Introduction
Alunite-supergroup minerals (ASM) have the general chemical formula DG 3(TX 4)2X’6 using the symbolism proposed by Smith et al. (Reference Smith, Roberts, Bayliss and Liebau1998); where the chemical elements and vacancies that may occur in the crystal-structure sites, listed in order of decreasing valency are: D = Th4+, (Ce, La, Nd, Bi)3+, (Ca, Sr, Ba, Pb, Hg)2+, (Na, K, Rb, Ag, Tl, NH4, H3O)+; □; G = Sn4+, (Al, Fe, V, Cr, Ga)3+, (Cu, Zn, Mg)2+; T = (S, Cr)6+, (P, As, Sb)5+, Si4+; X/X’ = O2–, OH–, F–, H2O (Bayliss et al., Reference Bayliss, Kolitsch, Nickel and Pring2010). There are extensive solid solutions at the D, G and T sites within the alunite supergroup, so the structure can incorporate a large number of elements and its chemical composition reflects those of the fluids from which it formed.
Alunite-supergroup minerals occur in diverse environments near the Earth's surface, in rocks of metamorphic, igneous and sedimentary origin (Dutrizac and Jambor, Reference Dutrizac, Jambor, Alpers, Jambor and Nordstrom2000; Dill, Reference Dill2001; Gaboreau et al., Reference Gaboreau, Beaufort, Viellard and Patrier2005) and there is evidence of jarosite, and recently also alunite, in Martian regolith (Klingelhöfer et al., Reference Klingelhofer, Morris, Bernhardt, Schroder, Rodionov, de Souza, Yen, Gellert, Evlanov, Zubkov, Foh, Bonnes, Kankeleit, Gutlich, Ming, Renz, Wdowiak, Squyres and Arvidson2004; Madden et al., Reference Madden, Bodnar and Rimstidt2004; Ehlmann et al., Reference Ehlmann, Swayze, Milliken, Mustard, Clark, Murchie, Breit, Brigitte Gondet, Poulet, Carter, Calvin, Benzel and Seelos2016). Supergene processes in sulfide ore deposits, pyritiferous rocks, acid soils and hypogene alteration are also responsible for the precipitation of ASM (Dutrizac and Jambor, Reference Dutrizac, Jambor, Alpers, Jambor and Nordstrom2000; Dill, Reference Dill2001; Bayliss et al., Reference Bayliss, Kolitsch, Nickel and Pring2010). The Pb-rich members of the beudantite group, corkite and hinsdalite, are rare secondary minerals, which typically form in the supergene alteration of base-metal deposits (e.g. Scott, Reference Scott1987; Stanley, Reference Stanley1987; De Bruiyn et al., Reference De Bruiyn, Van der Westhuizen, Beukes and Meyer1990; Rattray et al., Reference Rattray, Taylor and Bevan1996; Frost and Palmer, Reference Frost and Palmer2011; Frost et al., Reference Frost, Palmer and Xi.2011). Plumbojarosite and Pb-rich alunite are most commonly found in the gossans which overlie massive sulfide deposits (Scott, Reference Scott1987).
Rhabdophane-group minerals (RGM) are hexagonal phosphates with the general formula ABO4·nH2O; where A = (Th, U)4+, REE 3+, (Ca, Pb)2+; B = P5+ and minor S6+, As5+, Si4+; and n = 0.5–2 (Hukuo and Hikichi, Reference Hukuo and Hikichi1979; Jones et al., Reference Jones, Wall and Williams1996; Strunz and Nickel, Reference Strunz and Nickel2001). They have a similar mineral chemistry to monoclinic monazite-type structure minerals but yield lower totals from electron probe microanalysis because of the presence of molecular water in zeolitic channels (Mooney, Reference Mooney1950; Bowles and Morgan, Reference Bowles and Morgan1984; Kijkowska et al., Reference Kijkowska, Cholewka and Duszak2003). Rhabdophane loses water, even if heated to low temperatures (160°C for 1 hour, Bowles and Morgan, Reference Bowles and Morgan1984) and transforms to monazite at temperatures ≥ 500°C (e.g. Johansson and Vance Reference Johansson and Vance1986; Akers et al., Reference Akers, Grove, Harrison and Ryerson1993).
Models of RGM formation involve the low-temperature weathering of Th- and REE-bearing minerals (Sawka et al., Reference Sawka, Banfield and Chappell1986; Banfield and Eggleton, Reference Banfield and Eggleton1989; Shoji and Akai, Reference Shoji and Akai1994; Nagy et al., Reference Nagy, Draganits, Demény, Pantó and Arkai2002; Krenn and Finger, Reference Krenn and Finger2007; Berger et al., Reference Berger, Gnos, Janots, Fernandez and Giese2008). These processes occur in soils which develop on syenites and gneiss (Braun et al., Reference Braun, Pagel, Muller, Bilong, Michard and Guillet1990) and also in palaeosols on Archean granite (Murakami et al., Reference Murakami, Utsunomiya, Imazu and Prasad2001). Grayite-like minerals formed by the late-stage hydrothermal alteration of a Th-rich mineral, probably thorite, in Wisconsin granitic pegmatites, USA (Buchholz et al., Reference Buchholz, Falster and Simmons2010) and in small cavities in beryl from Sredna Gora microcline-albitic pegmatites, Bulgaria (Zidarov and Petrov, Reference Zidarov and Petrov2011). The REE phosphates of the rhabdophane group, including brockite, are documented from oxidized carbonatites (Andersen et al., Reference Andersen, Clark, Larson and Neill2016). Moreover, rhabdophane-(Y) has been recorded in a druse in alkaline Higashimatsuura basalt in Japan (Takai and Uehara, Reference Takai and Uehara2012); and brockitic rhabdophane-(Ce) and rhabdophane-(La) occur in alkaline basalt (‘teschenite’) of the Silesian Unit, Czech Republic (Matýsek, Reference Matýsek2013). Several occurrences of rhabdophane-group minerals and the closely related phase ningyoite have been reported in sedimentary uranium deposits of the Bohemian Cretaceous Basin, Czech Republic (Scharmová and Scharm, Reference Scharmová and Scharm1994).
Rhabdophane is stable in low-temperature environments (Akers et al., Reference Akers, Grove, Harrison and Ryerson1993) and oxidizing conditions (Nagy and Draganits, Reference Nagy and Draganits1999). Experiments suggest low-temperature (<100°C) precipitation from supersaturated acidic aqueous solutions (Herrero et al., Reference Herrero, Blanco, Oelkers and Benning2011). Analyses usually indicate distinctive fractionation and remobilization of REE during exogenic processes (e.g. Bowles and Morgan, Reference Bowles and Morgan1984; Banfield and Eggleton, Reference Banfield and Eggleton1989; Scharmová and Scharm, Reference Scharmová and Scharm1994).
