Introduction
The ovule, contemporaneous with the origin of leaves, is the plant reproductive and lateral organ where the fertilization, zygotic embryogenesis and finally the development of seeds, takes place (Galbiati et al., Reference Galbiati, Roy, Simonini, Cucinotta, Ceccato, Cuesta, Simaskova, Benkova, Kamiuchi, Aida, Weijers, Rudiger, Masiero and Colombo2013). Ovule diversity has a relatively stable feature in evolution (Endress, Reference Endress2011a). In Arabidopsis, the ovule is constituted of the funiculus (which transports nutrients, via the phloem, from the mother plant), the nucellus (which originates the female gametophyte) and the chalaza (CZSC; originates both outer (OI) and inner (II) integuments). The seed is the reproductive entity of flowering plants constituted by the embryo and the endosperm, which are two fertilization products surrounded by an entirely maternal (diploid) and non-fertilized covering named the seed coat (SC) (Olsen, Reference Olsen2004; Ingram, Reference Ingram2010; Endress, Reference Endress2011a,b). After fertilization, the integuments give rise to the SC, which in angiosperms comprises the testa and tegmen, derived from the OI and II, respectively. Contrary to the maternal SC, essential loci are expressed in the endosperm from the paternal genome immediately after fertilization (Aw et al., Reference Aw, Hamamura, Chen, Schnittger and Berger2010). Thus, by using cdka1 mutants (CYCLIN DEPENDENT KINASE A1, also known as CDC2) the failure of karyogamy (i.e. fusion of two haploid nuclei during sexual reproduction) was achieved in the central cell, preventing the incorporation of the paternal genome and endosperm development. Does this mean that the inheritance of integuments is maternally controlled (Berger et al., Reference Berger, Grini and Schnittger2006)? In short, the SC does not take part in the fertilization process and it is derived from the ovule integument(s) after having undergone some of the most striking known embryogenic differentiation processes (Radchuk and Borisjuk, Reference Radchuk and Borisjuk2014; Coen et al., Reference Coen, Fiume, Xu, De Vos, Lu, Pechoux, Lepiniec and Magnani2017; Coen and Magnani, Reference Coen and Magnani2018). However, the factor(s) triggering integument development are yet to be described in detail. As discussed below, it is suggested that the starting signal(s) is generated in the fertilized central cell/endosperm and the phytohormone auxin could be a strong candidate (Figueiredo et al., Reference Figueiredo, Batista, Roszak, Henning and Köhler2016; Figueiredo and Köhler, Reference Figueiredo and Köhler2018). The signalling pathway initiated by the growing endosperm is required for the formation of all integuments. This process proceeds through the action of AGL62, an AGAMOUS-LIKE MADS-box transcription factor (TF) specifically expressed in the syncytial endosperm (Roszak and Köhler, Reference Roszak and Köhler2011; Figueiredo et al., Reference Figueiredo, Batista, Roszak, Henning and Köhler2016). Plants lacking AGL62 show a premature endosperm cellularization and a seed-lethal phenotype. In response to this endosperm key signal, the SC cell layers (integuments) undergo a rapid phase of cell division and expansion, and follow different cell fates (Haughn and Chaudhury, Reference Haughn and Chaudhury2005). In contrast, the elimination of the SC or nucellus is triggered by the endosperm (Xu et al., Reference Xu, Fiume, Coen, Pechoux, Lepiniec and Magnani2016). All Arabidopsis integuments were thought to respond homogeneously to the fertilization of the central cell and to grow in a coordinated way with the endosperm (Coen et al., Reference Coen, Fiume, Xu, De Vos, Lu, Pechoux, Lepiniec and Magnani2017). However, it was recently demonstrated that ii1’, a highly vacuolated sub-epidermal cell layer from Arabidopsis, has a unique developmental program (Coen et al., Reference Coen, Fiume, Xu, De Vos, Lu, Pechoux, Lepiniec and Magnani2017). This interaction between the endosperm and integuments determines the final size of the seed (Li and Li, Reference Li and Li2015; Orozco-Arroyo et al., Reference Orozco-Arroyo, Paolo, Ezquer and Colombo2015). Accordingly, (i) defects in SC growth (e.g. transparent testa glabra 2; TTG2) may negatively impact endosperm growth (García et al., Reference García, Fitz Gerald and Berger2005); (ii) deterioration of endosperm proliferation has a harmful effect on SC development (García et al., Reference García, Saingery, Chambrier, Mayer, Jürgens and Berger2003, Reference García, Fitz Gerald and Berger2005; Luo et al., Reference Luo, Dennis, Berger, Peacock and Chaudhury2005); and (iii) increased integument cell proliferation (e.g. megaintegumenta/auxin responsive factor2) results in enlarged seeds with more abundant endosperm (Schruff et al., Reference Schruff, Spielman, Tiwari, Adams, Fenby and Scott2006).
Integuments are the layers of tissue that usually cover the ovule, enveloping the nucellus and forming the micropyle (i.e. a small opening through which the pollen tube penetrates for ovule fertilization) at the seed apex. In Arabidopsis, the beginning of seed integument development is characterized by an increase in mitotic activity after fertilization, followed by a sharp decline leading to a complete absence of cell division 4 days after pollination (DAP) (García et al., Reference García, Fitz Gerald and Berger2005; Locascio et al., Reference Locascio, Roig-Villanova, Bernardi and Varotto2014). Likewise, after fertilization the integument cell layers display expansion growth that is not homogeneous (Coen et al., Reference Coen, Fiume, Xu, De Vos, Lu, Pechoux, Lepiniec and Magnani2017). Integument II arises first, followed closely by the OI (Endress, Reference Endress2011a). This event is supported by the fact that the absence of the II is always accompanied in known mutants by the absence of the OI. However, the micropyle only crosses integument II in the most ancient angiosperm Amborella trichopoda (Tobe et al., Reference Tobe, Jaffré and Raven2000). Among other important peculiarities, the integuments provide and constitute the cavity for the growth of both embryo and endosperm after fertilization (Roszak and Köhler, Reference Roszak and Köhler2011; Lafon-Placette and Köhler, Reference Lafon-Placette and Köhler2014; Li and Li, Reference Li and Li2015). As SC growth proceeds by a process driven mostly by endosperm cell elongation, it is most likely that auxin synthesized in the fertilized central cell/endosperm and then exported to the integuments, is the trigger for SC formation (Locascio et al., Reference Locascio, Roig-Villanova, Bernardi and Varotto2014; Figueiredo et al., Reference Figueiredo, Batista, Roszak, Henning and Köhler2016). The SC grows from the CZSC and protects and ensures the effective response of the seed to both biotic and abiotic factors to reach seed maturity and facilitates the balance between seed dormancy and germination (Radchuk and Borisjuk, Reference Radchuk and Borisjuk2014). Taken together, once the SC, the endosperm and the embryo are completely differentiated, the storage compounds accumulate, desiccation tolerance is acquired, and, finally, primary seed dormancy is triggered (Graeber et al., Reference Graeber, Nakabayashi, Miatton, Leubner-Metzger and Soppe2012; Harada and Pelletier, Reference Harada and Pelletier2012; Smÿkal et al., Reference Smÿkal, Vernoud, Blair, Soukup and Thompson2014; Chahtane et al., Reference Chahtane, Kim and López-Molina2017).
