Introduction
The liver flukes comprise 2 families of food-borne trematodes that cause diseases in humans and animals: Opisthorchiidae (which includes Clonorchis sinensis and Opisthorchis viverrini) and Fasciolidae (which includes Fasciola hepatica). These parasites cause infection via the consumption of contaminated raw fish, crustaceans or vegetation. While infections are generally asymptomatic, higher worm burdens and/or continuous reinfection can cause severe liver disease (Haswell et al., Reference Haswell-Elkins, Mairiang, Mairiang, Chaiyakum, Chamadol, Loapaiboon, Sithithaworn and Elkins1994; Kaplan, Reference Kaplan2001; Valero et al., Reference Valero, Gironès, García-Bodelón, Periago, Chico-Calero, Khoubbane, Fresno and Mas-Coma2008; Kim et al., Reference Kim, Choi, Bae, Oh, Lim and Hong2011). Infection with C. sinensis and O. viverrini can result in the mineralization of bile ducts (cholangitis), formation of bile duct stones and a sub-type of liver cancer: cholangiocarcinoma (CCA), which is an adenocarcinoma with poor prognosis (Fried et al., Reference Fried, Reddy and Mayer2011; Lim, Reference Lim2011; de Martel et al., Reference de Martel, Ferlay, Franceschi, Vignat, Bray, Forman and Plummer2012). Fasciolosis is similarly associated with the development of cholangitis. However, despite being phylogenetically related to Opisthorchiidae, and ultimately residing in the same tissue of their mammalian hosts, infection with F. hepatica is not associated with the development of any cancers (Kaya et al., Reference Kaya, Beştaş and Cetin2011; Machicado et al., Reference Machicado, Machicado, Maco, Terashima and Marcos2016, Reference Machicado, Bertani, Herrera-Velit, Espinoza, Ruiz and Marcos2018).
Life cycle of the liver flukes
As with most trematodes, the liver flukes have a complex life cycle, requiring both intermediate and primary hosts (Fig. 1). The eggs of these parasites discharge with feces from their primary mammalian host, and then progress through several developmental stages in an aquatic snail intermediate host. Free-swimming cercariae, which are released from the snails, then encyst as metacercariae; the stage that is infectious to the definitive mammalian hosts. For C. sinensis and O. viverrini, this encystment occurs within the muscles or under the scales of freshwater fish, thereby making fish the secondary intermediate host and the vehicle for human infection. The metacercariae of F. hepatica encyst on aquatic vegetation (such as watercress), and are transmitted to human and animal hosts after ingestion of infected plants. Differences in the intermediate hosts underpin the distinct global distribution for each of these food-borne trematodes. Specifically, while the snail hosts for F. hepatica (Lymnaeidae family) are found in almost every country worldwide, the snail hosts for Clonorchis and Opisthorchis have a more restricted global distribution (Lu et al., Reference Lu, Gu, Limpanont, Song, Wu, Okanurak and Lv2018). In addition, the eating habits of populations around the world confer different susceptibilities to infection. For example, consuming raw (dried, fermented or salted) or undercooked fish is a common practice throughout Asia, and in the far eastern regions of the former Soviet Union (Sripa et al., Reference Sripa, Kaewkes, Intapan, Maleewong and Brindley2010). In contrast, the consumption of aquatic plants by animals and humans occurs worldwide. Because of these dietary variations, F. hepatica has been found in all inhabited continents (Mas-Coma et al., Reference Mas-Coma, Valero and Bargues2009; Lu et al., Reference Lu, Gu, Limpanont, Song, Wu, Okanurak and Lv2018), whereas infection with C. sinensis is only endemic in China, Korea and Vietnam, and O. viverrini is predominantly found in Thailand, Lao People's Democratic Republic, Cambodia and central Vietnam (Sripa et al., Reference Sripa, Kaewkes, Intapan, Maleewong and Brindley2010; Lu et al., Reference Lu, Gu, Limpanont, Song, Wu, Okanurak and Lv2018). Opisthorchis felineus is the predominant species found in Siberia, and like O. viverrini it is also suspected of being a group 1 carcinogen (Fedorova et al., Reference Fedorova, Fedotova, Zvonareva, Mazeina, Kovshirina, Sokolova, Golovach, Kovshirina, Konovalova, Kolomeets, Gutor, Petrov, Hattendorf, Ogorodova and Odermatt2020). This variation in dietary practices also introduces additional risk factors for the development of CCA, which may influence the differential outcome to infection with liver flukes. Salted and fermented fish contain high levels of nitrosamines, which are classified as carcinogenic factors. Their consumption may create a microenvironment that is more favourable to the development of malignancies, such as CCA, following infection with Clonorchis or Opisthorchiidae (Steele et al., Reference Steele, Richter, Echaubard, Saenna, Stout, Sithithaworn and Wilcox2018).
