Introduction
Aquatic ecotoxicity studies on the effects of contaminants of emerging concern (CECs) such as microplastics, anthropogenic nanomaterials and pharmaceuticals have rapidly increased in number in the last decade, focusing mainly on freshwater rather than saltwater or terrestrial environments (Dulio et al. Reference Dulio, van Bavel, Brorström-Lundén, Harmsen, Hollender and Schlabach2018). Pollution is one of the global anthropogenic drivers whose impact is expected to increase in the Antarctic in the near future (Morley et al. Reference Morley, Abele, Barnes, Cárdenas, Cotté and Gutt2020). Legacy anthropogenic pollutants such as persistent organic pollutants can reach Antarctica via long-range transport (Krasnobaev et al. Reference Krasnobaev, Ten Dam, Boerrigter-Eenling, Peng, Van Leeuwen and Morley2020), whereas CECs may also originate from local sources (e.g. activities related to fishing, tourism and scientific research), and if they are not monitored and regulated, CECs have the potential to accumulate in the environment and have adverse effects on polar wildlife.
The study of CECs in polar environments poses a challenge as few data are currently available regarding their sources, fate and impact in comparison with the intense research conducted at lower latitudes. Among CECs, anthropogenic nanoscale materials and nanoparticles (NPs) pose great concern due to their ability to cause a variety of injuries in exposed species (Blasco & Corsi Reference Blasco and Corsi2019). Nanoscale titanium dioxide (n-TiO2) is recognized globally as a potential hazard for humans and the environment (Sauvé & Desrosiers Reference Sauvé and Desrosiers2014, Slijkerman & Keur Reference Slijkerman and Keur2018). They are frequent components of commercial and industrial products (Gottschalk et al. Reference Gottschalk, Sonderer, Scholz and Nowack2009), and due to their photocatalytic and antibacterial properties they are widely present in commercial and consumer products including paints, sunscreens and textiles (Robichaud et al. Reference Robichaud, Uyar, Darby, Zucker and Wiesner2009, Ziental et al. Reference Ziental, Czarczynska-Goslinska, Mlynarczyk, Glowacka-Sobotta, Stanisz, Goslinski and Sobotta2020). Titanium dioxide NP occurrences in lakes and coastal seawaters (top surface layer and in the water column) have been demonstrated recently, with concentrations in the range of 10–900 μg l-1, probably being released from sunscreens (Gondikas et al. Reference Gondikas, von der Kammer, Reed, Wagner, Ranville and Hofmann2014, Labille et al. Reference Labille, Slomberg, Catalano, Robert, Apers-Tremelo and Boudenne2020). The ecotoxicity of titanium dioxide NPs has been widely investigated and several injuries have been documented in marine species belonging to different trophic levels. Titanium dioxide NPs can also be transferred to and along food webs (i.e. through bioaccumulation and biomagnification) and can exceed toxicity threshold levels for aquatic species (Corsi et al. Reference Corsi, Bergami and Grassi2020).
Polar regions are therefore not excluded from the potential threat posed by n-TiO2, as personal care products, ultraviolet (UV)-filter sunscreens and pharmaceuticals have been already reported in Antarctic coastal waters in the vicinity of Antarctic research stations (Emnet et al. Reference Emnet, Gaw, Northcott, Storey and Graham2015, Domínguez-Morueco et al. Reference Domínguez-Morueco, Moreno-Merino, Molins-Delgado, Díaz-Cruz, Aznar-Alemany and Eljarrat2021). Sewage can carry a significant amount of household products, increasing the risk of exposure for both fresh and marine species (Baker et al. Reference Baker, Tyler and Galloway2014). Nanoparticles have sizes < 100 nm and are characterized by a colloidal behaviour in water bodies, being susceptible to agglomeration and sedimentation (Klaine et al. Reference Klaine, Alvarez, Batley, Fernandez, Handy and Lyon2008). The wastewater treatment plants located in Antarctica receive various types of wastes, fats, oils and greases and also products containing NPs such as microplastics and nanometals. Depending on the type of plant and its maintenance, in some cases NPs may not be retained and may be released into the environment. Although polar species are well adapted to extreme but relatively stable environmental conditions, they are more vulnerable to environmental perturbations, including anthropogenic pollutants, compared to species from lower latitudes (Grotti et al. Reference Grotti, Soggia, Lagomarsino, Riva, Goessler and Francesconi2008).
