidopsis seeds might upregulate seed storage protein, oleosin and LEA genes.
Instead of expressing the ancient genes in Arabidopsis, equally or even more exciting is to introduce the modern seed genes to basal angiosperms. Functional genomics using Amborella may not be practical; however, it has been proposed that Nymphaea thermarum, a smallest water lily, could be used as a model system for basal angiosperms (Povilus et al., Reference Povilus, Losada and Friedman2015). Successful transformation of another Nymphaea species with Agrobacterium has also been reported (Yu et al., Reference Yu, Qiao, Qiu, Yu, Zhou, Shen, Yu, Jiang, Han, Liu, Zhang, Chen, Chen and Zhuo2018). Therefore, it is feasible to test how the modern seed genes of eudicots and monocots affect the biology of early diverging angiosperms. These ‘back-to-the-future’ experiments are anticipated to produce very interesting results, possibly reconstituting key evolutionary events that might have happened during the history of seed plants.
Beside the evolution of the molecular mechanisms of seed maturation programmes, important evolutionary questions associated with seed formation, such as the origin and evolution of the endosperm, can also be investigated by the back-to-the-future experiments. For example, Amborella seeds have a well-developed triploid endosperm (Fogliani et al., Reference Fogliani, Gâteblé, Villegente, Fabre, Klein, Anger, Baskin and Scutt2017), while seeds of some Nymphaea species develop only a minute diploid endosperm, with a perisperm being the major storage tissue (Povilus et al., Reference Povilus, Losada and Friedman2015). The Arabidopsis genes that have been identified for their function in nucellus (i.e. perisperm) development or degeneration (e.g. TRANSPARENT TESTA16; Xu et al., Reference Xu, Fiume, Coen, Pechoux, Lepiniec and Magnani2016) can be expressed in Nymphaea species. Their expression could alter the fate (expansion or degeneration) of the nucellus, hence the ratio of perisperm and endosperm volume in mature Nymphaea seeds, addressing important questions about a possible evolutionary transition of the replacement of the perisperm by the endosperm. Outcomes of those approaches could provide a convincing experimental proof for evolution, which is normally very difficult to acquire. Thus, phylogenomic studies of seeds can efficiently be integrated with experimental biology and could contribute back to the field of evolutionary biology.
Prospects
Phylogenomics offers promising tools to advance our understanding of seeds in both mechanistic and evolutionary aspects. Its contribution might not be limited to basic seed biology but could enhance applied aspects of seed science, possibly leading to technology development. Evolutionary pressure has selected seed traits advantageous for their adaptation and survival under natural conditions. By contrast, agriculture often takes advantage of seed traits that are not necessarily useful for plant survival or that are even against evolutionary pressure. A good example is a non-shattering (grain retention) phenotype, which blocks seed dispersal. From this point of view, some of the ancient seed traits might be useful for agriculture.
In the basal angiosperm Hydatella inconspicua (Nymphaeales) seeds that contain a perisperm as the major storage tissue, the accumulation of starch in the perisperm is initiated even before fertilization, which is a gymnosperm tendency (Friedman, Reference Friedman2008). This ancient trait in early diverging angiosperms is probably wasteful in terms of resource allocation within a maternal plant and has been suppressed by evolutionary pressure, so that only successfully fertilized ovules are nourished as observed in the endosperm of many angiosperm species (Friedman, Reference Friedman2008). However, this fertilization-independent reserve accumulation might be useful for crop modification. If perisperm or endosperm development can be induced without fertilization in grain crops, especially when it is pre-programmed in a spontaneous manner (with a fertility restoration programme), more efficient and sustainable grain production can be established. Recurring issues of reduced yield of grain crops under heat and drought conditions, which are caused by unsuccessful fertilization due to those stresses, can be addressed by this approach bypassing the stress-sensitive stage.
Fertilization-independent embryo formation could also be possible if the origin, evolution and core mechanisms of apomixis are fully understood. There was an encouraging development in rice apomixis research recently (Khanday and Sundaresan, Reference Khanday and Sundaresan2021). It might be sooner than we think when seed cloning of F-1 hybrid vegetables will also become possible, which could drastically change the architecture of global seed business. A better understanding of nucellus development, including its interaction with the integuments, might allow us to freely modify testa properties as well. For instance, if we could induce water- and gas-impermeable integuments (i.e. testa) typical of hard seeds, it will change common practice of seed storage and supply, because hard seeds could maintain their longevity without requiring specific warehouses or shipping packages while their germination can be induced by relatively simple scarification just before imbibition. Thus, more understanding of the evolution of developmental programmes of seed parts (testa, perisperm, endosperm and embryo) will offer opportunities of crop modification and seed business also.
While evolutionary studies are focused on past events, they could provide forward-looking perspectives for research and innovative ideas for technology development. Plants and seeds have performed a myriad of experiments over the course of evolution, probably with numerous failures from a fitness point of view, some of which can be revitalized for applied purposes as discussed above. Many of the experimental records of plants have probably been written in their genomes, including the ancient mechanisms, which might still be present in the genome but are just silenced. Those lost traits can be retrieved from plant genomes. Therefore, we should maximize the utility of plant genomes for future seed research and technology development. The potential of seed science is not limited to agricultural and pharmaceutical applications. Recently, ‘seed-inspired microfliers’, which can be equipped with electronic device to monitor environmental conditions, have been developed (Kim et al., Reference Kim, Li, Kim, Park, Jang, Wang, Xie, Won, Yoon, Lee, Jang, Lee, Chung, Jung, Heo, Lee, Kim, Cai, Kim, Prasopsukh, Yu, Yu, Avila, Luan, Song, Zhu, Zhao, Chen, Han, Kim, Oh, Lee, Lee, Huang, Chamorro, Zhang and Rogers2021). Thus, seeds have unlimited potential and are the ‘natural capital’ (Mattana et al., Reference Mattana, Ulian and Pritchard2021) for the 21st century.
Acknowledgements
We are grateful to Hugh Pritchard, Louise Colville and the scientific committees of the 13th ISSS conference for inviting to give the opening plenary lecture, which this article is based on. We also thank the audience of the ISSS conference who raised interesting questions and discussions for the future of seed science.
Conflicts of interest
None declared.