However, RGM and especially their chemistry and substitutions are poorly understood, and the published formulae of some RGM members are incorrect (e.g. grayite ideally ThPO4·H2O is not charge-balanced, Anthony et al., Reference Anthony, Bideaux, Bladh and Nichols2000) or represent empirical compositions. Therefore, the objective of our study is a contribution to the complex crystal chemistry and principal substitution mechanisms of ASM and RGM. Consequently, we describe here a complex assemblage of ASM, jarosite, Pb-rich jarosite and alunite, including very rare members (corkite and hinsdalite) with Ce-, La- and Nd-dominant RGM, which were identified in altered microgranite from the Velence Hills, Hungary. Some unique microtextures, displayed by RGM crystals, as well as specific chemical compositions of ASM and RGM are documented for the first time. Moreover, we suggest end-member formulae of divalent and tetravalent species of RGM: brockite, grayite and tristramite.
Regional geology
The Velence Hills cover a relatively small region on the Great Hungarian Plain near the town of Székesfehérvár in western Hungary (Fig. 1). The Velence Hills represents a horst structure belonging to the Transdanubian (Pelso) Unit. The oldest rocks include Ordovician to Devonian anchi- and epi-metamorphic metapelite to metapsammite with tuffaceous layers, rarely lydite, and limestone of the Lovas Slate Formation, also meta-andesite and dolerite occur in some places (Horváth et al., Reference Horváth, Daridáné Tichy, Dudko, Gyalog and Ódor2004).

Fig. 1. Simplified geological map of the Velence Hills area modified after Horváth et al. (Reference Horváth, Daridáné Tichy, Dudko, Gyalog and Ódor2004) and Benkó et al. (Reference Benkó, Molnár, Billström, Pécskay and Lespinasse2010, Reference Benkó, Molnár, Lespinasse, Billström, Pécskay and Németh2014).
Granitic rocks (Velence Granite Formation) intrude metamorphic rocks of the Lovas Slate Formation. The contact effect of the Velence granite on the surrounding rocks has produced andalusite (+biotite + muscovite + quartz) hornfels in the proximal and spotted slates (quartz + chlorite + muscovite) in the distal thermal–metamorphic aureole. The character of the contact metamorphism indicates relatively shallow-level emplacement and solidification of the granite intrusion. The Velence Granite Formation can be divided into the following intrusive phases (Horváth et al., Reference Horváth, Daridáné Tichy, Dudko, Gyalog and Ódor2004). (1) The oldest, early intrusive phase which includes mafic xenoliths of dioritic to granodioritic composition in the granites of the main phase. (2) The main intrusive phase which is characterized by widespread medium-grained, equigranular biotitic granite, less common porphyritic granite, microgranite, aplite and pegmatite dykes. (3) A dyke intrusive phase consisting of granite porphyries, microgranite, aplite, tourmaline-quartz dykes and silicified intrusive breccia. (4) The youngest, late phase which includes independent intrusion of the Kisfaludi microgranites. Microgranular quartz monzodioritic enclaves, fayalite-bearing nests of granitic pegmatites and metapelitic xenoliths were also described from the Velence granitic pluton (Buda, Reference Buda1993).
The most common accessory minerals of the Velence Granite Formation consist of zircon, ‘apatite’ (presumably fluorapatite), allanite-(Ce), ilmenite and pyrite, whereas monazite-(Ce), xenotime-(Y), rutile, anatase, magnetite, ‘garnet’ (presumably almandine to spessartine), ‘tourmaline’ (probably schorl), epidote and molybdenite are relatively rare (Uher and Broska, Reference Uher and Broska1994). The composition of allanite-(Ce) is described by Buda and Nagy (Reference Buda and Nagy1995). The zircon typology (dominant P-subtypes) and relatively low Hf content of zircon crystals (the weight Zr/Hf ratio attains 51 to 64 in central parts), biotite composition (annite with a high Fe/Mg ratio) and bulk-rock geochemistry classify the Velence Granite Formation as a K-rich and Ca-poor post-orogenic or anorogenic granite suite of A-type affinity (Uher and Broska, Reference Uher and Broska1994, Reference Uher and Broska1996; Broska and Uher, Reference Broska and Uher2001). These data are corroborated by the Rb–Sr isotope dating of biotite in the Velence Granite Formation which gave 280 ± 7 Ma (Buda, Reference Buda1985), and the pluton is therefore part of the post-Variscan (Permian) magmatic activity in the West-Carpathian–Pannonian area.
The Mesozoic rocks of the Velence Hills include Triassic limestone, marl, sandstone and dolomite and Upper Cretaceous lamprophyric dykes. Paleogene (Eocene to Oligocene) calc-alkaline, subduction-related andesite to diorite intrusions with related hydrothermal mineralization and subsequent low-temperature metasomatic alteration overprinted older, mainly granitic rocks in the eastern part of the Velence Hills (e.g. Daridáné-Tichy, Reference Daridáné-Tichy1987; Horváth et al., Reference Horváth, Daridáné Tichy, Dudko, Gyalog and Ódor2004). The youngest geological formations consist of Neogene (Pliocene) to Quarternary sediments, including gravel, sand, clay, loess, fluvial, deluvial, lacustrine and marsh sediments (Horváth et al., Reference Horváth, Daridáné Tichy, Dudko, Gyalog and Ódor2004).
Analytical methods and formula calculations
Electron probe microanalysis (EPMA) in wavelength-dispersive spectrometry (WDS) mode was performed using CAMECA SX 100 equipment at the Department of Electron Microanalysis at the State Geological Institute of Dionýz Štúr, Bratislava, Slovak Republic. An accelerating voltage of 15 kV was used and other relevant analytical conditions were chosen according to mineral type. The typical spot beam diameter varied from 3 to 10 µm, a more focused <1–3 µm beam was used for secondary Pb-rich ASM, and occasionally for REE phases. The ASM were analysed with 20 nA and RGM with 40 nA probe currents. The EPMA was calibrated using natural and synthetic standards (Table 1), and raw counts were converted to wt.% of oxides using ‘PAP’ matrix correction factors (Pouchou and Pichoir, Reference Pouchou, Pichoir and Armstrong1985).
Table 1. EPMA conditions used for each element.

ASM = alunite-supergroup minerals; RGM = rhabdophane-group minerals; n.a. = not analysed.
For comparison and elemental mapping, a selection of ASM and RGM were analysed using a JEOL JXA–8530 F electron microprobe at the Earth Science Institute of the Slovak Academy of Sciences in Banská Bystrica, under the following conditions: accelerating voltage 15 kV, probe current 20 nA and beam diameter <1–5 µm with ZAF matrix correction. Measurements performed under differing analytical conditions using different elemental standards (Table 1) gave consistent results within the error limit.