The fairy shrimp Branchinecta gaini (Daday 1910) is the largest freshwater invertebrate species in Antarctica and the only species of Anostraca present in the South Shetland Islands (Maritime Antarctica) and the Antarctic Peninsula (Nedbalová et al. Reference Nedbalová, Nývlt, Lirio, Kavan and Elster2017). Although the biology and ecology of B. gaini are known (Peck Reference Peck2004, Hawes Reference Hawes2008, Pociecha & Dumont Reference Pociecha and Dumont2008), its suitability as a biomonitor of anthropogenic pollutants and CECs has not been investigated thus far. Other species of Anostraca (e.g. brine shrimp Artemia spp.) have been widely used as models in ecotoxicity studies and recognized as suitably sensitive species for water pollution monitoring and impact assessment (Ates et al. Reference Ates, Daniels, Arslan and Farah2013, Libralato et al. Reference Libralato, Prato, Migliore, Cicero and Manfra2016, Bergami et al. Reference Bergami, Pugnalini, Vannuccini, Manfra, Faleri and Savorelli2017).
Here, for the first time, we investigated the ability the Antarctic fairy shrimp B. gaini to cope with n-TiO2 exposure by analysing the expression of genes involved in stress response (e.g. antioxidant, moulting and xenobiotic responses) with acute, short-term (9 h) in vivo n-TiO2 exposure (in the concentration range of 50–200 μg ml-1). Some of these stress-response genes as well as those involved in moulting have already been shown to be regulated by other nanoscale particles (i.e. functionalized polystyrene NPs) in aquatic crustaceans in both acute short-term and long-term exposure conditions (Bergami et al. Reference Bergami, Bocci, Vannuccini, Monopoli, Salvati, Dawson and Corsi2016, Reference Bergami, Pugnalini, Vannuccini, Manfra, Faleri and Savorelli2017, Reference Bergami, Manno, Cappello, Vannuccini and Corsi2020, Varó et al. Reference Varó, Perini, Torreblanca, Garcia, Bergami, Vannuccini and Corsi2019).
Methods
Sample collection and experiment
A total of 80 specimens of B. gaini were collected during the 2018–2019 summer (January–February) from a lake in Fildes Bay near Professor Julio Escudero scientific base (Instituto Antartico Chileno; INACH) on King George Island. Individual specimens were placed in polypropylene plastic containers filled with rock pool water from Laguna INACH (62°12.128'S, 58°57.992'W) at an ambient temperature of 4 ± 2°C.
Titanium (IV) oxide (n-TiO2) anatase nanopowders (< 25 nm particle size, 99.7% trace metal basis) were purchased from Sigma-Aldrich (St Louis, MO, USA). Working solutions of n-TiO2 were prepared in 0.2 μm filtered rock pool waters from a stock of 1 mg ml-1 in Milli-Q® water (mQW; ELGA LabWater, Sartorius, UK). Suspensions were sonicated for 20 m in an ultrasonic bath (Branson, Inc., Danbury, CT, USA) before and after dilution to prevent aggregation.
Three individual fairy shrimp specimens were placed in each 50 ml polypropylene graduated plastic container and exposed to 50, 100 and 200 μg ml-1 suspensions of n-TiO2 in rock pool water; a control group was exposed to rock pool water only. Experiments were run for 9 h according to the 202 OECD acute toxicity protocol (OECD 2004). After 9 h, fairy shrimps were removed and placed in RNAlater™ (Sigma-Aldrich) following the protocol of the manufacturer and stored at -80°C for gene expression analysis. Each experiment was repeated twice. Mortality was assessed by behaviour change and immobility of animals after 9 h.
