Introduction
Nematodes of the genus Physaloptera Rudolphi, 1819 are globally distributed and more than 100 species are known (Pereira et al., Reference Pereira, Alves, Rocha, Lima and Luque2014; São Luiz et al., Reference São Luiz, Simões, Torres, Barbosa, Santos, Giese, Rocha and Maldonado2015). They infect amphibians, reptiles, birds and mammals (Ortlepp, Reference Ortlepp1922; Chabaud, Reference Chabaud, Anderson, Chabaud and Wilmont1975; Anderson et al., Reference Anderson, Chabaud and Willmott2009). Their life cycle involves insects, including beetles, cockroaches and crickets, as intermediate hosts (Hobmaier, Reference Hobmaier1941; Goldberg & Bursey, Reference Goldberg and Bursey2002). The adult worms firmly attach to the stomach wall, where they feed on the mucosa and cause gastritis, enteritis and excessive mucous secretion by the host (Panti-May et al., Reference Panti-May, Hernández-Betancourt, Rodríguez-Vivas and Robles2015).
The genus Proechimys of caviomorph rodents belongs to the family Echimyidae, which stands out for its extraordinary ecomorphological diversity (Galewski et al., Reference Galewski, Mauvrey, Leite, Patton and Douzery2005). Proechimys is the most speciose and geographically widespread genus of echimyids (Patton & Leite, Reference Patton, Leite, Patton, Pardiñas and D’Elía2015). These species have terrestrial, nocturnal and solitary habits, although they are often the most abundant non-volant mammals in lowland Neotropical forests (Emmons & Feer, Reference Emmons and Feer1997). Proechimys gardneri da Silva, 1998 inhabits the Amazon rainforest and is distributed from its eastern limit at the Madeira and Solimões Rivers to its northern limit in a small stretch of the Amazon River (Eler et al., Reference Eler, Da Silva, Silva and Feldberg2012).
Amazonia is the largest and most diverse of the tropical forest wilderness areas (Silva et al., Reference Silva, Rylands and Fonseca2005). The Amazon biome is situated in the north of South America, distributed in parts of nine countries: Brazil, Venezuela, Colombia, Peru, Bolivia, Ecuador, Suriname, Guyana and French Guiana (Portal Amazônia, 2018). Sixty per cent of the Amazon rainforest lies in Brazilian territory (Migueis, Reference Migueis2001). However, there are few studies in this region, especially regarding helminth biodiversity.
In this study, we describe a new species of Physaloptera found in the echimyid rodent P. gardneri collected in the Brazilian Amazon rainforest in the state of Acre. Additionally, we describe molecular phylogenetic analyses of the new species and representatives of Physaloptera and other physalopteroid genera in order to determine its relationships within the genus.
Material and methods
Study area
Nematodes were collected from the stomach of five naturally infected P. gardneri specimens in the municipality of Xapuri (10°49′S, 68°21′W), Acre, Brazil. Collections were carried out between March 2014 and December 2015, using Tomahawk® (Tomahawk Live Trap, USA)(model 201; 16 × 5 × 5 inches or 40.6 × 12.7 × 12.7 cm) and Sherman® (Sherman Live Trap, USA) (model XLK; 3 × 3.75 × 12 inches or 7.6 × 9.5 × 30.5 cm) live traps. This study was approved by the ethics committee of the Oswaldo Cruz Foundation/FIOCRUZ (CEUA number LW-39/14). Capture and sampling of small mammals were authorized by the Chico Mendes Institute for Conservation of Biodiversity (ICMBIO/SISBIO, license number 13373). For euthanasia, the animals were heavily anaesthetized with an intramuscular injection of thiopental sodium, followed by intracardiac administration of potassium chloride, under the supervision of a licensed veterinarian (Gannon & Sikes, Reference Gannon and Sikes2011). Parasites were washed in 0.9% NaCl solution and fixed in hot alcohol-formalin-acetic acid (AFA) (2% glacial acetic acid, 3% formaldehyde and 95% ethanol) for morphological identification, or alternatively preserved in 70% ethanol for DNA isolation.
The nematodes were clarified in 80% phenol and mounted on temporary slides. Morphological analyses were conducted using an Olympus BX-51 light microscope (Tokyo, Japan) and images were captured using an Olympus DP-12 digital camera (Tokyo, Japan). Drawings were made using a drawing tube attached to a Nikon Y-IDT light microscope (Tokyo, Japan). Measurements were taken in millimetres (means are followed by the range in parentheses) from eight male and ten female adult specimens. Nematode identification was according to Ortlepp (Reference Ortlepp1922), Vicente et al. (Reference Vicente, Rodrigues, Gomes and Pinto1997) and Anderson et al. (Reference Anderson, Chabaud and Willmott2009). Holotype, allotype and paratypes were deposited in the Helminthological Collection of the Oswaldo Cruz Institute, Rio de Janeiro (CHIOC).
For scanning electron microscopy analysis, nematodes were washed in 0.1 M Na-cacodylate buffer, pH 7.2, post-fixed in 1% OsO4 and 0.8% K3Fe (CN)6, dehydrated in graded ethanol (30–100%) for 2 h and dried by the critical point method with CO2 (CPD 030, Balzers, Switzerland). The samples were mounted on aluminium stubs, coated with a 20 nm layer of gold and examined with a Jeol JSM 6390LV scanning electron microscope (operating at 15 kV) (JEOL, Akishima, Tokyo, Japan) at the Rudolf Barth Electron Microscopy Platform of Oswaldo Cruz Institute, RJ.