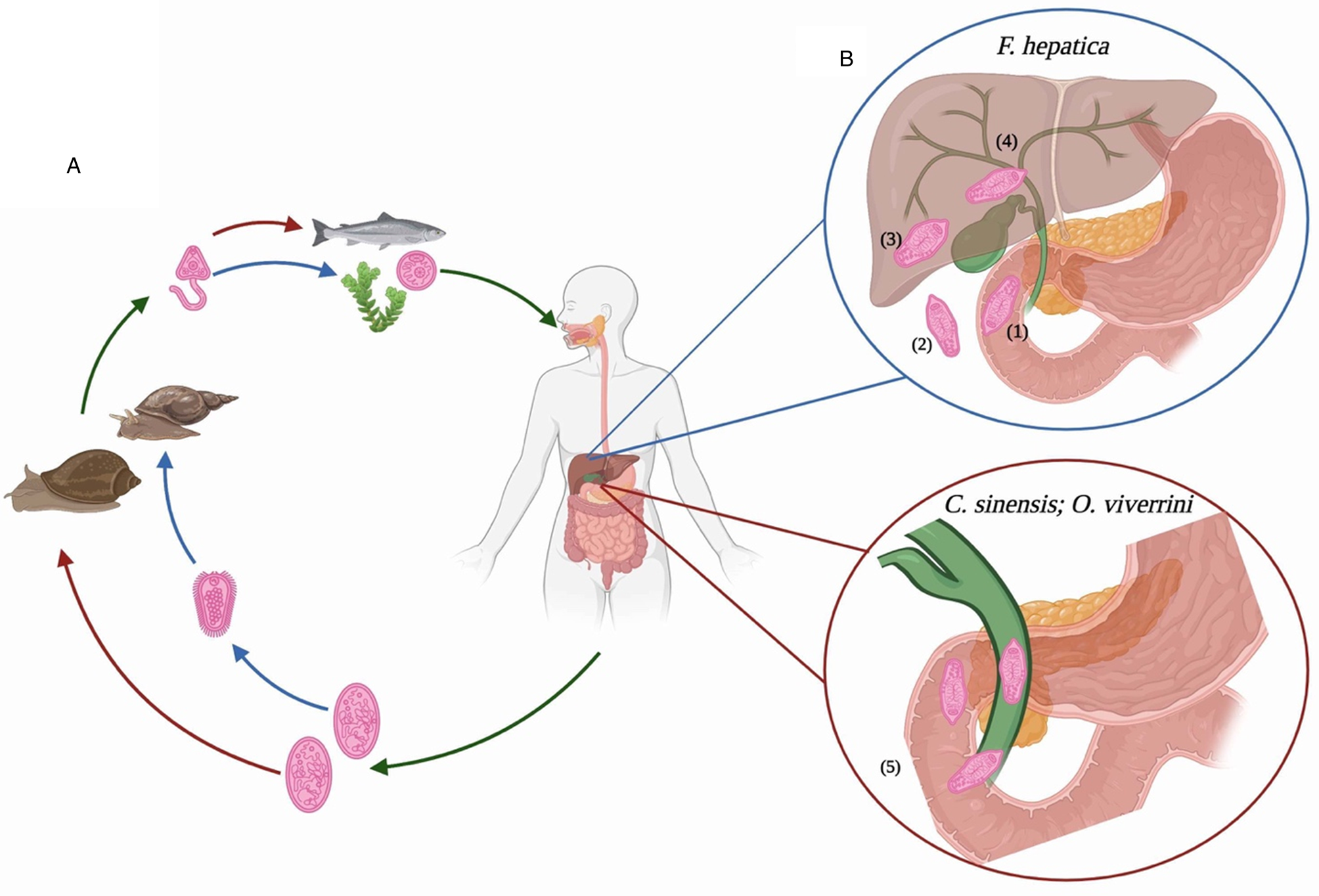
Fig. 1. Comparative life cycles and intra-mammalian migratory pathways of food-borne liver flukes. (A) All adult flukes produce eggs that are passed with feces from the mammalian host. For Fasciola hepatica, these eggs become embryonated in fresh water to release miracidia, which then invade a suitable snail host. In contrast, for Opisthorchis viverrini and Clonorchis sinensis this intermediate stage begins when the snail ingests the unembryonated eggs. Once inside the snail, the parasite undergoes several developmental stages before emerging as cercariae. These cercariae must encyst (on vegetation for F. hepatica; within freshwater fish for O. viverrini and C. sinensis) to become metacercariae; the infective stage for mammalian hosts. (B) After ingestion, the environment within the digestive system of the mammalian host promotes excystment of the metacercariae: (1) the emergent juvenile flukes begin the migratory journey to the bile duct. To achieve this, (2) the F. hepatica flukes penetrate the intestinal epithelia to enter the abdominal cavity. (3) After a period of days, the juvenile flukes begin to tunnel through the liver parenchyma. Once inside this tissue the parasites spend time feeding and maturing, (4) finally reaching the bile ducts approximately 12 weeks after infection. (5) In contrast, O. viverrini and C. sinensis travel a more direct route to their destination. After emerging from the metacercariae, the juvenile flukes ascend directly to the bile ducts from the duodenum, via the ampulla of Vater. There, after a period of 3–4 weeks they mature into egg-laying adults, thereby restarting the parasite life cycle. Image created using BioRender.
After ingestion of the metacercariae by mammalian hosts, a series of stimuli (including temperature, pH and bile salts) in the digestive tract activates the excystment of the newly excysted juvenile (NEJ) worms (Andrews, Reference Andrews and Dalton1999; Sithithaworn et al., Reference Sithithaworn, Sripa, Kaewkes, Nawa, Haswell, Farrar, Hotez, Junghanss, Kang, Lalloo and White2014; Cwiklinski et al., Reference Cwiklinski, Jewhurst, McVeigh, Barbour, Maule, Tort, O'Neill, Robinson, Donnelly and Dalton2018; Lalor et al., Reference Lalor, Cwiklinski, Calvani, Dorey, Hamon, Corrales, Dalton and De Marco Verissimo2021). At this developmental stage, Opisthorchiidae migrate to the ampulla of Vater and ascend into the bile ducts where the parasites mature (Fig. 1). The adults of these parasites typically reside in the intrahepatic bile ducts for 10 years (Attwood and Chou, Reference Attwood and Chou1978; Ramsay et al., Reference Ramsay, Sithithaworn, Prociv, Moorhouse and Methaphat1989). Although the destination of the Fasciola parasites is also the bile duct, their migratory route is quite different (Fig. 1). After penetrating the intestinal epithelia, the NEJs migrate through the peritoneal cavity to the liver, where over several weeks, they tunnel a path towards the bile duct (Mas-Coma et al., Reference Mas-Coma, Valero and Bargues2014). Like C. sinensis and O. viverrini, the adult F. hepatica parasites can also remain in the bile ducts for up to 12 years after infection (Durbin, Reference Durbin and Otto1952; Radostits et al., Reference Radostits, Gay, Hinchcliff and Constable2007). This review examines the possibility that variations in the migratory patterns of the liver flukes differentially influence the polarization of macrophages, which consequently mediates the distinct pathological outcomes to infection.
Polarization and functional activity of macrophages
Macrophages are both tissue resident and infiltrating immune cells that are critical for the innate immune response, repair of damaged tissue, systemic metabolism, cold adaptation and tissue homoeostasis and development (Cox et al., Reference Cox, Pokrovskii, Vicario and Geissmann2021). The initiation of each of these biological activities occurs in response to the composition of the local environment in which the macrophages reside, and the type of pathogen or injury to which macrophages are exposed (Nobs and Kopf, Reference Nobs and Kopf2021).