The ASM (Pb-free jarosites) are susceptible to beam damage and the small size and metamict state of some of the RGM precludes accurate analyses. These difficulties caused small deviations from ideal stoichiometry (usually overestimating the D site (ASM) and A site (RGM) and underestimating the T site (ASM) and B site contents (RGM), producing higher or lower totals (±5 wt.%) in some analyses). Despite these difficulties, almost all of these data are usable in compositional studies although the fine scale of the compositional segregation of Pb-free and Pb-rich ASM members occasionally prevented reliable chemical analyses of individual minerals. In these cases, the beam sizes in the μm range or less were preferable.
The Raman spectra of the RGM, synthetic rhabdophane-(La) and ASM were determined on thin sections using a LabRAM-HR Evolution (Horiba Jobin-Yvon) spectrometer system with a Peltier-cooled CCD detector and Olympus BX-41 microscope (at Masaryk University, Brno and the Earth Science Institute, Banská Bystrica). Raman spectra were excited by 473 and 532 nm diode lasers at a power of ~20 mW at the sample surface and collected in the 100–4000 cm–1 range. The spectral resolution was ~2 cm–1 using a grid of 600 gr.mm–1, and wavenumber calibration was by Rayleigh line and Ne-emission lines. The acquisition time was 10 s per frame and band fitting assumed combined Lorentzian-Gaussian band shape.
Rhabdophane-(La) synthesis was based on the procedure described by Kijkowska (Reference Kijkowska2003): 0.48 g of La2O3 was treated with 18 g of phosphoric acid (11 M H3PO4) to obtain a solution of 0.02 M La2O3 in 2 M H3PO4, and the mixture was magnetically stirred for 2 h, then left overnight to completely dissolve the La2O3. The resultant clear solution was diluted to 100 ml with deionized water, then transferred to a Teflon-lined pressure vessel and slowly heated to 140°C at 20°C/h. The pressure vessel was cooled rapidly and fine crystals (≤10 µm) of rhabdophane-(La) were removed from the solution and rinsed in deionized water.
Element contents in the mineral formulae are expressed in atoms per formula unit (apfu), The ASM nomenclature used is after Bayliss et al. (Reference Bayliss, Kolitsch, Nickel and Pring2010) and the formulae normalized on the basis of Σ(T + G) = 5 cations and the total H2O content calculated assuming OH + F = 6 apfu. The RGM were normalized on the basis of 4 oxygen atoms, and Ca–Pb–Th–U end-members and substitution tie-lines calculated as follows: tristramite (Ca0.5U0.5)PO4·2H2O, brockite (Ca0.5Th0.5)PO4·H2O and grayite (Pb0.5Th0.5)PO4·H2O.
Results
Sample description
The samples described herein are from an altered porphyritic microgranite which crops out on the eastern slope of Bence Hill, near Velence village in the eastern part of the Velence Hills. It forms the margin of the main intrusive phase of the Velence Granite Formation (Horváth et al., Reference Horváth, Daridáné Tichy, Dudko, Gyalog and Ódor2004). The microgranite has a very fine-grained granoblastic (aplitic) texture of quartz, K-feldspar and albite plagioclase with porphyritic phenocrysts of perthitic K-feldspar in some places. Anhedral aggregates of muscovite occur along the feldspar boundaries. Secondary quartz forms veinlets which cut primary magmatic quartz and alkali feldspars, and the groundmass, especially plagioclase, is altered extensively to a microscopic aggregate of white mica, clay mineral(s), a secondary phosphate-sulfate assemblage (consisting of alunite-supergroup and rhabdophane-group minerals) and iron oxyhydroxides (mostly goethite).
Alunite-supergroup minerals
The ASM consist of end-member jarosite [KFe3(SO4)2(OH)6], with Pb-rich jarosite, Pb-rich alunite [KAl3(SO4)2(OH)6] and the Pb-rich members of the beudantite group: corkite [PbFe3(P0.5S0.5O4)2(OH)6] and hinsdalite [PbAl3(P0.5S0.5O4)2(OH)6]. The jarosite and other ASM occur as a fine-grained matrix around, or in close vicinity to vugs (Fig. 2a) or as fissure-fillings with massive iron oxyhydroxides (goethite and/or hematite) (Fig. 2b) and associated clay minerals. There are also tiny veins of ASM present sporadically in the quartz (Fig. 2c). The groundmass has a heterogeneous chemical composition, mainly due to Al and Fe segregation and K- and Pb-rich ASM in some parts. The chemical heterogeneity is also recorded in coupled substitutions including K vs. Pb. vs. REE at the D site and S vs. P + As at the T site [K+ + 2S6+ ↔ Pb2+ + (P,As)5+ + S6+; K+ + 2S6+ ↔ REE 3+ + 2(P,As)5+] (Table 2, 3, Fig. 3, Fig. 4).

Fig. 2. Back-scattered electron (BSE) images (a–h) and secondary electron (SE) image (i) of secondary assemblages from the Velence aplitic dyke. (a) Fine-grained matrix of jarosite (Jrs) and Pb-rich alunite-supergroup minerals (Pb-ASM) with quartz (Qtz) and scattered rhabdophane (Rhb) grains. (b) Vein of alunite-supergroup minerals (ASM) and iron oxyhydroxide (Gth) crosscutting feldspar (Fs) and quartz (Qtz). (c) Vein and cavity filled with ASM in quartz (Qtz). (d) Euhedral to subhedral rhabdophane crystals (Rhb) irregularly distributed in jarosite (Jrs) and Pb-rich ASM matrix. Other rock-forming minerals are represented by the large aggregate of muscovite (Ms) in the central part and quartz (Qtz). (e) Aggregate of rhabdophane crystals (Rhb) in jarosite (Jrs) matrix rich in Pb in some places (Pb-rich ASM), K-feldspar (Kfs) and quartz (Qtz). (f) Hexagonal symmetry of a solitary rhabdophane (Rhb) crystal embedded in heterogeneous sulfate. Lead-rich alunite, hinsdalite and corkite (Pb-rich ASM) form small elongated crystals scattered in massive jarosite (Jrs). (g) A euhedral crystal of rhabdophane showing hexagonal symmetry in botryoidal limonite/goethite (Gth). (h) Botryoidal goethite aggregate (Gth) and Al-rich goethite (Al-rich Gth) with rhabdophane crystals (Rhb) in the cavity. (i) SE image of the detailed area in (h) with rhabdophane protruding above the goethite aggregate (Gth and Al-rich Gth).

Fig. 3. False colour X-ray mapping showing the distribution of chemical elements in the (a–h) ASM matrix rich in rhabdophane. The image is the same as rotated Fig. 2e. (i–p) Euhedral rhabdophane crystal in botryoidal goethite. The image is the same as in Fig. 2g. False colours: blue – very low, green – low, yellow – medium, orange – high, red – very high.