DNA isolation, polymerase chain reaction (PCR) and sequencing
Genomic DNA samples were isolated from two individual nematodes using the Qiagen QIAamp DNA Mini Kit, according to the manufacturer's protocol. DNA amplification by PCR was conducted using designed primers PHYSA_F (5′ GCGAACGGCTCATTATAA 3′) and PHYSA_R (5′ AATTTCACCTCTCAGCA 3′) for a partial region of the nuclear small subunit ribosomal RNA (18S) gene. For the barcode region of the mitochondrial cytochrome c oxidase subunit I (MT-CO1) gene, we used the primer cocktail described by Prosser et al. (Reference Prosser, Velarde-Aguilar, León-Règagnon and Hebert2013). Reactions were carried out in a total volume of 50 µl, containing 33.8 µl of water, 5 µl of 10X buffer (200 mm Tris-HCl (pH 8.4), 500 mm KCl), 3 µl of 50 mm MgCl2, 5 µl of 10 mm dNTP mix, 1 µl of each 10 µm primer, 0.2 µl of 5 U/μl Taq DNA polymerase and 1 µl of DNA sample. Thermal cycling conditions were 95°C for 2 min, followed by 39 cycles at 95°C for 30 s, 54°C for 30 s, 72°C for 1 min and a final extension at 72°C for 1 min. The resulting amplicons were visualized on 1% agarose gel, stained with GelRed, under ultraviolet light after electrophoresis. Successfully amplified products were purified using the QIAquick PCR Purification Kit (Qiagen).
Sequencing reactions and readings were performed at the subunit RPT01A – DNA Sequencing of the Genomic Platform, Technological Platforms Network, Fiocruz (PDTIS/FIOCRUZ), using the Big Dye Terminator v3.1 Cycle Sequencing Kit (Applied Biosystems, USA), on both strands, with the primers mentioned above. Fragments were assembled into contigs and edited for errors and ambiguities with the Geneious 9.1.8 suite (https://www.geneious.com) (Kearse et al., Reference Kearse, Moir, Wilson, Stones-Havas, Cheung, Sturrock, Buxton, Cooper, Markowitz, Duran, Thierer, Ashton, Meintjes and Drummond2012), resulting in consensus sequences. All sequences obtained in this study were deposited in the GenBank database under the accession numbers indicated in table 1.
Table 1. Specimens included in the molecular analyses associated with their GenBank accession numbers for the MT-CO1 and 18S genes, hosts and locality.

Phylogenetic tree reconstructions
We conducted phylogenetic analyses using two different datasets, one for the 18S gene and one for the MT-CO1 gene. We sequenced 11 nematode specimens for the partial 18S and MT-CO1 genes. Datasets consisted, along with our specimens, of sequences of other physalopterids retrieved from the GenBank. As outgroups, sequences of Gnathostoma turgidum were added to both matrices (table 1). The resulting 18S dataset had 24 taxa, whereas the MT-CO1 dataset had 20 taxa.
Alignments for the 18S gene sequences were produced using the SILVA (high-quality ribosomal RNA databases) online Incremental Aligner (SINA v1.2.11) (Pruesse et al., Reference Pruesse, Peplies and Glöckner2012), whereas alignments for the MT-CO1 gene sequences were produced using the Translator X server (Abascal et al., Reference Abascal, Zardoya and Telford2010). Initial alignment for Translator X was provided using MUSCLE (Edgard, Reference Edgard2004). Resulting alignments were edited and trimmed of regions with poor overlap using the Mesquite Version 3.51 (Maddison & Maddison, Reference Maddison and Maddison2018) software package. Uncorrected pairwise p-distances were calculated for each matrix using the program PAUP*, version 4.0a165 (Swofford, Reference Swofford2002).
Phylogenetic reconstructions using maximum parsimony (MP), as optimality criterion, were carried out using PAUP*. The parsimony settings were: collapse branches if the minimum length is zero (‘amb-’) and treatment of gaps as ‘new state’ (fifth base); all other parsimony and heuristic search settings were defaults. Branch supports were assessed by nonparametric bootstrap percentages (MP-BS) after 10,000 pseudo replications.
Phylogenetic reconstructions using maximum likelihood (ML), as optimality criterion, were carried out using the program FastTree 2.1 (Price et al., Reference Price, Dehal and Arkin2010) using the general time reversible (GTR) + G model to optimize the ML. Branch supports were assessed by local support values using the Shimodaira–Hasegawa test (ML-SH) with 1000 resamples. The best-fit nucleotide evolutionary model was calculated under the corrected Akaike information criterion using the Automated Model Selection on PAUP*.