Characterization of n-TiO2
The stability and agglomeration of n-TiO2 suspensions (at 200 μg ml-1) in mQW and rock pool waters (exposure medium) were determined using dynamic light scattering (DLS; Malvern Instruments, Malvern, UK) combined with the Zetasizer Nano Series software, version 7.02 (Particular Sciences, Dublin, Ireland). The Z-average (nm), polydispersity index (PDI; dimensionless) and ζ-potential (mV) were measured at 4°C as key parameters describing NP behaviour in complex environmental media (SCENIHR 2007, Stone et al. Reference Stone, Nowack, Baun, van den Brink, von der Kammer and Dusinska2010). Measurements were carried out at different time intervals (0, 3, 6 and 12 h) and were performed in triplicate, with each measurement set as 11 runs of 10 s (Z-average) or 20 runs (ζ-potential) according to Bergami et al. (Reference Bergami, Pugnalini, Vannuccini, Manfra, Faleri and Savorelli2017). The initial aggregation kinetics of n-TiO2 within 1.2 h after suspension in mQW and rock pool water were further investigated as individual sequential measurements at 6 min intervals.
RNA extraction, sequencing and assembly
RNAlater was removed from samples and RNA extracted according to the protocol of the E.Z.N.A.® Total RNA Kit (Omega Bio-tek, Norcross, GA, USA). RNA quality and quantity were determined on a 2100 Bioanalyzer (Agilent Technologies, Santa Clara, CA, USA). Quality control assessment gave a RNA integrity number (RIN) of > 7.5. An RNA library was produced using 1 μg of total RNA with a TruSeq Stranded mRNA kit (Illumina, San Diego, CA, USA). High-throughput sequencing was performed on a HiSeq 2500 sequencer provided by Macrogen (Seoul, South Korea) using ~40 million and a 2 × 100 base pair (bp) paired-end strategy. Trimmed reads were assembled using Trinity version 2.4.0 including an in silico read normalization for sequences with a minimum length of 200 bp. The resulting assembly contained 43 243 transcripts, a length observed for 50% of the contigs assembled (contig N50) of 1253 bp and an average length of 85 339 bp.
RNA sequence database annotation and identification of candidate genes
The nucleotide sequences of the genes coding for several heat-shock proteins (Hsps; Hsp83, Hsp90a, Hsp90b, Hsp70m, Hsp70a, Hsp70b), caspase 1, glutathione peroxidase (GPx), superoxide dismutase (Sod), cytochrome P450 4C1-like (P450) and cathepsin L precursor (Clap) were obtained from the transcriptome of B. gaini (Bioproject ID PRJNA661774). The degree of homology of these sequences was confirmed by similarity search using the Blastx program (NCBI, Bethesda, MD, USA), where amino acid sequences were extracted for multiple alignment using the CLC Main Workbench (QIAGEN, Hilden, Germany) to determine conserved areas. Subsequently, the primers were designed from the conserved areas using the software Primer 3.0.
Gene expression analysis through real-time quantitative polymerase chain reaction
In order to protect Antarctic ecosystems and to encourage preventative mitigation strategies against the potential negative effects from CEC exposure, here we investigated the ability of an Antarctic freshwater crustacean (B. gaini) to cope with n-TiO2 exposure by analysing the expression of several genes involved in stress response (Table I). The candidate genes were investigated through quantitative real-time quantitative polymerase chain reaction (RT q-PCR), using the mean expression of glyceraldehyde 3-phosphatase dehydrogenase (Gapdh) and low-density lipoprotein receptor-related protein 8 (Lrp8) as housekeeping genes. The retrotranscription was performed using 1 μg of RNA extracted by means of the M-MLV Reverse Transcriptase kit (Invitrogen, Waltham, MA, USA), using random hexamers and oligo(dT) as primers for the synthesis of cDNA. RT q-PCR was carried out using the Brilliant II SYBR® Green QPCR Master Mix kit (Agilent Technologies) in an Mx3005P QPCR System thermocycler (Agilent Technologies), analysing each gene in triplicate for each sample. The validation of Lrp8 and Gapdh as constitutive genes was carried out using the BestKeeper software (Pfaffl et al. Reference Pfaffl, Tichopad, Prgomet and Neuvians2004). The comparison of the expression levels of the targets genes was carried out by the 2-ΔΔC(T) method (Livak & Schmittgen Reference Livak and Schmittgen2001). Further details on primer sequences are presented in Table S1.