Accordingly, angiosperm evolution kept the SC as a multi-functional and key organ for viable seeds (Linkies et al., Reference Linkies, Graeber, Knight and Leubner-Metzger2010). That is, the SC is not only a mechanical and chemical protective envelope, but also a dynamic tissue that plays an indispensable role in a diverse set of processes such as maintenance of the balance between dormancy and germination, imbibition and nutrition of the endosperm and growing embryo (Beeckman et al., Reference Beeckman, De Rycke, Viane and Inze2000; Graeber et al., Reference Graeber, Nakabayashi, Miatton, Leubner-Metzger and Soppe2012; Vogiatzaki et al., Reference Vogiatzaki, Celia Baroux, Jung and Poirier2017; Carrillo-Barral et al., Reference Carrillo-Barral, Matilla, Rodríguez-Gacio and Iglesias-Fernández2018). In Arabidopsis thaliana, sugars are unloaded to the SC via the funicular phloem, which is symplastically connected to the integument OI (Stadler et al., Reference Stadler, Lauterbach and Sauer2005). Thus, three members of the SWEET transporter family are directly responsible for the translocation of sucrose through the integuments (Chen et al., Reference Chen, Lin, Qu, Sosso, McFarlane, Londono, Samuels and Frommer2015). The CZSC is the zone where the vascular vessels end in many seeds, including Arabidopsis. Therefore, CZSC represents an area of nutrients unloading and an important interface connecting the maternal and filial tissues (Patrick and Offler, Reference Patrick and Offler2001; Stadler et al., Reference Stadler, Lauterbach and Sauer2005; Vogiatzaki et al., Reference Vogiatzaki, Celia Baroux, Jung and Poirier2017). It is not surprising then that a considerable number of genes involved in water, sugar or amino acid transport are more expressed in the maturing zone of CZSC than in the distal SC (Obroucheva, Reference Obroucheva2013; Khan et al., Reference Khan, Millar, Girard and Belmonte2014). However, A. trichopoda lacks vascular bundles in the integuments (Tobe et al., Reference Tobe, Jaffré and Raven2000). The absence of plasmodesmata between SC and filial tissues indicates that they are apoplastically connected (Patrick and Offler, Reference Patrick and Offler2001; Zhang et al., Reference Zhang, Zhou, Dibley, Tyerman, Furbank and Patrick2007). Apart from this, the SC exerts its germination-restrictive action most of the time by its mechanical resistance to radicle protrusion (Rodríguez-Gacio et al., Reference Rodríguez-Gacio, Iglesias-Fernández, Carbonero and Matilla2012; Carrillo-Barral et al., Reference Carrillo-Barral, Matilla, Rodríguez-Gacio and Iglesias-Fernández2018; Steinbrecher and Leubner-Metzger, Reference Steinbrecher and Leubner-Metzger2018). In this context, A. thaliana SC mutants affected in testa shape and pigmentation, and which display reduced dormancy, confirms the importance of the seed envelope in the control of dormancy and germination, and highlight the importance of the testa as a restriction to radicle emergence (Debeaujon et al., Reference Debeaujon, Léon-Kloosterziel and Koornneef2000; Bentsink and Koornneef, Reference Bentsink and Koornneef2008; Rodríguez-Gacio et al., Reference Rodríguez-Gacio, Iglesias-Fernández, Carbonero and Matilla2012; Steinbrecher and Leubner-Metzger, Reference Steinbrecher and Leubner-Metzger2018). On the other hand, the requirement for gibberellins (GAs) in A. thaliana seed germination is determined by the SC characteristics, embryonic growth potential and embryonic abscisic acid (ABA) (Urbanova and Leubner-Metzger, Reference Urbanova, Leubner-Metzger, Hedden and Thomas2016; Nonogaki, Reference Nonogaki, Marion-Poll and Seo2019). Inhibition of ABA catabolism delays germination by inhibiting SC softening in Arabidopsis (Lee et al., Reference Lee, Piskurewicz, Tureckova, Strnad and López-Molina2010). Interestingly, this last feature has been confirmed in distantly related species. Together, the degradation of the SC surrounding the micropylar area makes the protrusion of the elongated embryonic axis more feasible. Recently, it has been shown in Arabidopsis that endosperm cell expansion is a key component of germination (Sánchez-Montesino et al., Reference Sánchez-Montesino, Bouza-Morcillo, Márquez, Ghita, Durán-Nebreda, Gómez, Holdsworth, Bassel and Oñate-Sánchez2019). All of these elongation and weakening processes require the hydrolysis of cell wall (CW) components for seed germination to occur (Holdsworth et al., Reference Holdsworth, Bentsink and Soppe2008; Linkies et al., Reference Linkies, Müller, Morris, Turecková, Wenk, Cadman, Corbineau, Strnad, Lynn, Finch-Savage and Leubner-Metzger2009; Lee et al., Reference Lee, Piskurewicz, Tureckova, Strnad and López-Molina2010; Rodríguez-Gacio et al., Reference Rodríguez-Gacio, Iglesias-Fernández, Carbonero and Matilla2012). In addition to GAs and ABA, auxin appears to be involved in CW softening. Auxin is well known to induce changes in CW mechanical properties and CW synthesis. Auxin-induced organ outgrowth requires demethylesterification of pectins, which causes CW loosening (Braybrook and Peaucelle, Reference Braybrook and Peaucelle2013). This pectin degradation process inhibits, inter alia, endosperm cellularization in Arabidopsis (Wolf et al., Reference Wolff, Jiang, Wang, Santos-González and Köhler2015; Batista et al., Reference Batista, Figueiredo, Santos-González and Köhler2019).