Bayesian phylogenetic inferences (BIs) were carried out using MrBayes version 3.2.6 (Ronquist et al., Reference Ronquist, Teslenko, Mark, Ayres, Darling, Höhna, Larget, Liu, Suchard and Huelsenbeck2012) in XSEDE using the CIPRES Science Gateway (Miller et al., Reference Miller, Pfeiffer and Schwartz2010). To account for different evolutionary processes at each codon position of the MT-CO1 gene, Bayesian analyses were performed using a distinct GTR + I + G model per codon position, with unlinking of base frequencies, GTR and parameters. The 18S gene Bayesian analyses were performed using a single GTR + I + G model. Markov chain Monte Carlo samplings for each matrix were performed for 10,000,000 generations with four simultaneous chains in two runs. We assessed branch supports in Bayesian trees by Bayesian posterior probabilities (BPPs), calculated from trees that were sampled every 100 generations, after the removal of a burn-in fraction of 25%. We assessed the robustness of sampling using the Tracer v1.7.1 program (Rambaut et al., Reference Rambaut, Drummond, Xie, Baele and Suchard2018) to calculate the effective sample sizes (ESSs) of parameters. After removal of a burn-in fraction of 25%, values above 100 effectively independent samples were considered sufficiently sampled.
The substitution saturation in each dataset was assessed as proposed by Xia et al. (Reference Xia, Xie, Salemi, Chen and Wang2003) and Xia & Lemey (Reference Xia, Lemey, Lemey, Salemi and Vandamme2009), using the program DAMBE, version 7.0.35 (Xia, Reference Xia2018). Each codon position was also tested separately in the MT-CO1 dataset. We also tested each dataset for the presence of a phylogenetic signal using the g1 statistic (Sokal & Rohlf, Reference Sokal and Rohlf1995), examining 10,000,000 randomly generated topologies and the permutation tail probability (PTP) test (Faith & Cranston, Reference Faith and Cranston1991), with 10,000 permutations. Both tests were implemented using PAUP*.
Results
Systematics
Superfamily: Physalopteroidea Sobole, 1949
Family: Physalopteridae Leiper, 1908
Subfamily: Physalopterinae Railliet, 1893
Genus: Physaloptera Rudolphi, 1819
Physaloptera amazonica n. sp.
Description
Body robust, filiform with fine transversal cuticular striations along the body. Sexual dimorphism present, females more robust than males. Anterior end forming cephalic collarette (figs 1a and 2a). Oral opening with two semi-circular pseudolabia presenting an external lateral tooth with a triangular shape, and three internal lateral teeth forming a tripartite structure on each side (fig. 3a, b). Apical region presenting two pairs of papillae, porous area and one pair of amphids (fig. 3a). Three well-delimited porous areas present on each lip (fig. 3a). Oesophagus divided into anterior muscular and posterior glandular parts (fig. 1a). Nerve ring surrounding the middle portion of muscular oesophagus. Excretory pore located in the first third of the body (fig. 1a).
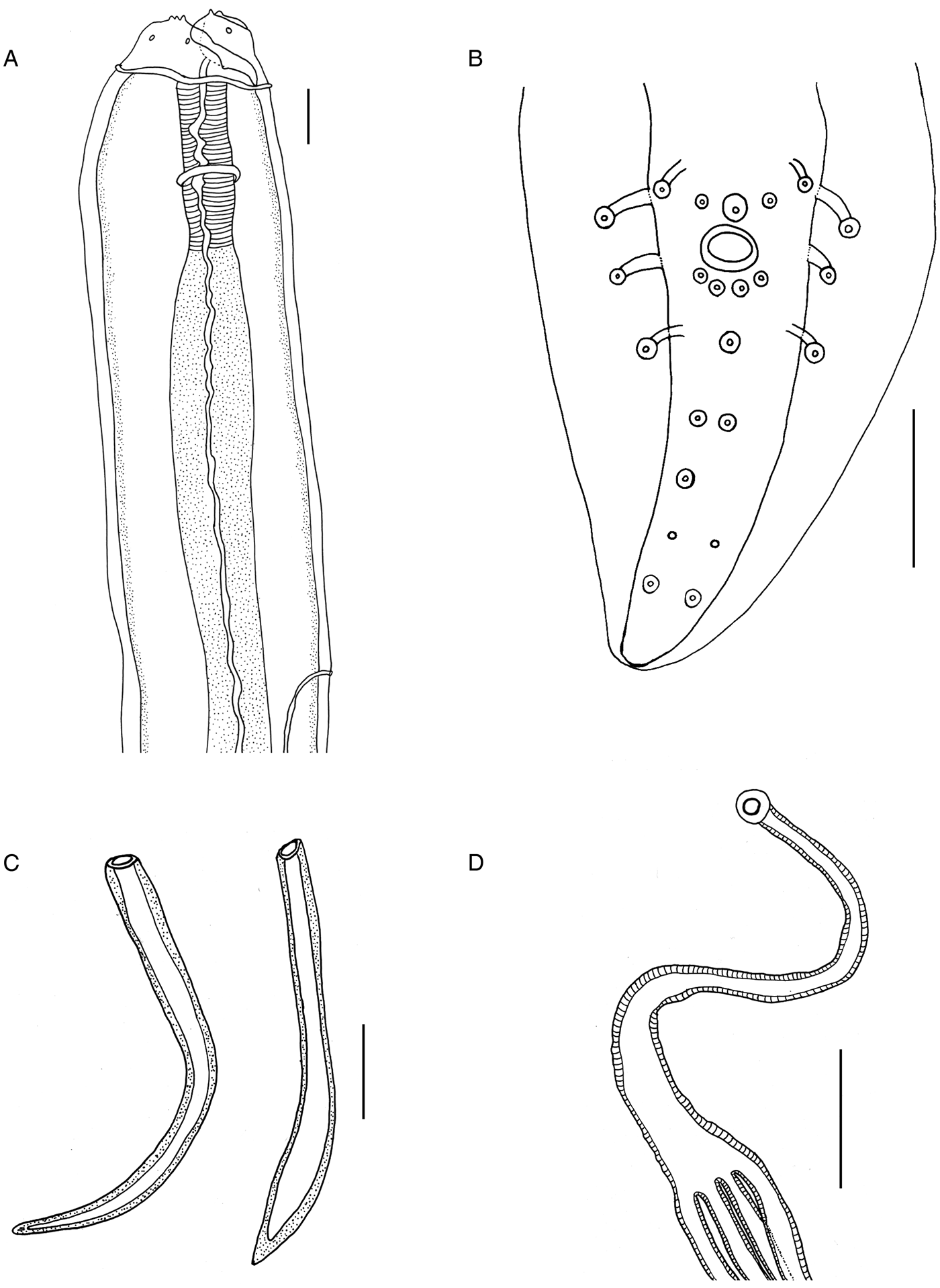
Fig. 1. Physaloptera amazonica n. sp. (A) Male, lateral view, anterior end showing tripartite tooth, cephalic papillae, collarette, muscular and glandular oesophagus, nerve ring and excretory pore. (B) Male, ventral view, posterior end with four pairs of pedunculated papillae and 13 sessile papillae. (C) Left and right spicules. (D) Uterus with four uterine branches. Scale bars: (A, C) 100 µm; (B, D) 50 µm.