Table I. Results from the Blastx sequence similarity searches of the target genes in the Branchinecta gaini transcriptome.

Statistical analysis
Gene expression datasets were tested for Gaussian distribution using the Shapiro-Wilk test. Homogeneity of variance was assessed using the Brown-Forsythe test for equal variances. Expression data of the reference genes were tested for differences between treatments using one-way analysis of variance and Tukey's post hoc test. In case of non-normally distributed data and non-equal variances, the analysis was performed using Mann-Whitney tests. All statistical analyses were conducted using the GraphPad Prism version 7.0 software for Windows (GraphPad Software, San Diego, CA, USA).
Results
None of the tested concentrations (range 50–200 μg ml-1) cause mortality in the Antarctic fairy shrimps. The stability and particle size distribution of n-TiO2 in mQW and rock pool waters were investigated at time 0 and after 3, 6 and 12 h. The DLS results showed similar patterns for n-TiO2 in rock pool waters and in mQW soon after preparation of the suspensions (Fig. 1 & Table S2). The aggregation kinetics within the first hour after dispersion confirmed the presence of stable populations of n-TiO2 aggregates with sizes of ~1500 and 1800 nm in mQW and rock pool waters, respectively (Fig. 2a).
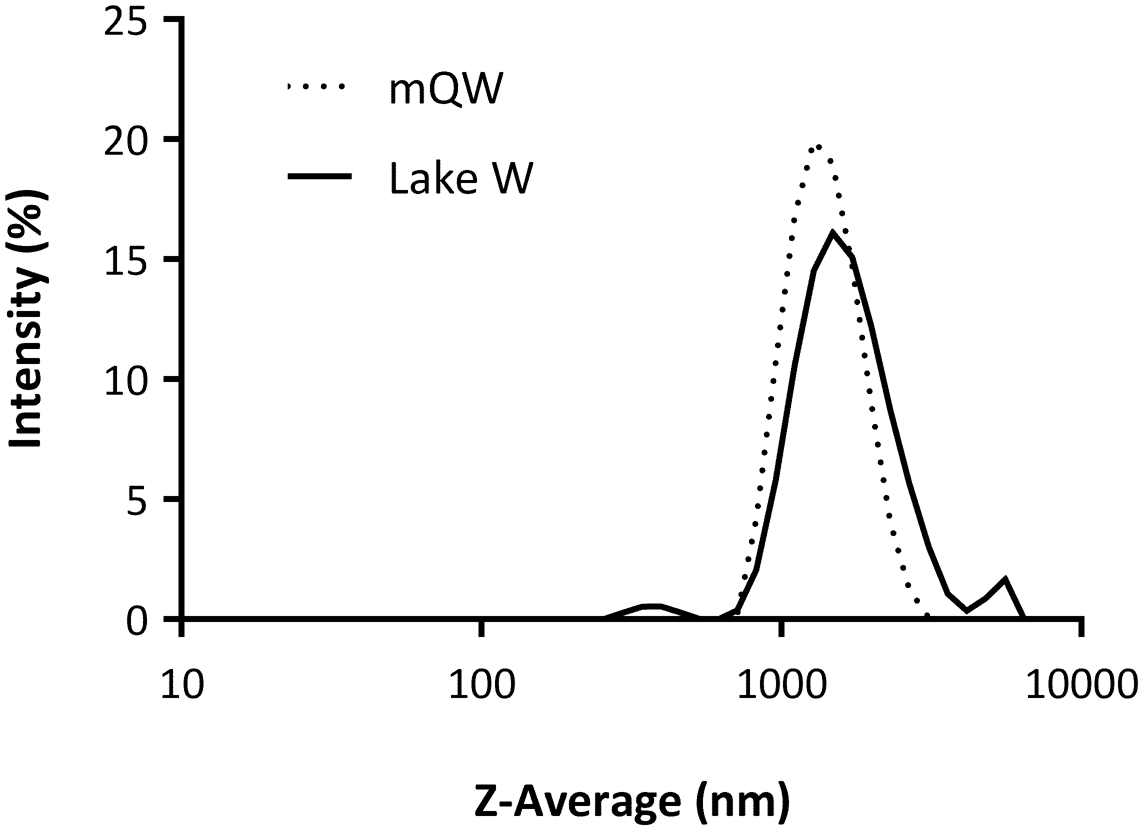
Fig. 1. Physicochemical characterization of nano-TiO2 (200 μg ml-1) in Milli-Q water (mQW; as reference medium) and rock pool water (Lake W; 0.20 μm filtered, pH 8.22, 4°C) via dynamic light scattering. Intensity-weighted size distributions of Z-averages (nm) are shown on a logarithmic scale (x-axis) starting at 10 nm, representing averages of three measurements for each medium.