Changes in the tissue microenvironment stimulate the polarization of differentiated tissue-resident macrophages into a diverse range of functional phenotypes (Cox et al., Reference Cox, Pokrovskii, Vicario and Geissmann2021; Wen et al., Reference Wen, Lambrecht, Ju and Tacke2021). When numbers of resident macrophages are insufficient to satisfy the functional demands within a tissue, the population can be expanded by local proliferation or recruitment. In addition, monocytes (macrophage precursors) can be recruited from the circulation to differentiate into functional macrophages within the affected tissues (Cox et al., Reference Cox, Pokrovskii, Vicario and Geissmann2021).
The polarization of macrophages into different phenotypes is a dynamic process, which occurs continuously throughout the inflammatory response to infection or tissue damage. This plasticity is a hallmark of macrophages and enables them to continuously respond to a changing microenvironment. This differentiation of macrophages requires an accurate regulation of gene transcription that is dependent on epigenetic modifications (Chen et al., Reference Chen, Yang, Wei and Wei2020). Dynamic and reversible changes of epigenetic markers at the promoters and enhancers of signal-sensitive genes are critical for the quick reprogramming of macrophage polarization and give macrophages the ability to switch rapidly between cellular programmes (Ghisletti et al., Reference Ghisletti, Barozzi, Mietton, Polletti, De Santa, Venturini, Gregory, Lonie, Chew, Wei, Ragoussis and Natoli2010). In addition, some signals (host and pathogen) can induce a more persistent ‘epigenetic memory’, which endows macrophages with a long-lasting capacity to respond more strongly to future challenges (Netea et al., Reference Netea, Domínguez-Andrés, Barreiro, Chavakis, Divangahi, Fuchs, Joosten, van der Meer, Mhlanga, Mulder, Riksen, Schlitzer, Schultze, Stabell Benn, Sun, Xavier and Latz2020). Thus, monocytes/macrophages emerging from the bone marrow are functionally conditioned to execute an enhanced (trained) or restricted (tolerant) response to subsequent restimulation (Divangahi et al., Reference Divangahi, Aaby, Khader, Barreiro, Bekkering, Chavakis, van Crevel, Curtis, DiNardo, Dominguez-Andres, Duivenvoorden, Fanucchi, Fayad, Fuchs, Hamon, Jeffrey, Khan, Joosten, Kaufmann, Latz, Matarese, van der Meer, Mhlanga, Moorlag, Mulder, Naik, Novakovic, O'Neill, Ochando, Ozato, Riksen, Sauerwein, Sherwood, Schlitzer, Schultze, Sieweke, Benn, Stunnenberg, Sun, van de Veerdonk, Weis, Williams, Xavier and Netea2021).
Each phenotype of macrophage is inherently linked to the activation of specific metabolic and molecular pathways, which ultimately determine the biological function of each subtype of macrophage (O'Neill and Pearce, Reference O'Neill and Pearce2016; Murray, Reference Murray2017). Early investigations to determine the mechanisms underlying macrophage polarization used models of dichotomous macrophage phenotypes, namely pro-inflammatory M1 or anti-inflammatory M2. This binary classification of macrophage phenotype was based on in vitro observations that macrophages treated with the type 1 T helper (Th1) cytokine, interferon gamma (IFNγ) or the Th2 cytokine, interleukin 4 (IL-4), exhibited distinct genetic expression patterns, termed M1 and M2, respectively (Liu et al., Reference Liu, Geng, Hou and Wu2021).
Generally, M1 macrophages are characterized by the upregulation of tumour necrosis factor (tnf), inducible nitric oxide synthase (iNOS) and IL-1β expression, which mediate anti-microbial innate immune responses (Varga et al., Reference Varga, Mounier, Horvath, Cuvellier, Dumont, Poliska, Ardjoune, Juban, Nagy and Chazaud2016). Production of IL-12 and IL-23 further supports the inflammatory state by promoting the differentiation and expansion of Th1 and Th17 cells, respectively (Liu et al., Reference Liu, Geng, Hou and Wu2021). In addition, M1 macrophages adopt a metabolic signature that favours glycolysis, thereby rapidly producing adenosine triphosphate (ATP) that supports pro-inflammatory signalling (Kieler et al., Reference Kieler, Hofmann and Schabbauer2021). In contrast, a typical M2 macrophage is associated with the resolution of inflammation and mediation of the healing process and is characterized by increased expression levels of different genetic markers, notably Arg1, Retnla, Ym1 and Ear11 (Murray et al., Reference Murray, Allen, Biswas, Fisher, Gilroy, Goerdt, Gordon, Hamilton, Ivashkiv and Lawrence2014). For M2 macrophages, oxidative phosphorylation is the predominant metabolic activity resulting in a delayed, but more prolific production of ATP, which is needed to support the specific functional demands of the M2 phenotype, as compared to the anti-microbial activities of M1 macrophages (Palsson-McDermott et al., Reference Palsson-Mcdermott, Curtis, Goel, Lauterbach, Sheedy, Gleeson, Van Den Bosch, Quinn, Domingo-Fernandez and Johnston2015; Xie et al., Reference Xie, Yu, Kang, Zhu, Yang, Zeng, Sun, Yang, Billiar and Wang2016). More recent studies of macrophage metabolism have revealed that while the anti-inflammatory activity of macrophages solely requires oxidative metabolism (Ip et al., Reference Ip, Hoshi, Shouval, Snapper and Medzhitov2017; Tao et al., Reference Tao, Zhang, Ling, Mccall and Liu2018), the expression of characteristic M2 genes induced by IL-4 simply requires a threshold of ATP, which can be reached via glycolysis or oxidative phosphorylation (Wang et al., Reference Wang, Zhang, Vuckovic, Jeon, Lerman, Folmes, Dzeja and Herrmann2018). These observations uncover a disconnect between the original, binary paradigm of macrophage phenotypes (characterized by a simple genetic signature), and the more recent functional characterizations of macrophage subsets according to metabolic preferences (Sanin et al., Reference Sanin, Ge, Marinkovic, Kabat, Castoldi, Caputa, Grzes, Curtis, Willenborg, Dichtl, Reinhardt, Dahl, Pearse, Eming, Gerbaulet, Roers, Murray and Pearce2021).