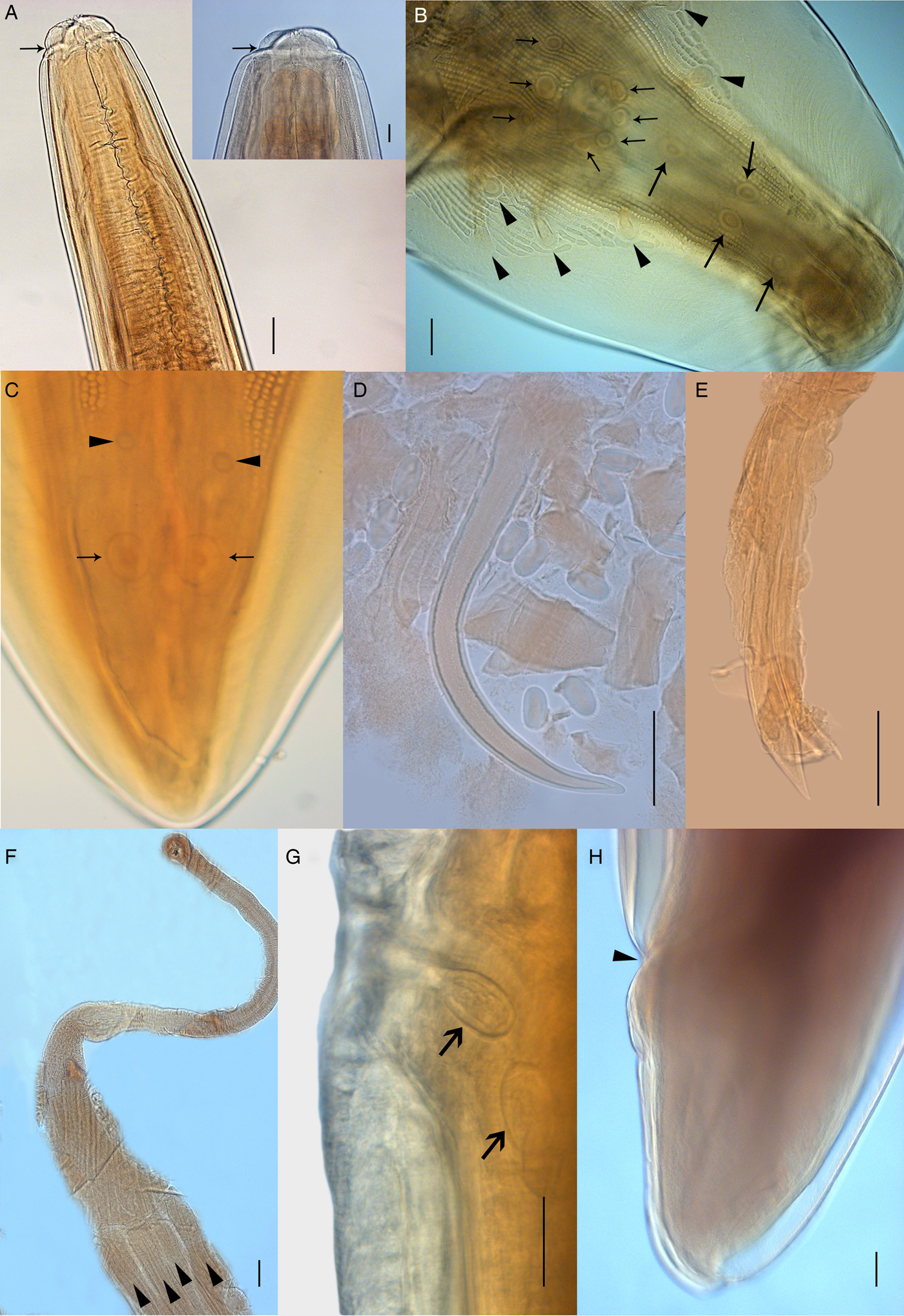
Fig. 2. Light microscopy of Physaloptera amazonica n. sp. (A) Male, ventral view, anterior end showing cephalic collarette and nerve ring (arrow). Detail showing tripartite tooth (scale bar: 50 µm). (B) Male, ventral view, posterior end showing pedunculated papillae (arrowheads) and sessile papillae (arrows). (C) Male, ventral view, posterior end showing phasmids (arrowheads) and pair of papillae (arrows). (D) Right spicule. (E) Left spicule. (F) Uterus with four uterine branches (arrowheads). (G) Vulva opening showing eggs, lateral view. (H) Female, lateral view, posterior end showing anus. Scale bars: (A, F, H) 100 µm; (B, C, D, E, G) 50 µm.