Fig. 2. Aggregation kinetics of nano-TiO2 (at 200 μg ml-1) in rock pool water (Lake; 0.20 μm filtered, pH 8.22, 4°C) and Milli-Q water (mQW). a. Z-average values (nm) and b. polydispersity index (PDI; dimensionless) obtained via dynamic light scattering are reported as means ± SDs of three measurements each at 0, 3, 6 and 12 h after preparation and as single measurements at 6 min intervals within 1.2 h of suspension.
However, in the exposure medium (i.e. rock pool water), a strong agglomeration and a broader size distribution of n-TiO2 occurred with time, as is shown by the PDI values (Fig. 2b), with an increase in hydrodynamic diameter observed at 6 and 12 h corresponding to Z-averages of 2000 ± 120 and 4024 ± 1042, respectively (Fig. 2 & Table S2). The agglomeration of n-TiO2 thus appeared to be time-dependent.
All genes involved in antioxidant response capacity, moulting and xenobiotic responses were upregulated after n-TiO2 exposure (Fig. 3). The very short exposure time (9 h) allowed us to observe the expression of the genes at an early stage of the toxicity response.

Fig. 3. Gene expression in Branchinecta gaini after exposure to nano-TiO2 determined using real-time quantitative polymerase chain reaction. Cell stress responses are shown for genes involved in a. xenobiotic response, b. moulting and apoptosis processes and c. antioxidant activity. All data were obtained from two independent experiments and each experiment was performed in triplicate. Significant differences in the Hsp70a and P450 expression levels in terms of two-group comparisons were assessed using Mann-Whitney tests. Asterisks represent Tukey's post hoc test results following one-way analysis of variance, expressed as means ± standard errors: *P < 0.05, **P < 0.01, ***P < 0.0001.
Our study investigated such responses at the molecular level in B. gaini, where the gene expression levels of Sod (50 μg ml-1, P = 0.0260; 200 μg ml-1, P = 0.0087) and GPx (50 μg ml-1, P = 0.0033; 100 μg ml-1, P = 0.0187; 200 μg ml-1, P = 0.0432) showed significant downregulation following n-TiO2 exposure, suggesting a decreased capacity to reduce reactive oxygen species (ROS) in exposed specimens (Fig. 3a).
In order to better understand the molecular mechanisms by which n-TiO2 could induce toxicity, the expression levels of five Hsps were investigated. Our results showed a gradual upregulation of only Hsp70a compared with the control when exposed to high concentrations of n-TiO2; however, significant upregulation was only found in specimens exposed to the highest concentration of n-TiO2 (200 μg ml-1; P = 0.0411; Fig. 3b) when the data were analysed in a non-parametric way.
The expression of the Cyp450 gene in B. gaini was upregulated at 100 and 200 μg ml-1 of n-TiO2 (P = 0.0087 and P = 0.087, respectively; Fig. 3c), suggesting that this gene may be involved in the response to n-TiO2. On the other hand, strong downregulation of Clap gene expression was also observed at all concentrations tested (50 μg ml-1, P = 0.0001; 100 μg ml-1, P = 0.0001; 200 μg ml-1, P = 0.0001; Fig. 3c). Finally, the relative expression level of the caspase-1 transcript remained unchanged with respect to the control (Fig. 3c), indicating that n-TiO2 did not affect the expression of this gene involved in the apoptotic process.