Unlike the gymnosperms, which only have one single integument, angiosperms have two (i.e. OI and II) which can be either two- or multi-cell layered (Endress, Reference Endress2011b; Coen et al., Reference Coen, Fiume, Xu, De Vos, Lu, Pechoux, Lepiniec and Magnani2017). As the seed matures, each layer acquires a specific fate. Thus, the outermost cell layer of the OI (oi2) produces mucilage, and usually forms a dead covering layer in mature seeds (Debeaujon et al., Reference Debeaujon, Lepiniec, Pourcel, Routaboul, Bradford and Nonogaki2007; Huang et al., Reference Huang, DeBowles, Esfandiari, Dean, Carpita and Haughn2011; Western, Reference Western2012; Tsai et al., Reference Tsai, Kunieda, Rogalski, Foster, Ellis, Ellis and Haughn2017). Mucilage accumulation leads to contraction of the cytoplasm that remains confined to the centre of the cell. After a while, the cytoplasm columns are replaced by CW components, thus forming the columella. Once all cell layer structures have crashed, the seed shape will hold (Haughn and Chaudhury, Reference Haughn and Chaudhury2005). When imbibition occurs, the mucilage is apoplastically released, promoting a moist environment during seed germination (Western, Reference Western2012). However, the inner cell layer (oi1) may remain viable (Figueiredo and Köhler, Reference Figueiredo and Köhler2014), whereas ii2 and ii1’ undergo programmed cell death (PCD) during seed development (Domínguez and Cejudo, Reference Domínguez and Cejudo2014). In Arabidopsis, the integument II has three layers and the innermost named endothelium (ii1) contacts directly with the endosperm cells and synthesizes, shortly after fertilization, flavonoid-derived tannins called proanthocyanidins (PAs) (Francoz et al., Reference Francoz, Lepiniec and North2018). Later in development PAs are released, oxidized and spread to the remaining SC layers conferring a brown colour to the seed (Lepiniec et al., Reference Lepiniec, Debeaujon, Routaboul, Baudry, Pourcel, Nesi and Caboche2006). Regarding the release of PAs, recent studies have demonstrated that these tannins are stored within the vacuole. Therefore, PAs and/or their precursors must be shuttled across the tonoplast. The elimination of the AHA10 gene, involved in encoding a putative P-type ATPase H+ pump in Arabidopsis SC, disrupts the production of PAs resulting in pale SC phenotypes with increased levels of PA precursors (Baxter et al., Reference Baxter, Young, Armstrong, Foster, Bogenschutz, Cordova, Peer, Haze, Murphy and Harper2005; González et al., Reference González, Brown, Hatlestad, Akhavan, Smith, Hembd, Moore, Montes, Mosley, Resendez, Nguyen, Wilson, Campbell, Sudarshan and Lloyd2016). It is noteworthy that PAs also sometimes act as germination inhibitors (Ma et al., Reference Ma, Guo, Han and Yang2015; Shah et al., Reference Shah, Ni, Chen, Wang, Liu, Chen, Tang, Fu and Wu2018). PAs inhibit seed germination by affecting ABA, GA and ROS regulatory genes (Jia et al., Reference Jia, Wu, Ye, Liu, Shi, Xu, Zhi, Rahman, Xia and Zhang2012). The absence of the endothelium results in seed abortion (Mizzotti et al., Reference Mizzotti, Mendes, Caporali, Schnittger, Kater, Battaglia and Colombo2012). Although no endothelium exists in the ancient A. trichopoda, the micropyle-adjacent cells are usually tanniniferous (Tobe et al., Reference Tobe, Jaffré and Raven2000). On the other hand, seed development relies on a coordinated interaction between the outer environment, the SC, the embryo and the endosperm (Radchuk and Borisjuk, Reference Radchuk and Borisjuk2014). Thus, the environmental cues and flow of nutrients from the mother plant to the endosperm and embryo must pass through the SC (Chen et al., Reference Chen, Lin, Qu, Sosso, McFarlane, Londono, Samuels and Frommer2015). All this trafficking is controlled at genetic, epigenetic, metabolic and hormonal levels in order to direct seed development (Haughn and Chaudhury, Reference Haughn and Chaudhury2005; Köhler and Makarevich, Reference Köhler and Makarevich2006; Köhler and Kradolfer, Reference Köhler and Kradolfer2011; Figueiredo and Köhler, Reference Figueiredo and Köhler2014). However, it is not yet known how this complex trafficking network is controlled. In addition, during seed development, maternal tissues enclosing the embryo (i.e. nucellus, SC in dicots, pericarp in monocots and nucellar projections) undergo a progressive degeneration or, where appropriate, disappearance by PCD (Domínguez and Cejudo, Reference Domínguez and Cejudo2014; Bastos-Lima et al., Reference Bastos-Lima, Trindade, da Cunha, Oliveira, Topping, Lindsey and Fernandes2015; Lu and Magnani, Reference Lu and Magnani2018; Matilla, Reference Matilla2019). Based on the above and current progress (e.g. Villanueva et al., Reference Villanueva, Broadhvest, Hauser, Meister, Schneitz and Gasser1999; Debeaujon et al., Reference Debeaujon, Léon-Kloosterziel and Koornneef2000; Yamada et al., Reference Yamada, Ito and Kato2003; Haughn and Chaudhury, Reference Haughn and Chaudhury2005; Reinheimer and Kellogg, Reference Reinheimer and Kellogg2009; Lee et al., Reference Lee, Piskurewicz, Tureckova, Strnad and López-Molina2010; Roszak and Köhler, Reference Roszak and Köhler2011; Liu et al., Reference Liu, Zhang, Zhao, Feng, Li, Yang, Luan, Li and He2013; Locascio et al., Reference Locascio, Roig-Villanova, Bernardi and Varotto2014), this update emphasizes the evolution of the SC, its biochemical characteristics and genetic control of its development by auxin, adding to the understanding of the roles of the SC in higher plants.
Evolutionary alterations of the seed coat: unitegmy and bitegmy
Cells from both OI and II undergo prompt growth and differentiation once the ovule has been fertilized (Haughn and Chaudhury, Reference Haughn and Chaudhury2005). These processes are coordinated with the endosperm (Coen et al., Reference Coen, Fiume, Xu, De Vos, Lu, Pechoux, Lepiniec and Magnani2017). The OI grows faster than the II and extends beyond the apex of the nucellus first (Souza-Caetano et al., Reference Souza-Caetano, Basso-Alves, Cortez, Garcia De Brito, Michelangeli, Reginato, Goldenberg, Carmello-Guerreiro and Pádua Teixeira2018). The II grows as a radially symmetrical structure to envelop the terminal nucellus whereas OI grows asymmetrically. OI shows a curvature that is distinct from II, due to the faster cell division rate in the abaxial than adaxial side (Fig. 1). After a time of fertilized ovule development, II and OI form the SC (Skinner et al., Reference Skinner, Hill and Gasser2004; Colombo et al., Reference Colombo, R. Battaglia and Kater2008). The SC of different species undergoes similar phases of development in relation to the endosperm and embryo. Nevertheless, the SC and endosperm develop first in legumes (Weber et al., Reference Weber, Borisjuk and Wobus2005). However, SC characteristics, such as composition, structure and thickness have evolved to adapt to the environment of the mother plant. Regarding SC thickness, this property is considered as a stable character and affects seed germination, dormancy and mortality (Linkies et al., Reference Linkies, Graeber, Knight and Leubner-Metzger2010; Endress, Reference Endress2011a). Data obtained from a large number of species belonging to diverse families indicate that the SC is different in architecture and thickness (Beeckman et al., Reference Beeckman, De Rycke, Viane and Inze2000; Coen and Magnani, Reference Coen and Magnani2018). Thus, the ovule can be covered in three ways. The first is the ancestral and more common bitegmic ovule covered by II and OI, respectively. Among other species, this is the case in Arabidopsis thaliana (Coen et al., Reference Coen, Fiume, Xu, De Vos, Lu, Pechoux, Lepiniec and Magnani2017), Prunus persica and P. armeniaca (Lora et al., Reference Lora, Hormaza and Herrero2015) and Oryza sativa (Endress, Reference Endress2011b). It