Fig. 3. Scanning electron microscopy of Physaloptera amazonica n. sp. (A) Female, apical view, anterior end showing pseudolabia with cephalic papillae (arrowheads), tripartite and small teeth, amphid (arrows), porous areas (*). (B) External lateral tooth and internal lateral tripartite teeth. (C) Female, ventral view, posterior end, showing the anal opening (arrow) and phasmids (arrowhead). (D) Transversal section showing uterus with eggs (arrowhead) and larvae at first stage (arrow). Scale bars: (A) 50 µm; (B) 10 µm; (C) 200 µm; (D) 20 µm.
Males. Based on holotype and seven paratypes. Total body length 27.86 (22.80–31.20); width at mid-body 1.26 (0.89–1.70). Muscular and glandular oesophagus 0.56 (0.50–0.69) and 2.85 (1.91–4.43) long, respectively. Nerve ring and excretory pore 0.62 (0.32–0.71) and 1.03(0.86–1.52) from the anterior end, respectively (figs 1a and 2a). Posterior end ventrally curved, with caudal alae ornate with irregular longitudinal ridges. Twenty-one caudal papillae: four pairs of pedunculated subventral papillae; one pair of subventral, precloacal, sessile papillae and bigger, ventral, median unpaired one; two pairs of sessile papillae immediately posterior to cloacal opening and three pairs of postcloacal papillae: first and second pairs asymmetrically displaced, left one anterior to right one. Third pair of postcloacal papillae equidistant near the caudal end. Presence of a pair of phasmids between the second and third pairs of postcloacal papillae (figs 1b and 2b, c). Spicules sub-equal and different in shape, right one curved without dilatation, 0.45 (0.40–0.50) long (figs 1c and 2d), and left one lanceolate at the final third 0.51 (0.42–0.63) long (figs 1c and 2e), representing 1.58–1.73% and 1.82–2.32% of total body length (SpL/BL), respectively.
Females. Based on allotype and nine paratypes. Total body length 37.71 (22.50–63.00) and width at mid-body 1.55 (0.87–3.00). Muscular and glandular oesophagus 0.67 (0.44–0.91) and 3.34 (2.21–4.02), respectively. Nerve ring and excretory pore 0.35 (0.11–0.43) and 1.66 (1.14–12.70) from anterior end, respectively. Uterus with four uterine branches (figs 1d and 2f). Vulva opening 13.84 (6.11–23.62) from the anterior end (fig. 2g). Slit-like anal opening 0.92 (0.51–1.22) from the posterior end (figs 2h and 3c). Tail conical. Eggs elliptical 0.05 (0.05) long and 0.03 (0.02–0.03) wide with a thick and hyaline wall (fig. 2g).
Taxonomic summary
Type host. Proechimys gardneri (da Silva, 1998) (Rodentia: Echimyidae).
Site of infection. Stomach.
Type locality. Municipality of Xapuri, state of Acre, Brazil (10°49′S, 68°21′W).
Prevalence. 15.6% (five rodents infected/32 rodents collected).
Mean intensity. 9 (1–19 per host).
Specimens deposited. Holotype: CHIOC 38715 (male); allotype: CHIOC 38720a (female); paratypes: CHIOC 38720b (one male and one female). All specimens were in wet materials.
Etymology. The specific epithet name refers to the biome where the hosts were collected.
Molecular and phylogenetic analyses
The 18S gene matrix had 22 taxa and 820 characters, of which 681 were constant, 79 were parsimony-uninformative variable and 60 were parsimony-informative variable (supplementary file S1). The MT-CO1 gene matrix had 20 taxa and 708 characters, of which 344 were constant, 122 were parsimony-uninformative variable and 242 were parsimony-informative variable (supplementary file S2). Both the PTP test and the g1 statistic indicated a strong phylogenetic signal in all datasets (supplementary file S3). Xia's test (Xia, Reference Xia2018) provided evidence of substantial saturation only at the third codon positions in the MT-CO1 dataset (supplementary file S4).
MP heuristic searches resulted in two most-parsimonious trees for the 18S matrix (scores = 202 steps; consistency indexes = 0.8020; supplementary file S1) and a single most-parsimonious tree for the MT-CO1 matrix (score = 960 steps; consistency index = 0.5687; supplementary file S2).