The need for a model for the activation of multiple macrophage phenotypes has become increasingly evident after the identification of numerous factors that drive the activation of macrophages into distinct phenotypes, which are characterized by distinct genetic and biological profiles (Colegio et al., Reference Colegio, Chu, Szabo, Chu, Rhebergen, Jairam, Cyrus, Brokowski, Eisenbarth and Phillips2014; Murray et al., Reference Murray, Allen, Biswas, Fisher, Gilroy, Goerdt, Gordon, Hamilton, Ivashkiv and Lawrence2014; Parisi et al., Reference Parisi, Gini, Baci, Tremolati, Fanuli, Bassani, Farronato, Bruno and Mortara2018). Thus, macrophage populations cannot always be appropriately assigned to either the M1 or the M2 phenotype (Helm et al., Reference Helm, Held-Feindt, Grage-Griebenow, Reiling, Ungefroren, Vogel, Krüger, Becker, Ebsen and Röcken2014; Ginhoux et al., Reference Ginhoux, Schultze, Murray, Ochando and Biswas2016). Accordingly, the notion that macrophage phenotypes lie along a spectrum between the polarized functional states of M1 (typically pro-inflammatory) and M2 (immune suppressive) is now widely accepted (Murray et al., Reference Murray, Allen, Biswas, Fisher, Gilroy, Goerdt, Gordon, Hamilton, Ivashkiv and Lawrence2014; Chen et al., Reference Chen, Liu, Gao, Shou and Chai2021).
This fluidity in polarization along a continuum of functional states is particularly evident for macrophages found within the tumour microenvironment (TME). These tumour-associated macrophages (TAMs) account for the largest fraction of the myeloid infiltrate in most human malignancies, including CCA (Zhou et al., Reference Zhou, Wang, Lu, Xu, Li, Jiang and Ma2021), and display a high degree of functional plasticity to adapt to the changes occurring during tumour progression and across different regions of the TME (Andrejeva and Rathmell, Reference Andrejeva and Rathmell2017; Mazzone et al., Reference Mazzone, Menga and Castegna2018). As a result, TAMs are phenotypically heterogeneous, comprising the spectrum extremes of M1 and M2 along with other, yet to be characterized, phenotypes (Biswas et al., Reference Biswas, Gangi, Paul, Schioppa, Saccani, Sironi, Bottazzi, Doni, Vincenzo, Pasqualini, Vago, Nebuloni, Mantovani and Sica2006; Lavin et al., Reference Lavin, Kobayashi, Leader, Amir, Elefant, Bigenwald, Remark, Sweeney, Becker, Levine, Meinhof, Chow, Kim-Shulze, Wolf, Medaglia, Li, Rytlewski, Emerson, Solovyov, Greenbaum, Sanders, Vignali, Beasley, Flores, Gnjatic, Pe'er, Rahman, Amit and Merad2017; Cheng et al., Reference Cheng, Bai, Shu, Ahmad and Shen2020). Like the macrophage subtypes identified during an inflammatory response to infection/injury or to the processes of tissue repair, this diversity in phenotypes is associated with distinct metabolic profiles. Switches in metabolic activity between glycolysis and oxidative phosphorylation direct the functional response within the tumour, with respect to rates of angiogenesis, tumour growth, metastasis and immune cell activation (Su et al., Reference Su, Wang, Bi, Ma, Liu, Yang, Qian and Yi2020). It has been proposed that the heterogeneity of macrophage phenotypes within the TME reflects a dual role of TAMs in tumours. The functional activity of M1/pro-inflammatory macrophages creates a mutagenic microenvironment that supports tumour initiation, while M2/wound-healing macrophages promote malignancy progression (Chanmee et al., Reference Chanmee, Ontong, Konno and Itano2014; Noy and Pollard, Reference Noy and Pollard2014; Salmaninejad et al., Reference Salmaninejad, Valilou, Soltani, Ahmadi, Abarghan, Rosengren and Sahebkar2019; Duan and Luo, Reference Duan and Luo2021).
Collectively, these observations demonstrate that conversions between macrophage phenotypes, which are largely induced by changes in metabolic flux, are a major determinant of macrophage function. In turn, this becomes a principal regulating factor, not only for the resolution of infection and removal of danger signals, but also in the initiation, progression and termination of several human diseases, including pathologies associated with helminth infection.
Macrophage activation and function during liver fluke infection
Macrophages dominate the immune responses to helminths. Due to their spectra of functional phenotypes, macrophages play a central and pleiotropic role in the host response to infection with helminths (reviewed by Cortes-Selva and Fairfax, Reference Cortes-Selva and Fairfax2021; Lechner et al., Reference Lechner, Bohnacker and Esser-von Bieren2021). It has been recently proposed that the functional roles of macrophages during helminth infection can be allocated to 3 categories (Coakley and Harris, Reference Coakley and Harris2020). Immediately after infection, an increase in tissue alarmins, released due to the migrating parasites, combined with the presence of helminth-secreted molecules, signals the presence of invading parasites that causes the macrophages to ‘react’ and produce a range of effector molecules that drive anti-helminth activities and recruit other immune cells to the infection site. Many parasites evade and/or modulate this initial host protective response, which supports their ability to survive and establish chronic infections. During these long-term infections, the feeding and migration behaviours of parasites cause extensive tissue damage, which is counteracted by the ‘repair’ activities of macrophages. These cells mediate the wound-healing response to prevent prolonged haemorrhaging and translocation of microbiota. However, this activity must be carefully balanced to prevent excessive tissue remodelling, which may cause fibrosis. Thus, macrophages enter a final ‘resolve’ phase during which they perform potent immune-regulatory activities.