is striking that the ancient A. trichopoda has also bitegmic ovules (Tobe et al., Reference Tobe, Jaffré and Raven2000). However, the origin and homology of the OI is not clear (Doyle, Reference Doyle2006; Doyle et al., Reference Doyle, Manchester and Sauquet2008) and therefore the existence of OI in bitegmic angiosperms remains elusive. However, the II of angiosperms is probably homologous to the only integument in gymnosperms and their ancestors (Reinheimer and Kellogg, Reference Reinheimer and Kellogg2009). The second way is the unitegmic ovule as the earliest Paleozoic seeds (Gerrienne et al., Reference Gerrienne, Meyer-Berthaud, Fairon-Demaret, Streel and Steemans2004), Solanaceae (Skinner et al., Reference Skinner, Brown, Kuzoff and Gasser2016), Prunus incisa (Lora et al., Reference Lora, Hormaza and Herrero2015), Arabidopsis thaliana mutant aberrant testa shape (ats) (McAbee et al., Reference McAbee, Hill, Skinner, Izhaki, Hauser, Meister, Venugopala Reddy, Meyerowitz, Bowman and Gasser2006), Asterids (e.g. Petunia and Solanum lycopersicum) and Rosaceae (Endress, Reference Endress2011b) and more common gymnosperms. The ovule is surrounded by only one integument in all these and other species (Gasser and Skinner, Reference Gasser and Skinner2019). The unitegmy existing in extant gymnosperms is probably homologous to that of ancestral seed plants. Strikingly, although phylogenetic analyses suggest that bitegmy is the ancestral condition in angiosperms, unitegmic ovules have arisen independently several times during angiosperm evolution (Endress, Reference Endress2011b; Gasser and Skinner, Reference Gasser and Skinner2019). However, the reason(s) for the presence of a single integument in some angiosperms is unknown. Although there are many studies on unitegmy, it is not clear if its appearance in angiosperms was the result of the loss of one of the two integuments or, alternatively, was the fusion of OI and II due to the presence of intercalary growth between II and OI (Colombo et al., Reference Colombo, R. Battaglia and Kater2008; Lora et al., Reference Lora, Hormaza and Herrero2015). McAbee et al. (Reference McAbee, Kuzoff, Charles and Gasser2005) were able to show in Impatiens that the bitegmic to unitegmic transition was the result of congenital fusion of OI and II. The pattern of expression of INO orthologs was conserved in bitegmic and unitegmic species. Thus, INO shows almost identical expression in the outermost layer of Arabidopsis and tomato, despite poor similarity between promoter sequences (Skinner et al., Reference Skinner, Brown, Kuzoff and Gasser2016). Genetic analyses in Arabidopsis suggest that integumentary fusion can be achieved through a lack of boundary formation between OI and II (Colombo et al., Reference Colombo, R. Battaglia and Kater2008; Kelley et al., Reference Kelley, Arreola, Gallagher and Gasser2012). This interpretation is confirmed by the analysis of molecular markers of boundary formation in Prunus bitegmic and unitegmic ovules (Lora et al., Reference Lora, Hormaza and Herrero2015; Table 1). Moreover, the expression analyses in Santalales ategmic ovules, revealed that integument molecular markers are expressed at the distal end of the ovules (Brown et al., Reference Brown, Nickrent and Gasser2010). These data have been interpreted as the fusion of an integument remnant to the nucellus. Regarding the fusion process, based on previous data and their own, Coen and Magnani (Reference Coen and Magnani2018) point out two different and non-exclusive mechanisms: (a) total or partial lack of boundary formation between II and OI; and (b) intercalary growth between them. Finally, the third way for ovule coating is the ategmy found in some species of the order Santalales in which the integuments may be missing or poorly developed (Brown et al., Reference Brown, Nickrent and Gasser2010). Remarkably, some taxa have a third envelope that originates from the funiculus, which becomes a fleshy structure and covers the SC (Endress, Reference Endress2011a,Reference Endressb). This additional envelope is called the aril and occurs, inter alia, in Passiflora L., Nymphaea L. and gymnosperm Taxus baccata (Coen and Magnani, Reference Coen and Magnani2018). In this case the two integuments inside of the aril become dry and hard. Secondly, the rice seed modifies the number of integuments after fertilization; the reason(s) for this second process is still unknown (Krishnan and Dayanandan, Reference Krishnan and Dayanandan2003).

Fig. 1. The spatial distribution of genes known in Arabidopsis to regulate seed integument formation and ovule development. More details are listed in Table 1. ATS, aberrant testa shape; CNA, corona; ETT, ettin; INO, inner no outer; KLU, P450 kluh; PHB, phabulosa; PHV, phavoluta; REV, revolute; SKT, seedstick; SUP, superman.
Table 1. Some genes and proteins involved in regulation of seed integuments and ovule development

Given that the general morphology of the ovule is fairly well conserved in angiosperms and that these plants have two integuments (i.e. II and OI) and gymnosperms only one (i.e. II) (Gasser and Skinner, Reference Gasser and Skinner2019), it is of interest to know what gymnosperm gene information disappeared and which remained and is exclusive for flowering plants (see next section and Table 1). Due to the absence of contrasting data in gymnosperms, it is necessary to compare ancient angiosperms, such as Amborella with recent angiosperms. The Amborellaceae family is the most ancient of angiosperms and consists of a single genus and a single species, Amborella, which has two-cell-layered OI and II. There are some reports that the II of angiosperms is considered a homologue to the single integument of gymnosperms (Reinheimer and Kellogg, Reference Reinheimer and Kellogg2009). In relation to this, some peculiarities about the evolution of SC thickness have been reviewed recently (Coen and Magnani, Reference Coen and Magnani2018). All these and other data may attempt to reveal why gymnosperms possess only II and why in many angiosperms II is covered by an OI or even also by a third layer called the aril. However, unless it is essential, evolution does not seem willing to increase the number of organs. An interesting reason to justify the presence of OI could well be to reinforce the protection of both ovule and seed. However, why then did II not become thicker and more resistant? An explanation could be that SC thickness is genetically considered a stable character (Endress, Reference Endress2011a). Thus, it can be concluded that the origin and the homology of the OI is at present uncertain. Current hypotheses support the origin of OI from a leaf-like structure called the cupule, which was present in groups such as the glossopterids and Caytonia, possible sisters of angiosperms (Doyle, Reference Doyle2006). If that was the case, there must be a genetic homology between these leaf-like structures and the current OI. That is, homologous genes should be expressed in homologous structures (i.e. cupule and OI). Data of YABBI and KANADI (see Table 1) seem to be congruent with this evolutionary hypothesis (Gasser and Skinner, Reference Gasser and Skinner2019; Jaramillo and Kramer, Reference Jaramillo and Kramer2007). Separate origins for both OI and II are also supported at the molecular level as the control of both II and OI development occurs through different genes (Arnault et al., Reference Arnault, Vialette, Andres-Robin, Fogliani, Gâteblé and Scutt2018). In conclusion, given the small number of species studied until now, to explain why the appearance of OI is necessary, it is imperative to expand the range of angiosperm species to those evolutionarily close to gymnosperms and study gene expression and genetics in both wild-type and deficient mutants in key genes clearly involved in the appearance and development of OI.