As the best-fit model for the 18S matrix, PAUP* selected GTR + I + G, with estimated frequencies, rates, Gamma (0.7139) and invariable sites (0.6558). The best log-likelihood ML-tree score for 18S was −2055.797 (supplementary file S5). As the best-fit model for the MT-CO1 matrix, PAUP* selected GTR + I + G, with estimated frequencies, rates, Gamma (0.3285) and invariable sites (0.3096). The best log-likelihood ML-tree score for MT-CO1 was −4626.594 (supplementary file S6).
For the BIs, at the 18S matrix, the mean estimated marginal likelihood was −2091.5880 and the median was −2091.2380 (supplementary file S7); at the MT-CO1 matrix, the mean estimated marginal likelihood was −4174.9832 and the median was −4174.6310 (supplementary file S8); ESSs for all parameters were above 100 effectively independent samples and much larger for most parameters, demonstrating the robustness of our sampling for both matrices (supplementary files S7 and S8).
The 18S matrix had pairwise interspecific genetic p-distances (supplementary file S9) ranging from zero between Physaloptera bispiculata and Physaloptera sp. from Costa Rica, and between P. mirandai and P. galvaoi to 10.9% between G. turgidum and P. galvaoi. Intraspecific genetic distances in the 18S matrix ranged from zero within P. mirandai and Turgida turgida to 0.2% within Heliconema longissimum. Distances of P. amazonica n. sp. ranged from 0.1% (P. mirandai and P. galvaoi) to 0.2% (Physaloptera sp. from Costa Rica), 0.3% (P. bispiculata), 0.5–0.6% (T. turgida), 0.9% (Physaloptera sp., from China), 1.0% (P. rara, Physaloptera sp. from USA and T. torresi), 1.8% (P. apivori), 1.9% (P. alata), 2.1% (P. thalacomys), 3.0% (Physalopteroides sp.), 3.8–3.9% (H. longissimum), 3.9% (P. obtusus), 4.4% (Proleptus sp.) and 10.4% (G. turgidum).
The MT-CO1 matrix had pairwise interspecific genetic p-distances (supplementary file S10) ranging from 3.4% between P. bispiculata and P. galvaoi to 35.6% between G. turgidum and P. obtusus. Intraspecific genetic distances in the MT-CO1 matrix ranged from zero within P. mirandai, Physaloptera sp. from Mexico and T. turgida to 9.3% within P. mirandai. Distances of P. amazonica n. sp. ranged from 9.7% (P. galvaoi) to 9.9% (P. bispiculata), 13.0% (P. retusa), 13.4–14.1% (P. mirandai), 13.8% (Physaloptera sp. from USA), 13.9% (T. turgida), 14.9–15.1% (Physaloptera sp. from Mexico), 15.3% (Physaloptera sp. from Costa Rica), 18.9–19.3% (Physalopteridae gen sp. 1 and Physalopteridae gen sp. 2), 22.5% (H. longissimum), 22.3–23.3% (P. obtusus) and 32.7% (G. turgidum).
Phylogenetic trees produced with different datasets were poorly resolved, with few strongly supported branches, regardless of the inference method used. Consistent similarities were found mostly involving moderately to well-supported branches (supplementary files S11–S18). Heliconema and Proleptus species sequences formed a poorly to well-supported monophyletic group in all phylogenies using either 18S (MP-BS = 0.79; ML-SH = 0.67; BPP = 0.90) or MT-CO1 (MP-BS = 1.00; ML-SH = 0.99; BPP = 1.00) matrices (fig. 4 and 5).

Fig. 4. Phylogenetic relationships of Physalopteridae based on the MT-CO1 gene generated by Bayesian inference. Values of Bayesian posterior probabilities are on the tree nodes. The scale bar represents the number of substitutions per site.

Fig. 5. Phylogenetic relationships of Physalopteridae based on the nuclear ribosomal RNA small subunit (18S) gene generated by maximum likelihood. Values of local support values using the Shimodaira–Hasegawa test with 1000 resamples are on the tree nodes. The scale bar represents the number of substitutions per site.
Physalopterinae species sequences formed a moderately to well-supported monophyletic group in all phylogenies using either 18S (MP-BS = 0.75; ML-SH = 0.94; BPP = 0.99) or MT-CO1 (MP-BS = 1.00; ML-SH = 0.98; BPP = 1.00). Relationships within Physalopterinae were poorly resolved and most species were connected with polytomies or weakly supported branches. Turgida species sequences formed a poorly to well-supported monophyletic group in all phylogenies using either 18S (MP-BS < 0.50; ML-SH = 0.79; BPP = n/a) or MT-CO1 (MP-BS = 1.00; ML-SH < 0.50; BPP = 1.00), except for the BI-18S tree. Moreover, Turgida consistently nested within clades formed by Physaloptera species sequences, making Physaloptera paraphyletic in all phylogenies, using both matrices.
Regarding exclusively the 18S matrix, P. alata and P. apivori sequences formed a well-supported monophyletic group in all phylogenies (MP-BS = 0.97; ML-SH = 0.97; BPP = 1.00), whereas P. rara, P. thalacomys and Physaloptera sp. from USA sequences formed a poorly to well-supported monophyletic group in all but the BI tree (MP-BS < 0.50; ML-SH = 0.92; BPP = n/a).
Regarding exclusively the MT-CO1 matrix, P. mirandai sequences formed a well-supported monophyletic group in all phylogenies (MP-BS = 0.81; ML-SH = 1.00; BPP = 1.00), whereas P. bispiculata and P. galvaoi sequences both formed a well-supported monophyletic group in all phylogenies (MP-BS = 1.00; ML-SH = 0.98; BPP = 0.99).