Discussion
Although the occurrence of n-TiO2 in the Antarctic environment has not been reported yet, traces of pharmaceutical and personal care products, including sunscreens, have been found in sewage effluents, costal seawaters and sea ice near Antarctic research stations (Emnet et al. Reference Emnet, Gaw, Northcott, Storey and Graham2015). As a UV blocker, n-TiO2 pigments are commonly used in several commercial products (e.g. paints, sunscreens and textiles), and although its release from formulations has been demonstrated (Al-Kattan et al. Reference Al-Kattan, Wichser, Vonbank, Brunner, Ulrich, Zuin and Nowack2013, Tovar-Sánchez et al. Reference Tovar-Sánchez, Sánchez-Quiles, Basterretxea, Benedé, Chisvert and Salvador2013, Gondikas et al. Reference Gondikas, von der Kammer, Reed, Wagner, Ranville and Hofmann2014), there are still major technical limitations to accurately detecting and quantifying its occurrence in wastewater discharges and to linking such exposures to the documented effects on aquatic biota. Here, we provide the first results showing the responsiveness and sensitivity of an Antarctic freshwater crustacean to n-TiO2 exposure and highlight its suitability as a biomonitor of CECs in Antarctica.
In aquatic environments, the agglomeration of n-TiO2 has been found to be controlled by the presence and concentration of dissolved and particulate organic matter, pH and ionic strength (Brunelli et al. Reference Brunelli, Pojana, Callegaro and Marcomini2013). The resulting micron and submicron-sized n-TiO2 agglomerates have been found to be taken up by organisms and producing toxic effects and changes in the expression of some genes, such as the suppression of antioxidant-related genes (Corsi et al. Reference Corsi, Bergami and Grassi2020).
To date, nano-ecotoxicity studies evaluating the acute effects of NPs on crustaceans at the molecular level have mostly considered gene expression related to antioxidant enzymes such as Sod, catalase, GPx and glutathione S-transferase (Kim et al. Reference Kim, Klaine, Cho, Kim and Kim2010, Clemente et al. Reference Clemente, Castro, Jonsson and Fraceto2014, Kögel et al. Reference Kögel, Bjorøy, Toto, Bienfait and Sanden2020). Recently, Thiagarajan et al. (Reference Thiagarajan, Seenivasan, Jenkins, Chandrasekaran and Mukherjee2020) showed that n-TiO2 caused reduced Sod activity in Artemia salina. It is well known that n-TiO2 increases ROS production and could activate antioxidant defence mechanisms such as Sod and GPx to protect cells from oxidative stress. In some cases, the literature remains inconclusive regarding the effects of n-TiO2, with studies reporting contradictory findings on the activity of these enzymes (Clemente et al. Reference Clemente, Castro, Jonsson and Fraceto2014).
Regarding cellular responses to exposures to toxicants such as nanometals, these could be used as early markers of toxicity via the induction of Hsp expression. The effect of n-TiO2 on the overexpression of the Hsp70 gene has been demonstrated previously in the human bronchial epithelial cell line (Okuda-Shimazaki et al. Reference Okuda-Shimazaki, Takaku, Kanehira, Sonezaki and Taniguchi2010). However, in the human alveolar type II-like epithelial cell line n-TiO2 induced cytotoxicity but did not induce Hsp70 and Grp78 expression (Aueviriyavit et al. Reference Aueviriyavit, Phummiratch, Kulthong and Maniratanachote2012). Previous research showed that the crustaceans Artemia franciscana and Daphnia magna respond to environmental stress by producing the Hsps, which play an important role in mitigating cellular damage (Kim et al. Reference Kim, Kim and Lee2017, Gbotsyo et al. Reference Gbotsyo, Rowarth, Weir and MacRae2020). However, whether Hsps are involved in the biological responses to n-TiO2 in crustaceans is currently unknown. Decreased detoxification due to Hsp declines also lead to tissue injury or cell death (Shah et al. Reference Shah, Shah, Hussain and Khan2017).