Gene expression during SC development
It is well known in Arabidopsis that the development of abaxial and adaxial parts (Fig. 1) of both OI and II depends on distinct sets of genes (Arnault et al., Reference Arnault, Vialette, Andres-Robin, Fogliani, Gâteblé and Scutt2018). A series of them involved in the development of the ovule and, very specifically, in the growth and development of II and OI are shown in detail (Table 1). Among the listed genes, INO, ANT, WUS, ETT and KAN deserve attention for their roles in the initiation and differentiation of integuments and subsequent SC appearance. However, it is not known which of these genes were already present in early plants and which evolved later. This fact is evolutionarily significant and requires further investigation.
INNER NO OUTER (INO) is a TF of the YABBY gene family sufficient in Arabidopsis for OI initiation at the CZSC level (i.e. gynobasal side of the ovule; Fig. 1) and OI asymmetric growth. That is to say, INO is a positive regulator of OI growth and ANT works as a negative regulator that helps to establish the spatial pattern of INO expression (Villanueva et al., Reference Villanueva, Broadhvest, Hauser, Meister, Schneitz and Gasser1999). Otherwise, ANT may promote OI development by facilitating II development and through the positive regulation of INO (Skinner et al., Reference Skinner, Brown, Kuzoff and Gasser2016). Conservation of the specific expression pattern and function of INO orthologs in OI has been observed in all other angiosperm species in which INO has been examined (Yamada et al., Reference Yamada, Ito and Kato2003, Reference Yamada, Yokota, Hirayama, Imaichi, Kato and Gasser2011; McAbee et al., Reference McAbee, Kuzoff, Charles and Gasser2005; Lora et al., Reference Lora, Hormaza, Herrero and Gasser2011; Skinner et al., Reference Skinner, Brown, Kuzoff and Gasser2016). In other words, INO was conserved in bitegmic and unitegmic species, indicating a likely conservation of INO gene function between Rosids and Asterids. Because INO is expressed only in the OI, it might serve as a marker for OI identity in the unitegmic process. Unfortunately, very few species of basal and higher angiosperms have been studied to date in order to explain the exact role of INO through evolution. However, expression studies in the basally diverging angiosperm Nymphaea suggest that INO’s tissue-specific role has been conserved from early stages of angiosperm evolution (Yamada et al., Reference Yamada, Ito and Kato2003, Reference Yamada, Yokota, Hirayama, Imaichi, Kato and Gasser2011). Likewise, genetic analyses show that LUG, SEU and STK contribute to the growth of the OI (Bao et al., Reference Bao, Azhakanandam and Franks2010; Mizzotti et al., Reference Mizzotti, Ezquer, Paolo, Rueda-Romero, Guerra, Battaglia, Rogachev, Aharoni, Kater, Caporali and Colombo2014). Recently, it was confirmed that INO interacts with both LUG and SEU co-repressors and with the co-activator PRZ1 (Simon et al., Reference Simon, Skinner, Gallagher and Gasser2017). Integrating the above data, INO has a bifunctional nature as it acts as both a repressor and activator of target genes. For more information about INO molecular properties, see Table 1. The similar expression patterns of the putative ortholog of A. thaliana ovule development gene INO was found inside intermediate integuments of Impatiens (i.e. an Ericales genus), supporting the hypothesis of integument fusion in this species with bitegmic (e.g. I. hookeriana), unitegmic (e.g. I. walleriana) and intermediate (e.g. I. balsamina) integument morphology (McAbee et al., Reference McAbee, Kuzoff, Charles and Gasser2005). On the other hand, the authors indicate that the role of INO has been conserved between two divergent angiosperms, the rosid Arabidopsis and the asterid Impatiens (McAbee et al., Reference McAbee, Kuzoff, Charles and Gasser2005). Likewise, an ortholog of Arabidopsis INO is similarly expressed in genus Nymphaea (NaINO), a diverging angiosperm (Yamada et al., Reference Yamada, Ito and Kato2003).
On the other hand, the WUSCHEL (WUS) TF is necessary to prevent INO expression in developing II (Sieber et al., Reference Sieber, Gheyselinck, Gross-Hardt, Laux, Grossniklaus and Schneitz2004). Thus, ectopic WUS expression in the CZSC resulted in the formation of a single II and multiple OI (Gross-Hardt et al., Reference Gross-Hardt, Lenhard and Laux2002; Sieber et al., Reference Sieber, Gheyselinck, Gross-Hardt, Laux, Grossniklaus and Schneitz2004). WUS is strictly expressed in the nucellar region with the highest level of expression coincident with integument initiation (Gross-Hardt et al., Reference Gross-Hardt, Lenhard and Laux2002). It is likely that both ANT and WUS expression need to occur at a distance on the surface of the ovule to induce integument initiation (Skinner et al., Reference Skinner, Brown, Kuzoff and Gasser2016), but it is a possibility that still requires thorough confirmation. However, what is clear is that ANT and WUS are essential for the formation of both OI and II. In the case of OI development, ANT and WUS interact with INO which positively affects ANT expression (Skinner et al., Reference Skinner, Brown, Kuzoff and Gasser2016). Given that the over-expression of WUS results in supernumerary integuments (Sieber et al., Reference Sieber, Gheyselinck, Gross-Hardt, Laux, Grossniklaus and Schneitz2004), the dynamics of the expression of this TF could explain both the origin of II and the variable number of integuments observed in seeds. This variation fluctuates from bitegmy to the supernumerary integuments of taxa nested within otherwise unitegmic clades (Yang, Reference Yang2004; Friis et al., Reference Friis, Crane and Pedersen2011), to third integuments in ancestrally bitegmic clades (Endress, Reference Endress2011b).
ARF3/ETTIN (ETT) is a master regulator of development and morphogenesis of the female reproductive structure (Simonini et al., Reference Simonini, Deb, Moubayidin, Stephenson, Valluru, Freire-Rios, Sorefan, Weijers, Friml and Østergaard2016). KANNADI (KAN4/ATS) and ETT are expressed in the abaxial side of the II (Fig. 1) and act to remove auxin from the zone between the two integument primordia (Kelley et al., Reference Kelley, Arreola, Gallagher and Gasser2012). In certain species of unitegmic Prunus, expression of the ETT ortholog was absent from the CZSC and II (Lora et al., Reference Lora, Hormaza and Herrero2015). It was proposed that the loss of this expression represents a possible evolutionary mechanism for the reduction from two integuments to one in these species (Lora et al., Reference Lora, Hormaza and Herrero2015). In Arabidopsis, there is a clear division of functions between the II and OI, as ATS is the principal KAN family member expressed in II, while the role of ATS is played redundantly by KAN1 and KAN2 in OI (McAbee et al., Reference McAbee, Hill, Skinner, Izhaki, Hauser, Meister, Venugopala Reddy, Meyerowitz, Bowman and Gasser2006). KAN is also present in Selaginella and Physcomitrella, suggesting an ancestral function of this gene family (McAbee et al., Reference McAbee, Hill, Skinner, Izhaki, Hauser, Meister, Venugopala Reddy, Meyerowitz, Bowman and Gasser2006).