Fig. 4. Composition of ASM with general formulae DG 3(TX 4)2X’6: (a) G site occupancy; (b) D site occupancy, Pb*=Pb + Ca + Ba + Sr; and (c) T site occupancy (atomic proportions).
Table 2. Representative compositions of EPMA of K-dominant ASM (in wt.% and apfu).
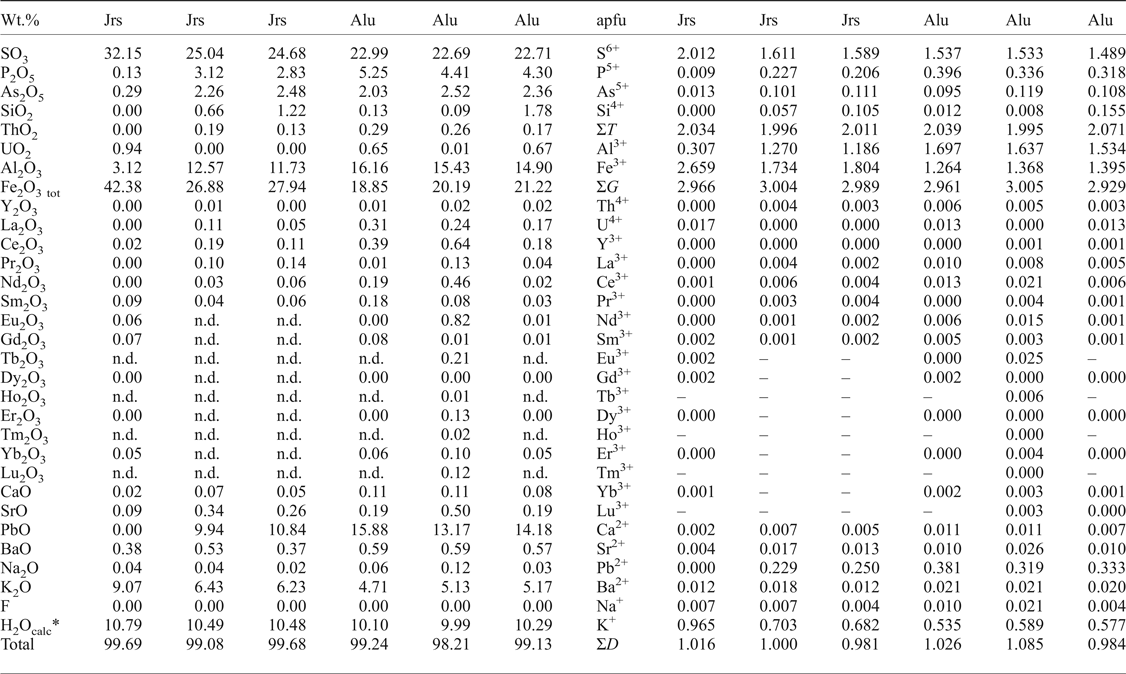
* H2O contents calculated on the basis of ideal stoichiometry.
Jrs = jarosite; Alu = alunite; n.d. = not detected.
Table 3. Representative compositions of EPMA of Pb-dominant ASM of the beudantite group (in wt.% and apfu).

*H2O contents calculated on the basis of ideal stoichiometry.
Cor = corkite; Hin = hinsdalite; n.d. = not detected.
The Fe concentrations range from 0.5 to 48 wt.% Fe2O3 (0.03–2.98 apfu Fe) and Al ranges from 1.8 to 29 wt.% Al2O3 (0.18–2.94 apfu Al); and thus the solid solution extends over almost the entire range of compositions at the G site (Fig. 4a). The D site content reveals major substitution between K and Pb, with values ranging from 0.9 wt.% K2O (0.1 apfu) to 9.3 wt.% K2O (1.00 apfu K) and 0.0 to 22 wt.% PbO (0.52 apfu Pb), respectively. Some analyses, especially those rich in Pb, include significant amounts of other divalent cations (0.22 apfu Ca + Ba + Sr), or 0.74 apfu Pb* (Pb + Ca + Ba + Sr) (Fig. 4b).
Sulfur is the dominant cation at the T site, ranging from 14 to 33 wt.% SO3 (0.98–2.00 apfu S), but Pb-rich ASM may have increased P (≤13 wt.% P2O5; ≤0.95 apfu P) and slightly increased As (≤3.3 wt.% As2O5; ≤0.14 apfu As) (Fig. 4c). Some analyses have negligible P + As (apfu) surplus compared to the bulk of divalent cations (Fig. 5a). This imbalance is compensated by an appropriate amount of REE; indicating minor presence of the florencite molecule [CeAl3(PO4)2(OH)6] (Fig. 5b). The total REE content never exceeds 10 wt.% REE 2O3 (0.37 apfu REE + Y) and it shows dominancy of light rare-earth elements (LREE, mainly Ce) over heavy rare-earth elements (HREE) in corkite and hinsdalite, whereas jarosite and alunite contain only negligible amounts of REE (Table 2 and 3).

Fig. 5. Composition of ASM in (a) P + As vs. Pb*; and (b) P + As vs. Pb*+REE + Y. Pb* = Pb + Ca + Ba + Sr (atomic proportions).
The ASM analyses reveal strong coupling of K (D site) with P + As (T site) and with Al (G site), Pb (D site) couples with S (T site) and Fe (G site) (Fig. 6). The Pb-rich member is preferentially Al-dominant (hinsdalite rather than corkite) and the K-rich member is preferentially Fe-dominant (jarosite rather than alunite) (Fig. 6). Thorium and uranium rarely reach 2.1 wt.% ThO2 + UO2 (0.04 apfu Th + U) and the concentrations of other trace elements such as Na, Mg and Si are negligible, and usually below the detection limit.

Fig. 6. Composition of ASM in Al/(Al + Fe) vs. (P + As)/(P + As + S). The selection of end-members containing P + As in the T site is restricted to Pb-dominant end-members of the beudantite and plumbogummite groups (atomic proportions).
Rhabdophane-group minerals
Rhabdophane-group phosphate minerals are represented by rhabdophane-(Ce), ideally CePO4·H2O, rhabdophane-(La) (LaPO4·H2O) and rhabdophane-(Nd) (NdPO4·H2O) end-members. They form euhedral to subhedral solitary crystals ~5 to 25 µm across and are irregularly scattered in the heterogeneous ASM matrix (Fig. 2d,e,f) or in botryoidal goethite (Fig. 2g,h). Some parts of the ASM matrix are richer in REE + Th, where rhabdophane forms sporadic aggregates of dozens of crystals (Fig. 2e, 3a–h). Most crystals are hexagonal. Oscillatory zoning and differential leaching of minerals results in an uneven surface where the more resistant rhabdophane protrudes above the ASM and goethite (Fig. 2h,i).