The react, repair and resolve phases of macrophage activation are evident during infection with F. hepatica (Fig. 2). Immediately after infection, there is an influx of immune cells, of which macrophages are the most predominant, into the peritoneal cavity signalling the host's reaction to the migration of NEJs from the intestine (Walsh et al., Reference Walsh, Brady, Finlay, Boon and Mills2009; Pérez-Caballero et al., Reference Pérez-Caballero, Buffoni, Martínez-Moreno, Zafra, Molina-Hernández, Pérez and Martínez-Moreno2018a). However, despite the presence of migrating parasites in combination with perforations to the intestinal epithelium and the likely translocation of intestinal microbiota (Valero et al., Reference Valero, Navarro, Garcia-Bodelon, Marcilla, Morales, Hernandez, Mengual and Mas-Coma2006; Lalor et al., Reference Lalor, Cwiklinski, Calvani, Dorey, Hamon, Corrales, Dalton and De Marco Verissimo2021), there is no evidence of a pro-inflammatory M1 phenotype, which is characteristic of the mammalian protective immune response. Peritoneal macrophages isolated from infected animals (sheep and mice) show low expression levels of major histocompatibility complex-II, and no significant increase in the production of pro-inflammatory cytokines (TNF, IL-12, IFNγ) or anti-microbial effectors [iNOS, nitric oxide (NO)] (Ruiz-Campillo et al., Reference Ruiz-Campillo, Molina Hernandez, Escamilla, Stevenson, Perez, Martinez-Moreno, Donnelly, Dalton and Cwiklinski2017; Pérez-Caballero et al., Reference Pérez-Caballero, Javier Martínez-Moreno, Zafra, Molina-Hernández, Pacheco, Teresa Ruiz-Campillo, Escamilla, Pérez, Martínez-Moreno and Buffoni2018b). These observations led to the hypothesis that the Fasciola NEJs possess mechanisms to actively suppress the ability of the host to activate an immediate protective pro-inflammatory innate response. This would ensure the survival of the parasite and support its safe passage from the intestine, across the peritoneal cavity and on to the liver (Lalor et al., Reference Lalor, Cwiklinski, Calvani, Dorey, Hamon, Corrales, Dalton and De Marco Verissimo2021). Support for this premise is evident from animal studies, which have shown a correlation between the activation of the M1 phenotype (and associated Th1 type immune responses) and experimentally acquired or naturally occurring resistance to F. hepatica infection (Piedrafita et al., Reference Piedrafita, Parsons, Sandeman, Wood, Estuningsih, Partoutomo and Spithill2001; Golden et al., Reference Golden, Flynn, Read, Sekiya, Donnelly, Stack, Dalton and Mulcahy2010; Molina-Hernández et al., Reference Molina-Hernández, Mulcahy, Pérez, Martínez-Moreno, Donnelly, O'Neill, Dalton and Cwiklinski2015).
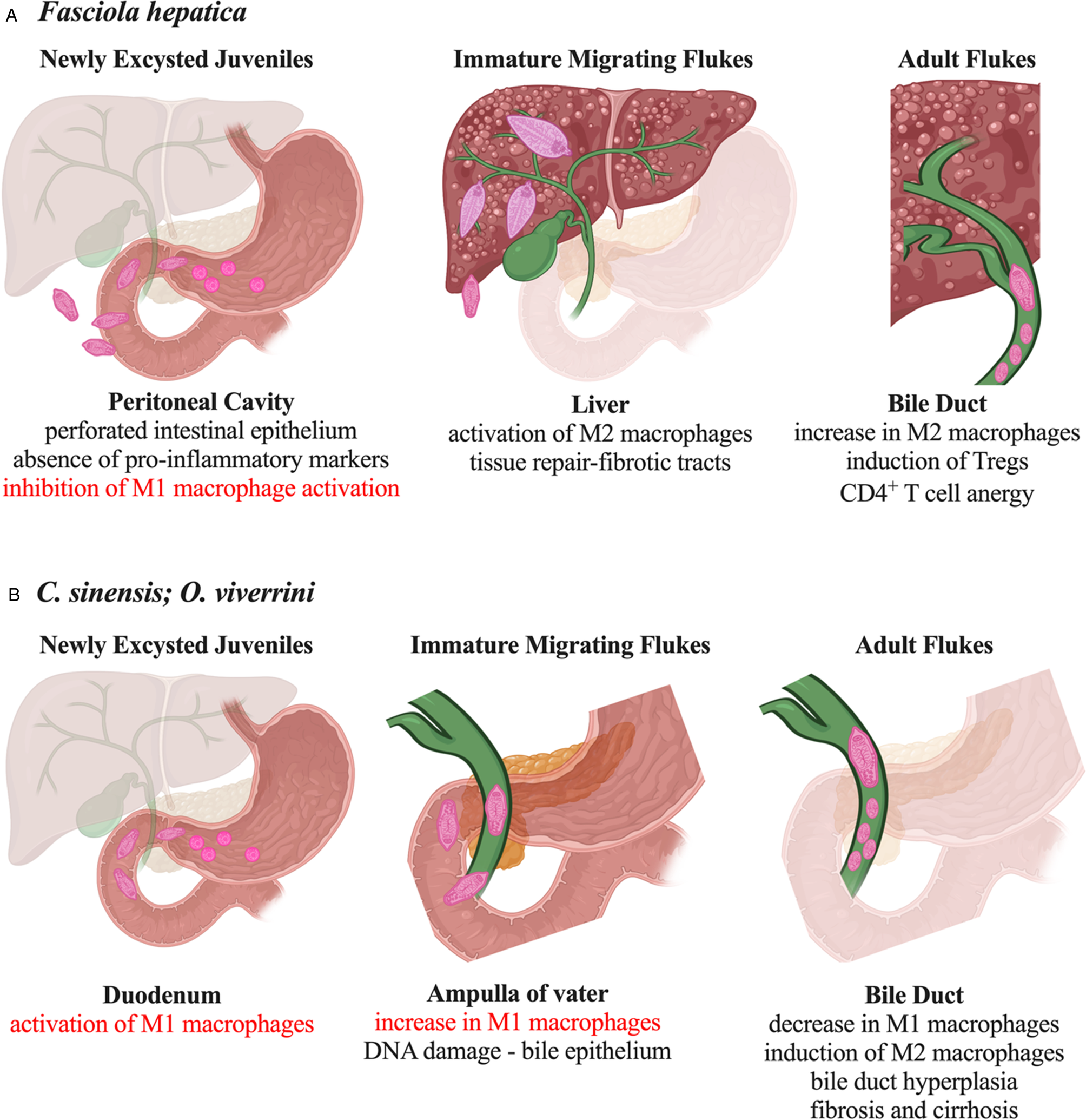
Fig. 2. Comparative activation of macrophages and pathological consequences of liver fluke infection. (A) During infection with F. hepatica the ability of macrophages to polarize towards a pro-inflammatory M1 phenotype is actively inhibited. In contrast, the activation of M2 macrophages, which occurs coincident with the parasite migration to the liver tissue, is actively promoted to mediate tissue repair and the regulation of effector T-cell responses. (B) Immediately after infection with either O. viverrini and C. sinensis, M1 macrophages are abundant and correlate with the presence of DNA damage within the bile duct epithelium. As infection progresses, this population of macrophages decreases, although are never totally absent, and M2 macrophages become the abundant phenotype coincident with the appearance of fibrosis and cirrhosis.