Taken together, it can be concluded that actual differences are ancient, and already existed in early flowering plants (Arnault et al., Reference Arnault, Vialette, Andres-Robin, Fogliani, Gâteblé and Scutt2018). The evolution to unitegmy from bitegmy in angiosperms is not uniform. In unitegmic basal angiosperms and some derived groups it probably evolved mainly by the loss of the OI. However, in derived eudicots (e.g. Asterids) it is mainly by incorporation of the OI into II (Endress, Reference Endress2011a). Taking the ATS task as an example (Table 1), it was demonstrated that loss of this function in the ats-1 mutant leads to the fusion of II and OI that grow as a unit to produce a single fused structure (McAbee et al., Reference McAbee, Hill, Skinner, Izhaki, Hauser, Meister, Venugopala Reddy, Meyerowitz, Bowman and Gasser2006; Dorcey et al., Reference Dorcey, Urbez, Blázquez, Carbonell and Perez-Amador2009; Arnault et al., Reference Arnault, Vialette, Andres-Robin, Fogliani, Gâteblé and Scutt2018). Interesting studies in unitegmic Prunus have demonstrated that expression of an ETT ortholog was absent from the chalaza and II. This loss of expression may be of evolutionary interest (i.e. reduction from two integuments to one in this Rosaceae). However, ETT is expressed in II in bitegmic species (Lora et al., Reference Lora, Hormaza and Herrero2015).
Auxin, a new player involved in SC formation and development
Given the great difficulty in isolating plant zygotes, the molecular and hormonal processes that take place as a result of fertilization are scarcely known. Nevertheless, the use of sophisticated methodologies is helping now to clarify it (Brunoud et al., Reference Brunoud, Wells, Oliva, Larrieu, Mirabet, Burrow, Beeckman, Kepinski, Traas, Bennett and Vernoux2012; Liao et al., Reference Liao, Smet, Brunoud, Yoshida, Vernoux and Weijers2015; Schon and Nodine, Reference Schon and Nodine2017; Robert et al., Reference Robert, Park, Gutièrrez, Wójcikowska, Pěnčík, Ondřej Novák, Junyi Chen, Grunewald, Dresselhaus, Friml and Laux2018). It is very likely that the onset of transcription and the activation of translation occur in the endosperm and the zygote (Aw et al., Reference Aw, Hamamura, Chen, Schnittger and Berger2010). One of the events that sustains this assertion derives from the fact that the fertilized endosperm development is required to induce the autonomous initiation of SC (García et al., Reference García, Saingery, Chambrier, Mayer, Jürgens and Berger2003; Aw et al., Reference Aw, Hamamura, Chen, Schnittger and Berger2010; Hands et al., Reference Hands, Rabiger and Koltunov2016; Figueiredo and Köhler, 2011, Reference Figueiredo and Köhler2018). Therefore, removal of the endosperm inhibits SC development (Weijers et al., Reference Weijers, van Hamburg, van Rijn, Hooykaas and Offringa2003) as developing endosperm produces molecular signals which are addressed to the integuments to initiate their formation (Ingram, Reference Ingram2010). Thus, endosperm and SC coordinate their growth through a cross-talk signalling pathway that was first identified in the study of the maternally acting TTG2 (García et al., Reference García, Fitz Gerald and Berger2005; Ingram, Reference Ingram2010). The ttg2 mutant shows premature arrest of endosperm development and reduced seed size (García et al., Reference García, Fitz Gerald and Berger2005; Ingram, Reference Ingram2010). Besides, mutations in genes belonging to the HAIKU family (iku1 and iku2) produce seeds with reduced endosperm growth and early cellularization, which results in decreased seed size (Garcia et al., Reference García, Saingery, Chambrier, Mayer, Jürgens and Berger2003). On the other hand, studies of endosperm development indicate that the presumed TF AGL62 (i.e. an AGAMOUS LIKE MADS-box domain protein) plays a determinant role in the development of central cell and/or endosperm (Figueiredo and Köhler, Reference Figueiredo and Köhler2018). The expression pattern of AGL62 and the phenotype of agl62-2 (endosperm cellularizes prematurely and shows retention of auxin) suggest that AGL62 functions during the syncytial phase by suppressing the expression of genes required for endosperm cellularization (Kang et al., Reference Kang, Steffen, Portereiko, Lloyd and Drews2008). Another indication that the endosperm regulates SC growth comes from the characterization of the agl62 mutant (Kang et al., Reference Kang, Steffen, Portereiko, Lloyd and Drews2008; Roszak and Köhler, Reference Roszak and Köhler2011). Seeds of the agl62 mutant show early endosperm cellularization and fail to develop a SC, despite the presence of a dividing endosperm. This suggests that AGL62 is crucial for the formation of the signal that initiates seed coat development. On the other hand, several studies have also shown that not only does the endosperm control SC growth, but that the reverse also happens. So, TRANSPARENT TESTA GLABRA2 (TTG2) is strongly expressed in all SC layers and is known to be a part of the PA- and mucilage synthesis pathways (Johnson et al., Reference Johnson, Kolewski and Smyth2002). The ttg2 mutation causes defects in endosperm growth and cellularization and decreased SC cell elongation (Garcia et al., Reference García, Fitz Gerald and Berger2005). Furthermore, the AGL62 expression pattern in fis mutant seed (i.e. endosperm cell is diploid) suggests that endosperm cellularization is triggered by suppression of AGL62 at the end of the syncytial phase and that this suppression is mediated by the Fertilization Independent Seed (FIS) Polycomb Repressive Complex 2 (i.e. FIS-PRC2; Kang et al., Reference Kang, Steffen, Portereiko, Lloyd and Drews2008; Roszak and Köhler, Reference Roszak and Köhler2011). The evolutionarily conserved Polycomb-Group (PcG) proteins are chromatin-associated factors contributing to the maintenance of transcriptional repression (Grossniklaus and Paro, Reference Grossniklaus and Paro2014; Laugesen et al., Reference Laugesen, Westergaard Højfeldt and Helin2019). The PcG are organized in multimeric complexes of which Polycomb Repressor Complex 1 and 2 (PRC1 and PRC2) are the best characterized. These proteins control a multitude of developmental transitions in plants (Derkacheva and Henning, Reference Derkacheva and Henning2014; Laugesen et al., Reference Laugesen, Westergaard Højfeldt and Helin2019). The fertilization-independent development of the ovule is repressed by FERTILIZATION INDEPENDENT SEED (FIS), a PRC2 complex. In particular, the FERTILIZATION INDEPENDENT ENDOSPERM (FIE) and MULTICOPY SUPPRESSOR OF IRA1 (MSI1) FIS, both expressed in the nucellus and SC, act sporophytically to repress the differentiation of the integuments (Roszak and Köhler, Reference Roszak and Köhler2011; Xu et al., Reference Xu, Fiume, Coen, Pechoux, Lepiniec and Magnani2016). FIS represses seed development in the absence of fertilization and is essential for seed growth. After fertilization of the central cell, the endosperm initiates a signal through the action of AGL62, relieving the FIS mediated repression and leading to the differentiation of the ovule integuments into the SC (Roszak and Köhler, Reference Roszak and Köhler2011).