All reproducible analyses returned low oxide totals; mostly 90 to 95 wt.% (Table 4). The low totals suggest the presence of water in the structure and this is confirmed by Raman spectroscopy. Rhabdophane has a predominance of LREE over HREE; with Ce, La and Nd the dominant LREE cations (Fig. 3a, i–k).
Table 4. Representative compositions of EPMA of rhabdophane-group minerals (in wt.% and apfu).
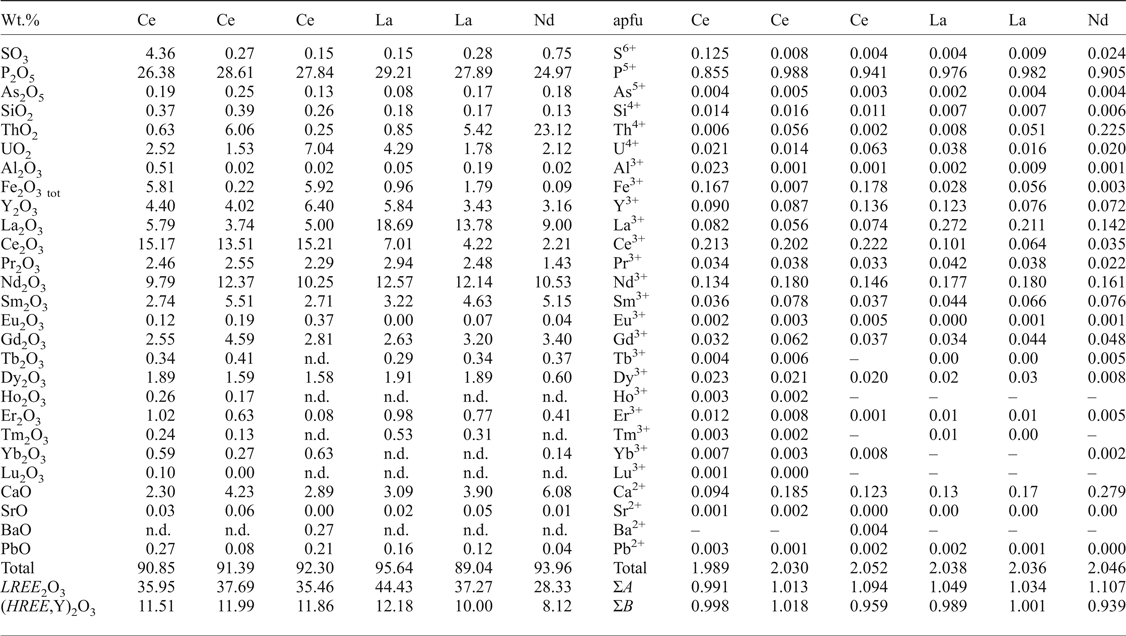
n.d. = not detected.
The distribution of Ce, La and Nd in rhabdophane generally clusters in the following groups: group I is characterized by low La content (0.03–0.10 apfu La) and slight predominance of Ce over Nd (0.12–0.27 apfu Ce; 0.11–0.21 apfu Nd) (Fig. 7) in most cases, but a composition with Nd~Ce>>La (0.17 apfu Nd, 0.17 apfu Ce, 0.06 apfu La) was noted in one case (Fig. 7); group II has low Ce (0.03–0.11 apfu Ce) content and slight predominance of La over Nd (0.14–0.28 apfu La; 0.11–0.18 apfu Nd) in most cases, but a composition with Nd > La>>Ce (0.16 apfu Nd, 0.14 apfu La, 0.04 apfu Ce) was noted also in one case (Fig. 7). The majority of rhabdophane crystals show La–Nd enrichment in the core (group II) and Ce enrichment in the distal zones (group I) (Fig. 3i–k).

Fig. 7. Ternary diagram Ce–La–Nd showing rhabdophane end-members and two different groups with contrasting Ce, La and Nd distributions (atomic proportions).
The Y content is relatively high with a maximum value of 8.3 wt.% Y2O3; 0.17 apfu Y and HREE can attain 9.6 wt.% HREE 2O3; 0.13 apfu HREE, and the increased Y + HREE content suggests less of a miscibility gap between LREE and Y + HREE in rhabdophane-group minerals.
Measurements also confirm relatively high actinide contents (≤25 wt.% ThO2; 0.24 apfu Th and ≤8.6 wt.% UO2; and 0.07 apfu U) and significantly elevated Ca, Fe and S (≤8.2 wt.% CaO; 0.33 apfu Ca, ≤7.6 wt.% Fe2O3tot, 0.23 apfu Fe and ≤4.4 wt.% SO3; and 0.13 apfu S, respectively), Table 4. The presence of Th, U, Ca and minor Fe and S (Fig. 3c,e,h,m–p) indicate more complex chemical composition and intermediate solid solution in RGM. Most analyses also show a trend between brockite and tristramite tie lines which may indicate that Th, U, Ca are commonly incorporated in the rhabdophane structure via CaThREE –2 (Ca2+ + Th4+ ↔ 2REE 3+) and CaUREE –2 (Ca2+ + U4+ ↔ 2REE 3+) substitution mechanisms (Fig. 8). The RGM also have relatively low Pb content (≤1.3 wt.% PbO; ≤0.014 apfu Pb; Table 4), which contrasts with high Pb values in the ASM and indicates a minimal grayite PbThREE –2 (Pb2+ + Th4+ ↔ 2REE 3+) substitution.

Fig. 8. Composition of rhabdophanes in Ca + U vs. REE + Y substitution diagram (atomic proportions) with ideal grayite (PbThREE –2), brockite (CaThREE –2) and tristramite (CaUREE –2) tie-lines. Global occurrences of rhabdophane-group minerals are plotted for comparison. Note: *Buchholz et al. (Reference Buchholz, Falster and Simmons2010) data from pers. comm. **Compositions by Zidarov and Petrov (Reference Zidarov and Petrov2011) are published as grayite, but atomic ratio Ca:Pb = 5:1; Ca:Th = 1:1 classifies it as brockite.
In addition, incorporation of Si is very low (≤0.59 wt.% SiO2; 0.02 apfu Si) indicating a negligible ThSiREE –1P–1 (Th4+ + Si4+ ↔ REE 3+ + P5+) huttonite-like substitution. Rhabdophane crystals are preferentially rich in the brockite molecule in their central parts (Fig. 3h, m–n) and tristramite is present in the outer parts (Fig. 3o).