The penetration of the liver capsule by the NEJs is coincident with the appearance of an M2 phenotype of macrophage, as characterized by an increase in the expression levels of Arg1 and Ym1 (Donnelly et al., Reference Donnelly, O'Neill, Sekiya, Mulcahy and Dalton2005). The primary role of macrophages at this stage of infection is the promotion of tissue repair, rather than anti-helminth responses. A lack of programmed death-ligand 2+ (PD-L2+) M2 macrophages in Fasciola-infected mice had no effect on worm burden or size but led to exaggerated liver damage resulting in premature host death (Stempin et al., Reference Stempin, Motrán, Aoki, Falcón, Cerbán and Cervi2016). The immune-mediated repair of liver tissue is evidenced by the visible formation of fibrotic tracts and granulomas, both of which are characteristic liver pathologies associated with fasciolosis (Alvarez Rojas et al., Reference Alvarez Rojas, Ansell, Hall, Gasser, Young, Jex and Scheerlinck2015). During the later stages of liver migration and the final life stage in the bile duct, Fasciola infection stimulates the expansion of regulatory and anergic T-cell populations, which are important in reducing the severity of tissue pathology and sustaining the suppression of parasite-specific effector immune responses. The observation that PD-L2+ M2 macrophages induced by F. hepatica stimulated the differentiation of forkhead box p3+ T regulatory cells and anergy in CD4+ T cells ex vivo (Lund et al., Reference Lund, O'Brien, Hutchinson, Robinson, Simpson, Dalton and Donnelly2014; Guasconi et al., Reference Guasconi, Chiapello and Masih2015) suggests a critical role for macrophages in the initiation and perpetuation of these adaptive immune responses. However, additional in vivo studies with specific depletion of PD-L2+ macrophages will be required to definitively characterize their functional role in the promotion of host immune responses and the control of pathological outcomes.
Like the host response to F. hepatica, macrophages are the predominant immune cell population within the bile duct immediately after infection with O. viverrini and C. sinensis (Sripa et al., Reference Sripa, Jumnainsong, Tangkawattana and Haswell2018; Wang et al., Reference Wang, Bai, Jin, Tang, Yang, Sun, Li, Wang, Chang, Liu and Liu2021), and remain abundant for several months after infection (Bhamarapravati et al., Reference Bhamarapravati, Thammavit and Vajrasthira1978). However, in contrast to Fasciola, the expression levels of iNOS in macrophages is significantly increased as early as 3 days after infection in animal models (Wang et al., Reference Wang, Bai, Jin, Tang, Yang, Sun, Li, Wang, Chang, Liu and Liu2021) (Fig. 2). The proportion of these CD16/32+ iNOS+ M1 macrophages steadily increases in the liver and bile duct over the next 18 days of infection (Kim et al., Reference Kim, Kwak, Yi, Kim, Sohn and Yong2017; Wang et al., Reference Wang, Bai, Jin, Tang, Yang, Sun, Li, Wang, Chang, Liu and Liu2021). Then, coincident with the maturation of the parasite and subsequent egg production, this population of macrophages begins to decrease, but does not return to uninfected basal levels. At the same time, a population of CD206+Arg1+ macrophages emerges, indicating the presence of an M2 macrophage phenotype (Kim et al., Reference Kim, Kwak, Yi, Kim, Sohn and Yong2017; Wang et al., Reference Wang, Bai, Jin, Tang, Yang, Sun, Li, Wang, Chang, Liu and Liu2021). The wound-healing activity of macrophages, as described above, is correlated with their increased abundance during the fibrotic and cirrhotic stages of infection, when they become localized adjacent to the areas of collagen deposition within tissues (Bility and Sripa, Reference Bility and Sripa2014). Moreover, increasing the number of M2 macrophages in tissue exacerbated bile duct hyperplasia, and examination of tissue sections revealed an association between macrophages and cancer-associated fibroblasts (Thanee et al., Reference Thanee, Loilome, Techasen, Namwat, Boonmars, Pairojkul and Yongvanit2015; Yan et al., Reference Yan, Wu, Xu, Li, Zhou, Yang, Cheng, Liu, Dong, Koda, Zhang, Yu, Chen, Tang and Zheng2021). These observations highlight a significant role for M2 macrophages in the progression of CCA.
A comparison of macrophage phenotypes induced in response to liver fluke infections emphasizes that the induction of M1 macrophages is the primary difference in the innate immune response to O. viverrini and C. sinensis vs F. hepatica. With an understanding that pro-inflammatory M1-like macrophages have been suggested to drive the mutagenesis that supports tumour initiation, it is plausible that the induction of this phenotype of macrophage contributes to the carcinogenic effect of Opisthorchiidae infection, and a reason for the differential pathology between liver fluke infections. Supporting this proposition, it has been shown that the production of NO and the superoxide anion radical, O2.−, by M1 macrophages caused an increase in oxidative and nitrosative DNA damage, and accumulation of proliferating cell nuclear antigen, in the epithelium of bile ducts of hamsters infected with O. viverrini (Pinlaor et al., Reference Pinlaor, Ma, Hiraku, Yongvanit, Semba, Oikawa, Murata, Sripa, Sithithaworn and Kawanishi2004). Notably, repeat infections with O. viverrini resulted in an earlier and enhanced production of iNOS by macrophages and led to increased DNA damage (Pinlaor et al., Reference Pinlaor, Ma, Hiraku, Yongvanit, Semba, Oikawa, Murata, Sripa, Sithithaworn and Kawanishi2004). This finding corroborates evidence that the chronic inflammation due to reinfection with O. viverrini or C. sinensis is the primary risk factor for the development of CCA (IARC, 2012).
Activation of macrophages by fluke-derived molecules
The premise that parasite-derived factors interact with host macrophages to influence their functional activity is supported by analyses of tissue from animals infected with F. hepatica and O. viverrini. For both parasites, their antigens commonly co-localize with inflammatory cell infiltrates, notably macrophages, even at sites that are distant from the flukes (Sripa and Kaewkes, Reference Sripa and Kaewkes2000; Tliba et al., Reference Tliba, Sibille, Boulard and Chauvin2000). Exploring this hypothesis, many groups have used an ‘omics’ approach to characterize the proteins of the food-borne flukes within their excretory–secretory products, as these would be the most likely to interact with host immune cells and mediate the pathogenesis of infection (Ravidà et al., Reference Ravidà, Cwiklinski, Aldridge, Clarke, Thompson, Gerlach, Kilcoyne, Hokke, Dalton and O'Neill2016; Cwiklinski and Dalton, Reference Cwiklinski and Dalton2018; Suttiprapa et al., Reference Suttiprapa, Sotillo, Smout, Suyapoh, Chaiyadet, Tripathi, Laha and Loukas2018; Shi et al., Reference Shi, Yu, Liang, Huang, Ou, Wei, Wan, Yang, Zhang and Jiang2020).