Physaloptera amazonica n. sp. did not consistently cluster with other species in the 18S matrix. Instead, it sometimes grouped with Turgida species, Physaloptera sp. from China, Physaloptera sp. from Costa Rica, P. mirandai, P. bispiculata and P. galvaoi in a poorly resolved and poorly to moderately supported monophyletic group in all but the BI tree (MP-BS < 0.50; ML-SH = 0.84; BPP = n/a); or grouped with P. alata and P. apivori, P. bispiculata, P. galvaoi, P. mirandai, Physaloptera sp. from China, Physaloptera sp. from Costa Rica, Physalopteroides sp. and Turgida in a poorly resolved, well-supported monophyletic group in the BI consensus tree (BPP = 0.95). In the MT-CO1 matrix, P. amazonica n. sp. formed a well-supported monophyletic group with the P. bispiculata + P. galvaoi clade in all phylogenies (MP-BS = 0.98; ML-SH = 1.00; BPP = 1.00).
Discussion
The new species presented here belongs to the genus Physaloptera due to the following morphological features: cuticle forming a cephalic collarette at anterior end, two pseudolabia surrounding the oral opening with one external lateral tooth and internal lateral tripartite tooth, males with caudal alae well-developed presenting papillae (four lateral pedunculated pairs, three precloacal and five sessile postcloacal pairs), spicules sub-equal, females presenting vulva at the anterior third of the body and less than ten uterine branches (Travassos, Reference Travassos1920; Ortlepp, Reference Ortlepp1922; Morgan, Reference Morgan1947).
Comparing P. amazonica n. sp. with the species described in rodents from the Americas, it can be distinguished from P. longispicula Quentin, 1968, P. murisbrasiliensis Diesing, 1860, P. calnuensis Sutton, 1989 and P. bispiculata Vaz & Pereira, 1935 by having the first and second pair of sessile papillae asymmetrically organized, with the left one anterior to the right one. The new species differs from all Physaloptera parasites from American rodents by the spicule shape – that is, right one curved without dilatation and left one lanceolate at the final third. Moreover, the ratio of left spicule/total body length of P. amazonica n. sp. is larger than those of P. goytaca Ederli, Gallo, Oliveira & Oliveira, 2018, P. galvaoi São Luiz, Simões, Torres, Barbosa, Santos, Giese, Rocha & Maldonado Júnior, 2015, P. hispida Schell, 1950 and P. murisbrasiliensis. The position of the excretory pore from the anterior end in P. amazonica n. sp. is larger than in P. calnuensis and P. longispicula, both in male and female specimens. The number of uterine branches from the present species differs from P. hispida, P. bispiculata, P. murisbrasiliensis and P. goytaca by having four branches. The distance of vulva from the anterior end of the new species is larger than in P. bispiculata and P. calnuensis (table 2).
Table 2. Morphological features of Physaloptera males/females infecting rodents in the Americas.

All measurements are in millimetres.
a Distance from posterior end.
b Distance from anterior end.
RSp/BL and LSp/BL = ratio of right spicule/body total length or left spicule/body total length.
Currently, five species of Physaloptera infecting rodents have been reported, found in different biomes in Brazil: P. bispiculata parasitizing Nectomys squamipes Brants, 1827 and P. murisbrasiliensis in Holochilus brasiliensis (Desmarest, 1819) (syn. Mus brasiliensis), both in the Atlantic Rainforest; P. longispicula in Thrichomys apereoides (Lund, 1839) in Caatinga; P. galvaoi in Cerradomys subflavus (Wagner, 1842) in Cerrado; and P. goytaca in C. goytaca in Restinga (sandplain) (Ortlepp, Reference Ortlepp1922; Morgan, Reference Morgan1947; Vicente et al., Reference Vicente, Rodrigues, Gomes and Pinto1997; São Luiz et al., Reference São Luiz, Simões, Torres, Barbosa, Santos, Giese, Rocha and Maldonado2015; Ederli et al., Reference Ederli, Gallo, Oliveira and de Oliveira2018). In this study, P. amazonica n sp. represents the only species infecting an echimyid rodent in the Amazon forest.
Our phylogenetic analyses were inconclusive in some respects due to several reasons, as follows. (1) Insufficient taxonomic coverage: since Physaloptera is such a speciose genus, our datasets represented less than 10% of the total number of species recognized for the genus. (2) Taxonomic uncertainties: several sequences in each of our datasets have not been identified at the species level, some have not even been identified at the genus level. Moreover, there may be cryptic species of Physaloptera yet undetected. Finally, there is the issue of taxonomic reliability of DNA sequences in public sequence databases, which sometimes are not sufficiently complete or do not contain correctly and informatively annotated entries. Indeed, up to 20% of the named sequences in public databases may be misidentified (Vilgalys, Reference Vilgalys2003; Nilsson et al., Reference Nilsson, Ryberg, Kristiansson, Abarenkov, Larsson and Kõljalg2006). (3) Unbalanced taxonomic representativeness between matrices: not all taxa were represented in both matrices. (4) Molecular markers' evolutionary rates: MT-CO1 performs better at species-level differentiation, while 18S is more adequate at deeper nodes. However, MT-CO1 evolves faster and is more inclined to saturation, whereas 18S evolves slower, has a larger effective population size and is more inclined to incomplete lineage sorting. Therefore, including a third marker, with intermediate mutational rates, such as the ITS region, would provide a more complete perspective on the relationships between species of Physaloptera and genera of physalopterids. (5) Inference methods consistency: during analyses, we noticed that divergence levels and saturation were relatively low, even for the MT-CO1, although heterogeneous. In such situations, MP may perform better than probabilistic methods due to overparameterization (Russo et al., Reference Russo, Takezaki and Nei1996; Kolaczkowski & Thornton, Reference Kolaczkowski and Thornton2004) and lack of gap treatment of the last, particularly when there are gaps in the matrix (e.g. the 18S), which are treated as a fifth state in MP (Warnow, Reference Warnow2012). Indeed, we found more congruence between MP and ML than between ML and BI, in both matrices, suggesting inconsistency of the statistical methods for our data.