Raman spectroscopy
The jarosite Raman spectrum (Table 5, Fig. 9) is interpreted according to Frost et al. (Reference Frost, Wills, Weier, Martens and Mills2006a, Reference Frost, Palmer and Xi.2011), and contains lattice vibrations at 141 cm–1. Bands between 300 and 400 cm–1 are attributed to M–O (Fe–O) stretching, those between 433 and 452 cm–1 can be described as split (SO4)2– ν2 symmetric bending modes and bands at 626 and 638 cm–1 correspond to ν4 (SO4)2– antisymmetric bending modes. The strong Raman band at 1009 cm–1 results from ν1 (SO4)2– symmetric stretching and the two bands at 1105 and 1156 cm–1 may be due to ν3 antisymmetric stretching in both (SO4)2– and the substituting (PO4)3– tetrahedra.

Fig. 9. Raman spectra of the jarosite investigated: (a) 100–1200 cm–1 region; (b) 3000–4000 cm–1 region. The jarosite studied in the 100–1200 cm–1 region is compared to jarosite and corkite (Lafuente et al., Reference Lafuente, Downs, Yang, Stone, Armbruster and Danisi2015). The measured band in the 3000–4000 cm–1 region is deconvoluted into 3 bands. The asterisk marks the spectral artefact.
Table 5. Raman bands of the jarosite studied compared to other alunite-supergroup minerals. Band assignments were made according to Serna et al. (Reference Serna, Parada-Cortina and García-Ramos1986), Frost and Palmer (Reference Frost and Palmer2011) and Frost et al. (Reference Frost, Wills, Weier and Martens2005, Reference Frost, Wills, Weier, Martens and Mills2006a, Reference Frost, Wills, Weier, Martens and Kloprogge2006b, Reference Frost, Palmer and Xi.2011).

Jrs = jarosite; HJrs = hydroniumjarosite; PbJrs = plumbojarosite; Alu = alunite; Hin = hinsdalite; Cor = corkite.
The presence of water is indicated by characteristic bands; OH···H hydrogen-bond vibrations in the 100–1200 cm–1 region have a strong band at 224 cm–1 but the possible OH deformations at 559 and 579 cm–1 have also been interpreted as M–O (Fe–O) stretching. Additional O–H stretching vibrations are observed in a relatively broad band which can be resolved into bands at 3366 (very broad), 3378 (sharp), 3411 (sharp) and 3449 (broad) cm–1.
The rhabdophane Raman spectrum contains lattice vibrations between 113 and 415 cm–1 and bond vibrations of (PO4)3– tetrahedra between 464 and 1038 cm–1 (Table 6, Fig. 10). A relatively sharp band was also measured at 3297 cm–1 and although this is surrounded by luminescence features, it has a different shape to those at 113 and 415 cm–1. This may be due to water or intergrowing goethite although no goethite bands were observed in the 100–1200 cm–1 region. All bands at this region can be assigned to rhabdophane on the basis of comparisons with synthetic rhabdophane-(La) and monazite-(Ce) (R040106, RRUFF database) spectra. Consequently, the 3297 cm–1 band is attributed to H2O in the rhabdophane structural channels.

Fig. 10. Raman spectra of the rhabdophane investigated: (a) 100–1200 cm–1 region; (b) 2800–3800 cm–1 region. The rhabdophane studied in the 100–1200 cm–1 region is compared to synthetic rhabdophane-(La) and monazite-(Ce). Dashed lines document the Raman band shift at 960 cm–1 in the rhabdophane spectrum to lower values relative to monazite-(Ce).
Table 6. Raman bands of the rhabdophane investigated compared to synthetic rhabdophane-(La) and monazite-(Ce)*.
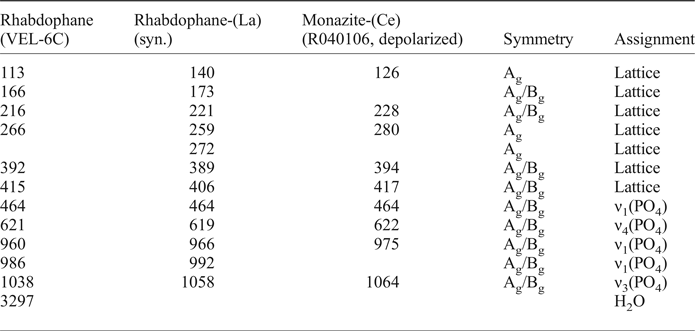
* Symmetry and band assignment are according to Silva et al. (Reference Silva, Ayala, Guedes, Paschoal, Moreira, Loong and Boatner2006).
Discussion
ASM and RGM compositional variations
The ASM aggregates in the Velence aplite dyke are predominantly fine grained. The host jarosite matrix is characteristically massive and intimately intergrown with cryptocrystalline aggregates of Pb-rich jarosite/alunite and sporadic hinsdalite/corkite which may have resulted from solid-state diffusion segregation (Desborough et al., Reference Desborough, Smith, Lowers, Swayze, Hammarstrom, Diehl, Leinz and Driscoll2010). The fine-grained ASM appears limited to near-surface low-temperature supergene environments (Stoffregen et al., Reference Stoffregen, Alpers, Jambor, Alpers, Jambor and Nordstrom2000) and is sporadically hosted on granitic and pegmatitic bedrock (Dill, Reference Dill2001). There is no evidence for the occurrence of plumbojarosite or its hypothetical Al-analogue ‘plumboalunite’ Pb0.5Al3(SO4)2(OH)6, but lead-rich alunite with composition close to K0.5Pb0.5Al3(SO4)1.5(PO4)0.5(OH)6 has been identified, the substitutions at the D and T sites being required for charge balance (cf. Scott, Reference Scott1987).
Raman spectroscopy proved that the major jarosite phase has a spectrum similar to K-dominant jarosite although it was measured in the Pb-enriched area of the sample. Compared to the sample studied, the alunite spectra are moved to higher Raman-shift values due to the dominance of Al over Fe (Table 5, Frost et al., Reference Frost, Wills, Weier, Martens and Mills2006a,Reference Frost, Wills, Weier, Martens and Kloproggeb). Similarly, the spectrum studied displays band shifts compared to the hinsdalite spectrum (Frost et al., Reference Frost, Palmer and Xi.2011). However, it is not possible to exclude plumbojarosite and hydroniumjarosite exclusively on the basis of Raman spectroscopy, and the extensive splitting of M–O stretching and ν2(SO4)2– symmetric bending suggests a partly mixed composition of these end-members because Raman bands in the OH-stretching share similarities with both jarosite and hydroniumjarosite (Frost et al., Reference Frost, Wills, Weier, Martens and Mills2006a).
Raman spectroscopy was also used to distinguish rhabdophane from monazite. The spectra of both minerals display many similarities due to their similar crystal-chemical properties. Both contain PO4 tetrahedra interconnected by Ce coordinated by eight oxygens.