Proteomics comparisons of the excretory–secretory products of the liver flukes reveal a slight variation in the proteome for each parasite, which likely reflects the different biological activities required to support their distinct migratory paths through the host tissue. For example, proteolytic enzymes were underrepresented among the secreted proteins of O. viverrini, as compared to those of F. hepatica (Mulvenna et al., Reference Mulvenna, Sripa, Brindley, Gorman, Jones, Colgrave, Jones, Nawaratna, Laha, Suttiprapa, Smout and Loukas2010). A more detailed examination of peptidase expression in the flukes revealed that while O. viverrini and C. sinensis produced high levels of cathepsin F (Kaewpitoon et al., Reference Kaewpitoon, Laha, Kaewkes, Yongvanit, Brindley, Loukas and Sripa2008; Pinlaor et al., Reference Pinlaor, Kaewpitoon, Laha, Sripa, Kaewkes, Morales, Mann, Parriott, Suttiprapa, Robinson, To, Dalton, Loukas and Brindley2009; Kang et al., Reference Kang, Bahk, Cho, Hong, Kim, Sohn and Na2010), F. hepatica secreted a variety of cathepsin L and cathepsin B enzymes with no cathepsin F detected (Cwiklinski et al., Reference Cwiklinski, Dalton, Dufresne, La Course, Williams, Hodgkinson and Paterson2015). This stark variation clearly relates to the different routes each parasite takes through host tissue. Fasciola requires the collagenolytic activities of the cathepsin L and B enzymes to disrupt the interstitial matrix to cross the intestinal wall and to subsequently penetrate the liver. However, this enzymatic activity is not required by O. viverrini or C. sinensis as they reach the bile ducts from the duodenum by simply ascending the hepatopancreatic duct (ampulla of Vater). However, this difference in migratory patterns may also impact the host immune responses. For example, cathepsin L enzymes secreted by F. hepatica selectively inhibit several pro-inflammatory signalling pathways in mammalian macrophages, which prevent the development of an M1 phenotype (Donnelly et al., Reference Donnelly, O'Neill, Stack, Robinson, Turnbull, Whitchurch and Dalton2010).
In fact, the secreted proteins of F. hepatica typically skew the phenotype of macrophages away from a pro-inflammatory M1 phenotype and/or towards a wound-healing/regulatory M2 phenotype. In contrast, the secreted proteins of O. viverrini and C. sinensis predominantly promote the development of pro-inflammatory M1 macrophages (Table 1). These reported differences in protein activity could be explained by differences in the production and purification methods used across different research groups. Traditional recombinant production using bacterial cells can result in the presence of residual bacterial lipopolysaccharide, which will contaminate the recombinant protein and skew the macrophage response towards an M1 phenotype. However, as this differential activation of macrophages by fluke-derived proteins is consistent across multiple research groups, and with both native secretions and recombinant/synthetic proteins it seems plausible that these differences in biological activities reflect a specific functional adaptation for each parasite.
Table 1. Impact of fluke-derived proteins on macrophage activation in vitro

LPS, lipopolysaccharide; NF-κB, nuclear factor kappa B; TNF, tumour necrosis factor; IL, interleukin; Arg-1, arginase-1; Ym1, chitinase-like protein 3; Fizz1, resistin-like molecule alpha 1; ERK, extracellular signal-regulated kinase; JNK, Jun N-terminal kinase; TLR, Toll-like receptor; TRIF, TIR-domain-containing adapter-inducing interferon-β; SMAD, transcription factor small mothers against decapentaplegic; PD-L1, programmed death-ligand 1; NO, nitric oxide; v-ATPase, vacuolar-type ATPase; NLRP3, NOD-like receptor protein 3; PKA, protein kinase A; MAPK, mitogen-activated protein kinase; SOCS1, suppressor of cytokine signalling 1; Clec7a, C-type lectin domain containing 7A; iNOS, inducible nitric oxide synthase; COX-2, cyclooxygenase-2.
This discrepancy in the immune modulating activity of the parasite-derived proteins may be attributed to the initiation of distinct protective host responses associated with different anatomical locations. As described previously, the induction of anti-microbial pro-inflammatory M1 macrophages in the peritoneal cavity is characteristic of a protective immune response against F. hepatica (Piedrafita et al., Reference Piedrafita, Parsons, Sandeman, Wood, Estuningsih, Partoutomo and Spithill2001). In contrast, the expulsion of intestinal helminths is mediated by a Th2 immune response (Perrigoue et al., Reference Perrigoue, Marshall and Artis2008). Rather than utilizing direct anti-microbial mechanisms, this immune phenotype drives an accelerated epithelial cell turnover, which effectively dislodges the parasites resulting in their clearance (Cliffe et al., Reference Cliffe, Humphreys, Lane, Potten, Booth and Grencis2005). Importantly, the expulsion of worms from the intestinal lumen is mediated by intestinal contractility, which is regulated by M2 macrophages (Zhao et al., Reference Zhao, Urban, Anthony, Sun, Stiltz, van Rooijen, Wynn, Gause and Shea-Donohue2008). Of relevance to the liver flukes, susceptibility to infection with the intestinal fluke Echinostoma caproni is linked to the induction of a Th1 type immune response, which slows epithelial cell turnover and promotes tissue hyperplasia. This allows parasites to more stably attach to host cells, which favours the establishment of chronic infections (Cortéz et al., Reference Cortés, Muñoz-Antoli, Martín-Grau, Esteban, Grencis and Toledo2015). Putatively, a similar protective mechanism is initiated in the bile duct in response to infection with Clonorchis and Opisthorchis. This would explain the broad capacity for their secreted proteins to drive M1/Th1 type immune responses.