Figueiredo et al. (Reference Figueiredo, Batista, Roszak, Henning and Köhler2016) suggest that auxin is the putative fertilization signal that coordinates endosperm and SC development. The important role of AGL62 is demonstrated by the fact that once the PRC2 repression is relieved, AGL62 leads to differentiation of the ovule integuments into SC (Roszak and Köhler, Reference Roszak and Köhler2011). Thus, AGL62 expression controls the timing of cellularization (Hehenberger et al., Reference Hehenberger, Kradolfer and Köhler2012) and seeds lacking this putative TF fail to initiate formation of the integuments (Roszak and Köhler, Reference Roszak and Köhler2011; Coen et al., Reference Coen, Fiume, Xu, De Vos, Lu, Pechoux, Lepiniec and Magnani2017). In short, the development of endosperm and SC is tightly linked to PRC2 function. In contrast, the initiation of embryo development is probably controlled by other repressive pathways unrelated to PRC2 (Roszak and Köhler, Reference Roszak and Köhler2011). Although some components of the PRC2 complex show evolutionary conservation, the identity of the imprinted genes encoding PRC2 components is not conserved (e.g. in cereal endosperm; Tonosaki and Kinoshita, Reference Tonosaki and Kinoshita2015). Genomic imprinting is an epigenetic phenomenon rendering maternal and paternal alleles specifically expressed dependent on their parent of origin (Gehring, Reference Gehring2013). PRC2 blocks the SC development and this blocking seems necessary to be suppressed upon ovule fertilization. Thus, PRC2 must be strongly disabled following fertilization (i.e. phase of syncytial endosperm), whereas during cellularization of the endosperm negatively affects AGL62 (Figueiredo et al., Reference Figueiredo, Batista, Santos-González and Köhler2019). Recently, a subset of AGL62 genes was subjected to FIS-PRC2-dependent maternal imprinting or FIS-PRC2-independent paternal imprinting, indicating a dual role for FIS-PRC2 in the regulation of its target genes during early endosperm development (Zhang et al., Reference Zhang, Wang, Zhang, Skaggs, Lloyd, Ran, An, Schumaker, Drews and Yadegari2018). Recently, Creff et al. (Reference Creff, Brocard and Ingram2015) have shown that during the onset of fertilization, endosperm-derived pressure is perceived in the innermost cell layer of the OI which subsequently undergoes thickening of its inner CW (for more details, see Fig. 1).
Until now, the role of phytohormones in zygotic embryogenesis mainly refers to the study of the eudicot Arabidopsis thaliana. Auxin involvement in the formation of the seed is now undisputed (Smit and Weijers, Reference Smit and Weijers2015) but very little is known about auxin biosynthesis and homeostasis, polar auxin transport and response during early embryogenesis in monocots. However, some of these features seem to be conserved in both monocot and dicot seeds (Robert et al., Reference Robert, Park, Gutièrrez, Wójcikowska, Pěnčík, Ondřej Novák, Junyi Chen, Grunewald, Dresselhaus, Friml and Laux2018; Robert, Reference Robert2019). At the beginning of the investigation of the possible intervention of auxins in SC formation, it has been shown that the female gametophyte cellularization is directly dependent on the establishment of an auxin gradient inside the gametophyte (Figueiredo et al., Reference Figueiredo, Batista, Santos-González and Köhler2019). This gradient defines the fates of the female gametophyte cells. Thus, the highest auxin concentration originates synergids, followed by egg cell and finally, the lowest concentrations originate the central cell and antipodals (Pagnussat et al., Reference Pagnussat, Alandete-Saez, Bowman and Sundaresan2009). In addition, female gametophyte development requires both localized auxin biosynthesis and auxin import from the sporophytic ovule (Panoli et al., Reference Panoli, Martin, Alandete-Saez, Simon, Neff, Swarup, Bellido, Yuan, Pagnussat and Sundaresan2015). Although auxin is sufficient to initiate autonomous endosperm development in A. thaliana, by inducing the first few divisions of the central cell (maximal auxin response in the seed of monocot maize; Chen et al., Reference Chen, Lausser and Dresselhaus2014), auxin alone is not sufficient to form a fully differentiated and cellularized endosperm (Figueiredo et al., Reference Figueiredo, Batista, Roszak and Köhler2015). Recently, ample molecular evidence has emerged, suggesting that once fertilization has become effective and consolidated, auxin biosynthesis in the endosperm drives the development of its own endosperm and is responsible for the start of the appearance of the SC (Dorcey et al., Reference Dorcey, Urbez, Blázquez, Carbonell and Perez-Amador2009; Figueiredo et al., Reference Figueiredo, Batista, Roszak and Köhler2015; Smit and Weijers, Reference Smit and Weijers2015). It was also demonstrated that auxin synthesized in the developing endosperm is transported into the integuments to support SC growth (Figueiredo et al., Reference Figueiredo, Batista, Roszak, Henning and Köhler2016) (Fig. 2). This hypothesis was evidenced by the authors since (i) auxin rapidly accumulates in the integuments after fertilization; (ii) impaired endosperm auxin signalling, but not auxin synthesis, did not impair SC formation; and (iii) ectopic auxin production in the central cell initiates SC development without fertilization. Auxin exerts its early physiological task directly in the zone where the integuments are beginning their formation (Figueiredo et al., Reference Figueiredo, Batista, Roszak, Henning and Köhler2016; Figueiredo and Köhler, Reference Figueiredo and Köhler2018). However, the mechanism of this transport is still not clear.