Relationships between most taxa within Physaloptera were poorly resolved in our phylogenies, producing multifurcations or a star phylogeny. Star-like phylogenetic patterns have been observed for different taxa, from viruses to vertebrates (Leite & Patton, Reference Leite and Patton2002; Castillo et al., Reference Castillo, Cortinas and Lessa2005; Almeida et al., Reference Almeida, Giannini, DeSalle and Simmons2011; Poon et al., Reference Poon, Walker, Murray, McCloskey, Harrigan and Liang2013), and may occur due to poor taxonomic sampling or lack of phylogenetic signal, either because of saturation in variable genes (such as the MT-CO1) or because of insufficient mutations in conserved genes (such as the 18S). Although both genes have very different mutation rates, as was demonstrated by our distance matrices, we found little saturation in most datasets, except for the third codon positions in the MT-CO1, and a strong phylogenetic signal conveyed by all datasets. Finally, the star-like pattern may be attributed to evolutionary processes where past simultaneous species diversification events took place. Explosive speciation processes in parasites may be a result of new host capture in a short span, enabling rapidly diverging lineages to persist over time, leading to short branches near the root and long branches at the tips. This is a reasonable conjecture for Physaloptera, considering that species are found infecting marsupials, rodents, reptiles, amphibians and birds (Honisch & Krone, Reference Honisch and Krone2008; Humberg et al., Reference Humberg, Tavares, Paiva, Oshiro, Bonamigo, Júnior and Oliveira2011; Pereira et al., Reference Pereira, Alves, Rocha, Lima and Luque2012; Quadros et al., Reference Quadros, Marques, Moura and Antonelli2014).
Despite all uncertainties concerning the phylogenies, there was a consensus, in our molecular analyses, that P. amazonica n. sp. belongs to a lineage independent of the other species of Physaloptera and should, therefore, be considered a separate species. Both pairwise genetic distances and phylogenetic trees approximated P. amazonica n. sp. to other Neotropical species, notably P. bispiculata and P. galvaoi.
Our phylogenetic analyses also showed Turgida consistently forming a monophyletic group, although not always well-supported. This group nested within Physaloptera, which was, therefore, paraphyletic, suggesting that Turgida may have evolved from a Physaloptera-like monodelphic ancestor and could be a derived form of Physaloptera, as pointed out by Ortlepp (Reference Ortlepp1922), stressing that polydelphy is a common trait among several species within both genera. Likewise, morphological diagnostic differences between Physaloptera and Turgida are inconsistent, so the use of morphological differences such as the number of uterus branches, pattern of caudal papillae arrangement, spicule size and shape should be viewed carefully and considered with caution, as it could also be the case that some species classified as Physaloptera really belong to other genera.
In conclusion, P. amazonica n. sp. is distinguished from other species of the genus by morphological and molecular evidence, emphasizing the importance of integrative taxonomy in supporting systematic studies of nematodes. Due to the great diversity of Physaloptera, further analyses should include more taxa, particularly the polydelphic ones, and more molecular markers for all taxa to elucidate the underlying evolutionary history between species of Physaloptera and genera of physalopterids.
Supplementary material
To view supplementary material for this article, please visit https://doi.org/10.1017/S0022149X19000610.
Acknowledgements
We thank the staff of the Laboratory for Biology and Parasitology of Wild Mammal Reservoirs of IOC/Fiocruz, Brazil, for helping in the field work, and Ricardo Baptista Schmidt of the Image Production and Treatment Service, IOC/Fiocruz,.
Financial support
AMJ received a grant from the National Council for Scientific and Technological Development – CNPq (grant number 306352/2014-1). ROS received a grant from the Rio de Janeiro State Research Foundation – FAPERJ (grant number E-26/202.128/2015).
Conflicts of interest
None.
Ethical standards
Animals were captured under the authorization of the Brazilian government's Chico Mendes Institute for Biodiversity and Conservation (ICMBIO, license number 13373, 45839-2, 17131-4), the Santa Catarina State Environmental Foundation (FATMA, license number 043/2014) and the Rio de Janeiro State Environmental Institute (INEA-020/2011). All procedures followed the guidelines for capture, handling and care of animals of the Ethical Committee on Animal Use of the Oswaldo Cruz Foundation (CEUA FIOCRUZ license number 066/08; L-049/08, LW81/12 and 39/14, CEUA UESC-003/2013).