An alternate/additional benefit of inducing M1 macrophages may be to balance the development of M2 macrophage populations that mediate wound healing in the bile duct, as excessive fibrotic scar tissue would prevent the parasites effectively accessing the epithelium for feeding. This notion is supported not only by the evident induction of M1 macrophages after infection, but also by the observation that this population is sustained, albeit at a reduced proportion, throughout the course of infection (Kim et al., Reference Kim, Kwak, Yi, Kim, Sohn and Yong2017; Wang et al., Reference Wang, Bai, Jin, Tang, Yang, Sun, Li, Wang, Chang, Liu and Liu2021). Furthermore, the manipulation of innate immune signalling pathways in mice infected with C. sinensis, which resulted in a reduction of M1 macrophages and an increase in M2 macrophages, was associated with aggravated peribiliary fibrosis (Yan et al., Reference Yan, Wu, Xu, Li, Zhou, Yang, Cheng, Liu, Dong, Koda, Zhang, Yu, Chen, Tang and Zheng2021). This functional role for M1 macrophages in the context of a parasite infection is further strengthened by studies of mice infected with the blood fluke, Schistosoma mansoni. For this helminth, the manipulation of cytokines to create a bias towards the development of M1 macrophages resulted in reduced collagen deposition and smaller granuloma formation around parasite eggs deposited in the liver (Wynn et al., Reference Wynn, Cheever, Jankovic, Poindexter, Caspar, Lewis and Sher1995; Hesse et al., Reference Hesse, Modolell, La Flamme, Schito, Fuentes, Cheever, Pearce and Wynn2001). Furthermore, the resulting mixed immune response, with a slight predominance of Th1/M1 cells, was most effective as it afforded sufficient protection from tissue damage caused by the parasite while simultaneously minimizing fibrosis (Barron and Wynn, Reference Barron and Wynn2011).
Consideration of the pathogenesis of S. mansoni is of relevance when comparing the biological activity of the helminth defence molecules (HDMs), which are peptides secreted by all trematode parasites (Robinson et al., Reference Robinson, Donnelly, Hutchinson, To, Taylor, Norton, Perugini and Dalton2011). Remarkably, the HDM secreted by F. hepatica (FhHDM) inhibits the development of M1 macrophages, but despite being classified in the same family of peptides, the HDM secreted by C. sinensis (CsHDM) induces an M1 phenotype (Table 1; Lund et al., 2016; Alvarado et al., Reference Alvarado, To, Lund, Pinar, Mansell, Robinson, O'Brien, Dalton and Donnelly2017; Kang et al., Reference Kang, Yoo, Lê, Lee, Sohn and Na2020). Analysis of the phylogenetic relationship of the HDM peptide family shows that while CsHDM is on the same branch as FhHDM, it is evolutionarily closer to another branch of HDM peptides, the Sm16 peptides, found exclusively in Schistosoma (Shiels et al., Reference Shiels, Cwiklinski, Alvarado, Thivierge, Cotton, Gonzales Santana, To, Donnelly, Taggart, Weldon and Dalton2020). Characterization of the Sm16 from S. mansoni showed that it was primarily expressed by cercariae and eggs, and like the CsHDM induces a pro-inflammatory response in macrophages. These findings thus suggest a functional role in the management of wound-healing responses associated with the predominance of M2 macrophages.
Concluding remarks
Helminths have evolved multiple mechanisms to modulate the immune response of their mammalian hosts to support the establishment of chronic infections, and to ensure sustained reproductive success. The development of immune modulatory mechanisms is informed by different phenomena within the host, the most evident of which is the migratory pathways of each parasite. The analyses of macrophage phenotypes presented here highlight a remarkable difference in host immune responses to carcinogenic flukes, as compared to non-carcinogenic F. hepatica. Evidence supports the likelihood that this phenomenon reflects the specific requirements associated with each parasite's distinctive migration patterns and final anatomical location within the mammalian host.
The influence that anatomical location has on the immune-mediated pathological outcome to infection with helminths can be extended to infection with Schistosoma. While the hepatic schistosomes of humans, Schistosoma japonicum and S. mansoni, do not cause cancer, Schistosoma haematobium, a parasite that resides in the bladder, is carcinogenic, with infection associated to the development of squamous cell carcinoma (Mostafa et al., Reference Mostafa, Sheweita and O'Connor1999). A comparison of the immune and fibrotic response to the deposition of S. haematobium eggs in the bladder, compared to the response of S. mansoni eggs in the liver revealed distinct collagen-remodelling pathways associated with each anatomical location (Ray et al., Reference Ray, Nelson, Fu, Patel, Gong, Odegaard and Hsieh2012). This likely reflects the specific adaptation of host immune responses to infection within the bladder, which has been characterized as a highly polarized Th2 type immune response that directs the rapid re-epithelialization, and thus prioritizes the regeneration of tissue at the expense of preventing re-infections (Wu et al., Reference Wu, Hayes, Phoenix, Macias, Miao, Choi, Hughes, Todd Purves, Lee Reinhardt and Abraham2020). While there was no specific analysis of macrophage populations, analysis of gene expression within the tissues revealed that like the carcinogenic liver flukes, the immune response for S. haematobium reflected a greater mix of type 1 and type 2 immune responses, compared to the predominance of M2 macrophages and a Th2 type immune response associated with S. mansoni infection (Ray et al., Reference Ray, Nelson, Fu, Patel, Gong, Odegaard and Hsieh2012).
Therefore, while multiple factors likely modulate susceptibility to fluke-induced cancer, the evidence from both flukes and schistosome infections would support a scenario in which the differential regulation of an M1/pro-inflammatory type of macrophage is a major contributory factor in the pathological outcome to infection with liver flukes. However, while the studies reviewed here classified macrophage phenotypes as M1 or M2, in the most part these characterizations were based on the expression of only a small number of characteristic genes (Arg1, Ym1, PD-L2) or cytokines (TNF, IL-6, IL-10). As macrophages are more likely to be present as multiple functional variants throughout the different stages of parasite infection, further longitudinal and more detailed characterization of the genotype and functional phenotype of these macrophages will be critical to fully understand how the biological activities of macrophages modulate the host responses to infection with liver flukes.
Author contributions
S. L. Q. and S. D. conceived and planned the review. S. L. Q. conducted the literature search, designed and wrote the first draft of the manuscript. S. D. and B. O. B. contributed to the writing and editing of the final manuscript.
Financial support
S. L. Q. is supported by an Australian Government Research Training Program Scholarship.
Conflict of interest
The authors declare there are no conflicts of interest.