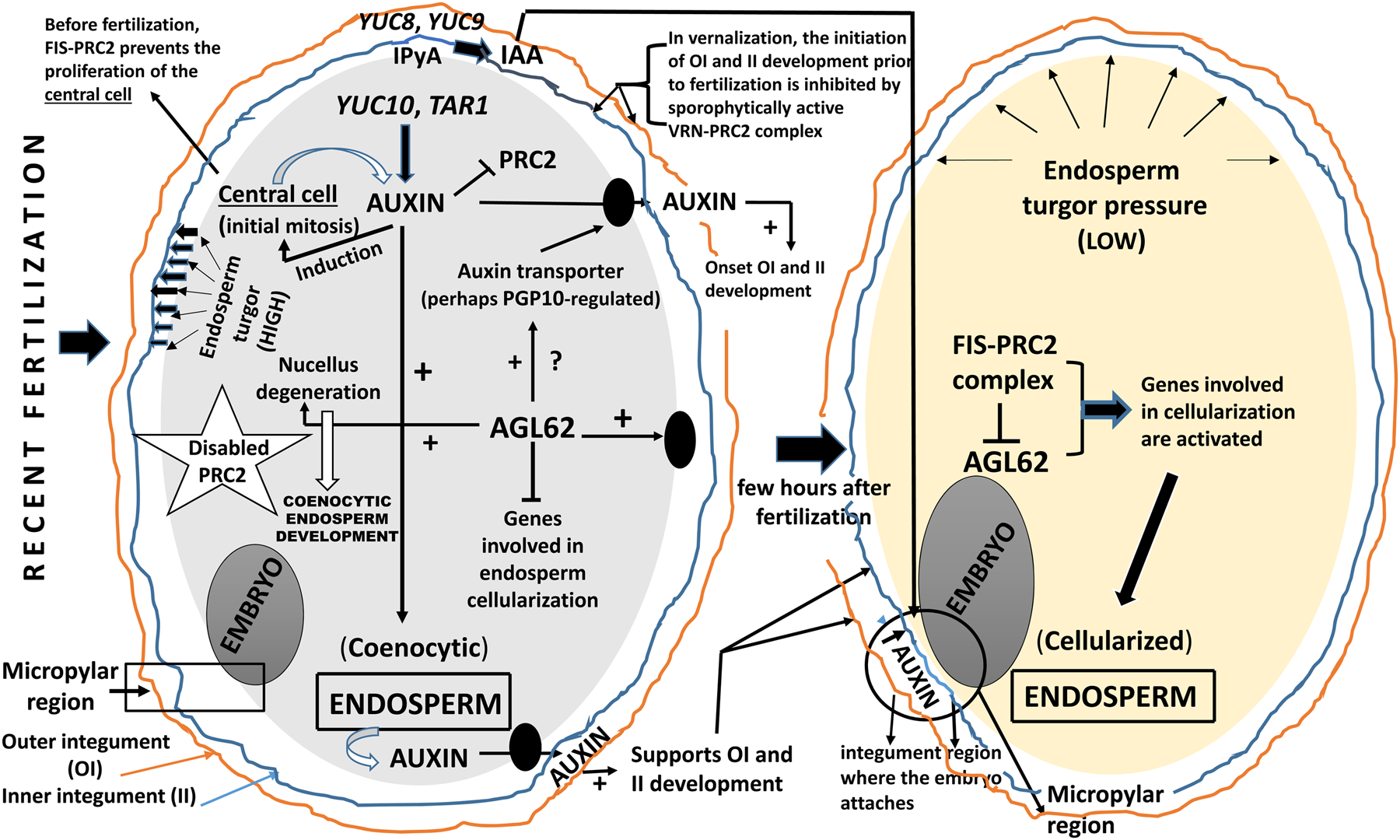
Fig. 2. Current status of the knowledge of auxin intervention in ovule fertilization and post-fertilization. It is important to note the mediation of AGL62 and PRC2 in the development of seed integuments and endosperm. AGL62, Agamous-like 62; IPyA, indolepyruvic acid; PRC2, polycomb-repressive complex 2; TAR, tryptophan aminotransferase-related; VRN, vernalization; YUC, YUCCA flavin monooxygenase.
By contrast, the possibility of an auxin being transported from SC to endosperm was recently also demonstrated (Chen et al., Reference Chen, Lausser and Dresselhaus2014). Interestingly, fertilization causes restriction of auxin export through the funiculus, resulting in the spread of auxin throughout the integuments (Larsson et al., Reference Larsson, Vivian-Smith, Offringa and Sundberg2017). It is appropriate to highlight that endosperm auxin synthesis is a feature which is evolutionarily conserved (Hatorangan et al., Reference Hatorangan, Laenen, Steige, Slotte and Kohler2016; Sun et al., Reference Sun, Wang, Wang, Jiang, Mao, Zhu, Wen, Wang, Lu, Yue, Xu and Ye2017). At present, the possibility of bidirectional transport of auxin is not ruled out. On the other hand, it is feasible that transport of auxin to integuments is necessary for the abolition of the PRC2 task on the integuments, then allowing the intervention by GAs and production of PAs in the endothelium (Lepiniec et al., Reference Lepiniec, Debeaujon, Routaboul, Baudry, Pourcel, Nesi and Caboche2006; Dorcey et al., Reference Dorcey, Urbez, Blázquez, Carbonell and Perez-Amador2009; Doughty et al., Reference Doughty, Aljabri and Scott2014; Figueiredo and Köhler, Reference Figueiredo and Köhler2018). However, it is still unknown how auxin intervenes in the regulation of the FIS-PcG complex (i.e. FIS-PRC2) during fertilization. Existing data suggest that the repression of the autonomous endosperm formation by PRC2-mediated control of auxin biosynthesis seems to be a conserved feature in angiosperms (Luo et al., Reference Luo, Platten, Chaudhury, Peacock and Dennis2009; Tonosaki and Kinoshita, Reference Tonosaki and Kinoshita2015; Figueiredo and Köhler, Reference Figueiredo and Köhler2018). Finally, it cannot be not ruled out that some mobile signal external to the endosperm contributes to the development of both endosperm and integuments. It is speculated that this signal could be the auxin from the pollen grain (Fig. 2). Finally, in a recent study related to auxin dynamics during the onset of embryo growth in Arabidopsis, Robert (Reference Robert2019) provided some outstanding evidence: (i) the expression of the auxin biosynthetic machinery and the auxin signal output are differentially regulated during ovule maturation and pollination; (ii) an increased activity of the auxin production via the indole-3-pyruvic acid (IPyA) pathway is a major contributor of auxin accumulation in the integuments after pollination; (iii) maternal auxin production in the integuments surrounding the embryo contributes to early embryo development; (iv) presumably, the integuments provide auxin directly to the embryo, while the endosperm-derived auxin does not have a notable role; and (v) in the maternal tissues of Arabidopsis (dicot) and maize (monocot) there is a similar increase in auxin response despite the remarkable difference in the organization of their ovules. Summarizing this section, different localization of auxin accumulation, signalling and activity coordinates embryo, endosperm and SC development. However, there are many gaps in clarifying the regulation of auxin synthesis and distribution during the life of the unfertilized ovule and subsequent embryogenesis, specifically the fertilized ovule with both coenocytic and cellularized endosperm. In other words, all recently obtained results suggest that the distribution of auxin to different intracellular or extracellular compartments is strongly controlled. This control by still unknown genes, is necessary to carry out the signalling depicted in Fig. 2.
Concluding remarks and future perspectives
The unravelling of SC regulation is an important objective of modern plant molecular biology. A promising challenge for the future is to translate basic research into practical applications in crops. In particular, the application to a selection of species of agricultural interest and the study based on the molecular regulation of the source–sink relationship that constitutes the SC and endosperm, are two main reasons to explore the SC at several -omics levels. Deepening the study of the expression of integument master regulator genes in higher plants would help better address the nature and evolution of SC components. Recent progress in the genetic and molecular mechanisms of SC development has been summarized in this update. Several genetic frameworks are emerging. Although the role of auxin in seed development is not fully understood, several studies have supported the idea that this phytohormone is essential. However, how auxin specifically influences development of the remaining seed components – endosperm and seed coat – is poorly understood. Analysis of auxin reporter activity suggests that after fertilization there is an increase of auxin content in the seed. Also, it is known that in several species, including Arabidopsis thaliana, application of exogenous auxin leads to parthenocarpic fruit formation. Given the importance of ABA in the seed maturation process, and in the induction and loss of dormancy, a study of the ABA–auxin inter-relationship at the various levels is timely.
Acknowledgments
The author thanks Henk Hilhorst and Robert Benech-Arnold for editing and critical reading of the manuscript.