However, there are small structural differences between them. The monazite structure has regular alternating PO4 tetrahedra and CeO8 polyhedra (Ni et al., Reference Ni, Hughes and Mariano1995), this regularity is disrupted in rhabdophane which has tunnels parallel to the c axis which can accommodate water (Mooney, Reference Mooney1950). Consequently, the environment of PO4 in rhabdophane is slightly different to monazite. The P–O bond length increases from 1.52–1.54 Å in monazite (Ni et al., Reference Ni, Hughes and Mariano1995) to 1.56 Å in rhabdophane (Mooney, Reference Mooney1950). Vibrations in the PO4 tetrahedron can be used to differentiate rhabdophane and monazite.
Most rhabdophane crystals in the Velence aplite dyke are euhedral hexagonal prisms which are rarely observed due to predominantly indistinguishable crystals forming massive and cryptocrystalline earthy aggregates, mammillary rounded forms, encrustations or pseudomorphs (cf. Hildebrand et al., Reference Hildebrand, Carron and Rose1957; Fisher and Meyrowitz, Reference Fisher and Meyrowitz1962; Atkin et al., Reference Atkin, Basham and Bowles1983; Nagy and Draganits, Reference Nagy and Draganits1999; Takai and Uehara, Reference Takai and Uehara2012; Matýsek, Reference Matýsek2013). Chemically, they are typically Ce dominant, but in some La or Nd are dominant. Compositions dominant in La and Nd have also been documented in rhabdophane encrustations on limonitic ores (Hildebrand et al., Reference Hildebrand, Carron and Rose1957). Similarly, rhabdophane from the type locality at Fowey Consols Mine in Cornwall also shows Nd >La≈Ce (Bowles and Morgan, Reference Bowles and Morgan1984). The deficiency in Ce relative to La and Nd can be explained by formation in a strongly oxidizing environment which causes fractionation of REE by oxidation of Ce3+ to Ce4+ (Fisher and Meyrowitz, Reference Fisher and Meyrowitz1962; Banfield and Eggleton, Reference Banfield and Eggleton1989; Demartin et al., Reference Demartin, Pilati, Diella, Donzelli and Gramaccioli1991). Cerium tends to become fixed in its quadrivalent state, and is not readily mobilized during weathering (Fisher and Meyrowitz, Reference Fisher and Meyrowitz1962). Another mechanism involves selective adsorption of Ce on iron oxyhydroxides (Bau and Koschinsky Reference Bau and Koschinsky2009; Vodyanitskii, Reference Vodyanitskii2012). Negative Ce anomalies are rare in minerals and rocks, but mainly occur in very oxidized weathered environments (e.g. Sawka et al., Reference Sawka, Banfield and Chappell1986; Braun et al., Reference Braun, Pagel, Muller, Bilong, Michard and Guillet1990; Krenn and Finger, Reference Krenn and Finger2007).
The rhabdophane compositions have a significantly higher Y and HREE content than comparable monazite, where there is usually a wide miscibility gap between LREE and HREE. This appears a typical RGM feature, several authors have reported high Y and HREE contents (e.g. Bowles and Morgan, Reference Bowles and Morgan1984; Nagy et al., Reference Nagy, Draganits, Demény, Pantó and Arkai2002; Krenn and Finger, Reference Krenn and Finger2007; Čopjaková et al., Reference Čopjaková, Novák and Franců2011; Andersen et al., Reference Andersen, Clark, Larson and Neill2016). Rhabdophane-(Y) with the empirical formula (Y0.33La0.31Nd0.21Pr0.08Gd0.05Ce0.02Sm0.00)1.01Ca0.09(P0.96O4)·1.15H2O has been found in a miarolitic cavity of the Higashimatsuura basalt, Japan (Takai and Uehara, Reference Takai and Uehara2012) and a synthetic equivalent of rhabdophane-(Y) has also been prepared (Mogilevsky and Boakye, Reference Mogilevsky and Boakye2007).
Although some authors claim that REE are not readily fractionated under supergene conditions (e.g. Nance and Taylor, Reference Nance and Taylor1977; Hanson, Reference Hanson1980), our results support the premise that some exogenic processes, especially supergene alteration and granite weathering in low pH and early diagenesis, may be responsible not only for significant REE (LREE) mobility but also for their fractionation and precipitation of mineral phases with Nd- and/or La-dominant compositions instead of the most common Ce-dominant members (cf. Scharmová and Scharm, Reference Scharmová and Scharm1994; Čopjaková et al., Reference Čopjaková, Novák and Franců2011; Santana et al., Reference Santana, Wall and Botelho2015).
The RGM described herein are rich in minor elements including Ca, Th, U, Fe and S and represent an uncommon rhabdophane–tristramite–brockite solid solution with the most extensive compositional variation recorded. This unique series of analyses of RGM invites questions about the substitutions, our data suggest, that the incorporation of Ca, Th, U and S is controlled by substitution mechanisms comparable to those in monazite-group minerals (MGM) including CaThREE −2 (Ca2+ + Th4+ ↔ 2REE 3+) analogous to cheralite (Ca0.5Th0.5PO4) (cf. Fisher and Meyrowitz, Reference Fisher and Meyrowitz1962), CaUREE –2 (Ca2+ + U4+ ↔ 2REE 3+) analogous to a hypothetical Ca0.5U0.5PO4 MGM end-member and CaS(REE)−1P−1 (Ca2+ + S6+ ↔ REE 3+ + P5+) analogous to ‘clinoanhydrite substitution’ (cf. Ondrejka et al., Reference Ondrejka, Uher, Pršek and Ozdín2007; Pršek et al., Reference Pršek, Ondrejka, Bačík, Budzyń and Uher2010) where S6+ balances the charge requirements in Ca-rich but Th-poor RGM.