The sequence identified in the transcriptome of B. gaini belongs to the Cyp4 family of proteins, which are involved in ecdysteroids hormone metabolism and xenobiotic metabolism in arthropods (James & Boyle Reference James and Boyle1998, Baldwin et al. Reference Baldwin, Marko and Nelson2009). The increased expression of Cyp450 may be responsible for hormone synthesis and metabolism, resulting in endocrine disruption. Therefore, the observed upregulation of this gene raises further questions regarding the ability of n-TiO2 to disrupt hormone synthesis and metabolism in the fairy shrimp. Cytochrome P450 monooxygenases catalyse the oxidation and metabolism of a large number of endogenous compounds, including xenobiotics (Shankar & Mehendale Reference Shankar and Mehendale2014). In marine invertebrates, P450s play a key role in physiological adaptation to environmental pollutants (Rewitz et al. Reference Rewitz, Styrishave, Løbner-Olsen and Andersen2006).
Clap is involved in key processes related to crustacean development, particularly moulting (Le Boulay et al. Reference Le Boulay, Sellos and Van Wormhoudt1998, Butler et al. Reference Butler, Aiton and Warner2001). Qiao et al. (Reference Qiao, Wang, Mao, Liu, Song and Su2017) demonstrated moulting stage-regulated expression during the five moulting stages of a kuruma shrimp Marsupenaeus japonicus, indicating its role in the ontogenic development of this species. The full-length cDNA sequences of Clap isolated from B. gaini has two main domains characteristic of proteases (the cathepsin pro-peptide inhibitor domain (I29) and the papain family cysteine protease), confirming its potential functional role. Zhang et al. (Reference Zhang, Liu, Tang, Li, Jiang and Zhang2020) have reported a similar reduction in cathepsin gene expression in Daphnia pulex upon exposure to polystyrene NPs for 21 days). The contribution of caspases to the apoptotic process is evolutionarily conserved (Menze et al. Reference Menze, Fortner, Nag and Hand2010). The role of caspase 1 is well determined in vertebrates, and the activated caspase 1 has the capacity to cleave IL-1β into the mature protein form. However, reports characterizing the biochemical regulation of caspases in crustaceans are scarce and focused more on their cloning and molecular characterization. Caspase 1-mediated cell death was activated by white spot syndrome virus to counteract viral infection (Yang et al. Reference Yang, Wang, Luo and Zhang2019), and in another study, the expression of caspase-1 in Artemia sinica was measured at various times during embryonic development, and it was found that gene expression increased during early embryo development (Chu et al. Reference Chu, Yao, Cheng, Wu, Mei and Li2014).
Our results provide the first assessment of the acute short-term effects of n-TiO2 on an Antarctic freshwater crustacean species. Future studies should focus on toxicity over longer exposure times and at relevant predicted environmental concentrations, if available. Furthermore, in order to predict the consequences for early life stages, studying the effects of n-TiO2 on the embryonic and larval stages of B. gaini is recommended. In this regard, the transcriptomic analysis performed on B. gaini recommends its use as a suitable model organism to monitor the ice-free freshwater systems of the Maritime Antarctic and the Antarctic Peninsula region, which are areas of high anthropogenic activity and hence heavily influence the current and predicted impacts of contamination and climate change (Siegert et al. Reference Siegert, Atkinson, Banwell, Brandon, Convey and Davies2019, Morley et al. Reference Morley, Abele, Barnes, Cárdenas, Cotté and Gutt2020).
Future studies assessing the risk posed by n-TiO2 as a model CEC to Antarctic biota under relevant natural scenarios are encouraged, as well as those aiming to determine the environmental concentrations of this pollutant to facilitate environmental management.
Supplemental material
Supplemental tables can be found at https://doi.org/10.1017/S0954102022000086.
Acknowledgements
Thanks are due to all of the members of the IDEAL team for their help during fieldwork. We also thank the crew of Professor Julio Escudero Station (INACH) for their logistical support. Dynamic light scattering analysis was performed at the Centro Ricerca Energia e Ambiente (CREA; University of Siena, Italy: www.crea.unisi.it). Thanks are given to two anonymous reviewers for their feedback.
Financial support
The study was financed by grants from ANID-Chile (Fondecyt 11190802).
Author contributions
MG-A, GI and EB conceived the experimental design. GI carried out the experimental assays. EB carried out the dynamic light scattering analysis. RR and AF carried out the sequence analyses and transcriptome production. GI and MG-A carried out the statistical analyses. EB, IC, CAC and MG-A wrote the manuscript with contributions from AF, RR and GI. All authors read and approved the final manuscript.