Trivalent iron entry to the RGM structure appears to be controlled by simple uncoupled and homovalent FeREE –1 (Fe3+ ↔ REE 3+) substitution, the difference in the ionic radii of Fe3+ and LREE 3+ is probably the limiting factor for Fe incorporation in monazite; but not for hexagonal RGM. The incorporation of divalent Fe via coupled heterovalent FeThREE –2 (Fe2+ + Th4+ ↔ 2REE 3+) substitution in the rhabdophane structure and the existence of the hexagonal Fe0.52+Th0.5PO4·H2O end-member is discussed by Kucha (Reference Kucha1979) and Kucha and Wieczorek (Reference Kucha and Wieczorek1980). Elevated Fe2O3 contents of up to 4.5 wt.% in rhabdophane-(Ce) from the Drahany Upland low-grade meta-sediments was reported by Čopjaková et al. (Reference Čopjaková, Novák and Franců2011); but these authors interpreted this Fe content as an admixture of the Fe-oxides-hydroxides commonly intimately associated with rhabdophane. Iron in RGM has been reported in brockite from the Wet Mountains, Colorado, USA, but this was considered an impurity and omitted from the calculation (Fisher and Meyrowitz, Reference Fisher and Meyrowitz1962), and 6.0 wt.% Fe2O3; 0.17 apfu Fe content was reported in tristramite from Wheal Trewavas, Cornwall, UK (Atkin et al., Reference Atkin, Basham and Bowles1983). Finally, tristramite from a sandstone-type uranium deposit located in Erlian basin of Inner Mongolia, China displays an average FeO content of 0.8 wt.% (Guang et al., Reference Guang, Xiang-kum and A-peng2013). All these data together with Fe-rich RGM from the Velence Hills suggest that the incorporation of Fe2+ and Fe3+ in the RGM is possibly via above-mentioned substitution mechanisms but does not produce a valid end-member and shouldn't be included in formula calculation. The low concentration of Si in our RGM analyses indicates a negligible ThSiREE –1P–1 (Th4+ + Si4+ ↔ REE 3+ + P5+) heterovalent mechanism, analogous to huttonite (ThSiO4) which presents another possible monazite-like substitution involved in RGM (cf. Buchholz et al., Reference Buchholz, Falster and Simmons2010).
The presented intermediate chemical composition of RGM from Velence aplite dyke shows that continuity in the rhabdophane–brockite–tristramite series does exist and indicates also that the arrangement of substitution vectors among RGM end-members corresponds to MGM. To summarize, end-member formulae of RGM with dominant divalent and tetravalent cations (brockite, tristramite and grayite), where A 2+ = Ca, Pb; A 4+ = Th, U, can be expressed following the principles of MGM where A 2+ + A 4+ substitute for 2A 3+ (e.g. Ca2+ + Th4+ ↔ 2REE 3+ in cheralite, Ca0.5Th0.5PO4), whereas P5+ exclusively occupies the B site. Accordingly, proposed end-member formulae of divalent and tetravalent RGM are as follows: (Ca0.5Th0.5)PO4·H2O for brockite, (Pb0.5Th0.5)PO4·H2O for grayite and (Ca0.5U0.5)PO4·2H2O for tristramite.
Origin of ASM and RGM
The diverse environments documented for ASM formation near the Earth's surface show that there are numerous possible sources of the elements required for this mineral group (Gaboreau et al., Reference Gaboreau, Beaufort, Viellard and Patrier2005). Rhabdophane can precipitate during weathering and low-temperature alteration of REE-Th-bearing minerals, including apatite (Sawka et al., Reference Sawka, Banfield and Chappell1986; Banfield and Eggleton, Reference Banfield and Eggleton1989; Muramaki et al., Reference Murakami, Utsunomiya, Imazu and Prasad2001), monazite (Nagy et al., Reference Nagy, Draganits, Demény, Pantó and Arkai2002; Berger et al., Reference Berger, Gnos, Janots, Fernandez and Giese2008) and allanite (Krenn and Finger, Reference Krenn and Finger2007).
The described mineral assemblage of ASM and RGM precipitated as a result of extensive chemical alteration and leaching of the primary magmatic minerals in the microgranite by hydrothermal sulfate-rich, highly acidic aqueous solutions, connected with the intrusion of adjacent Palaeogene andesitic–dioritic rocks. Hydrothermal mineralization of epithermal, high-sulfidation type formed dyke-like bodies and veinlets with sulfide minerals (mainly pyrite, enargite and chalcopyrite) in the eastern part of the Velence Hills, near the Permian granitic rocks (Molnár, Reference Molnár2004). Moreover, the hydrothermal centres are characterized by silica bodies surrounded by alunite–kaolinite and pyrophyllite–dickite–kaolinite–diaspore–alunite alteration and occurrences of topaz, zunyite and native sulfur (Horváth et al., Reference Horváth, Daridáné Tichy, Dudko, Gyalog and Ódor2004). Here, the alunite crystals are rich in Na and contain beudantite-group minerals (woodhouseite and svanbergite) in their cores (Bajnóczi et al., Reference Bajnóczi, Molnár, Maeda, Nagy and Vennemann2002; Molnár, Reference Molnár2004).
The source of K, Ca, Sr and Ba in ASM could be from hydrolysis of alkali feldspars, with sulfuric acid produced by the oxidation of massive sulfides (cf. Dill, Reference Dill2001). Hydrothermal sulfides are the most probable source of Pb and sulfates. Rare-earth elements, especially LREE in RGM and ASM may be produced by the alteration of allanite-(Ce), the most widespread primary magmatic REE phase in the Velence granitic rocks (Uher and Broska, Reference Uher and Broska1994; Buda and Nagy, Reference Buda and Nagy1995). However, Y + HREE enrichment in the rhabdophanes suggests that other possible primary magmatic mineral(s), mainly xenotime-(Y), are a possible source.
Conclusions
An assemblage of alunite-supergroup minerals (ASM) consisting of jarosite, Pb-rich jarosite and alunite, corkite and hinsdalite and Ce- La- and Nd-dominant members of the rhabdophane series occur in Permian A-type porphyritic microgranite in the Velence Hills, Hungary. Compositional variations and substitution trends indicate that Th, U, Ca and Pb are incorporated into the rhabdophane structure by a (Ca, Pb)2+ + (Th, U)4+ ↔ 2REE 3+ substitution mechanism. Consequently, we suggest the following end-member formulae for species containing divalent and tetravalent cations: (Ca0.5Th0.5)PO4·H2O for brockite, (Pb0.5Th0.5)PO4·H2O for grayite and (Ca0.5U0.5)PO4·2H2O for tristramite.
The formation of this mineral assemblage is associated with low-temperature alteration of the host granite and its primary magmatic minerals by sulfate-rich, highly acidic aqueous hydrothermal solutions, associated with the intrusion of Palaeogene andesitic–dioritic rocks. The occurrence and chemical composition of RGM suggest remobilization and fractionation of lanthanides (mostly LREE) during low-temperature processes and incorporation of Ca, Th, U, Fe and S in the RGM structure in a continuous rhabdophane–tristramite–brockite solid solution. The source of remobilized REE, Th, U and P is probably the chemically leached accessories allanite-(Ce) and fluorapatite, possibly with xenotime-(Y).
Acknowledgements
This work was supported by the Slovak Research and Development Agency under contracts APVV-14-0278, APVV-15-0050, VEGA Agency Nos. 1/0257/13, 1/0079/15 and 1/0499/16 and by the European Regional Development Fund through the projects: ITMS 26220120064 and ITMS 26210120013. We thank I. Holický and V. Kollárová for providing the EPMA facilities and R.J. Marshall and D. Green for reviewing the English content. Finally we thank D. Green, and D. Atencio (Reviewers), M. Rumsey (Associate Editor) and P. Williams and S. Mills (Principal Editors) for their constructive suggestions.